Oncologic Imaging/Oncologic Anatomy
This chapter addresses two topics central to the management of patients with cancer: oncologic anatomy and oncologic imaging. Although these topics are relevant for all specialties involved in the treatment of cancer, they are particularly germane for radiation oncologists. A sound understanding of anatomy, especially pertaining to malignant processes, facilitates interpretation of imaging studies. Likewise, understanding the advantages and limitations of individual imaging modalities assists in defining rational clinical target volumes (CTVs) that maximize the therapeutic ratio.
Advances in diagnostic imaging have increased our ability to visualize macroscopic disease, referred to as gross tumor volume (GTV). Imaging is currently unable to identify microscopic tumor extension around a primary tumor or occult nodal involvement. A CTV is created to account for both of these uncertainties (Table 30.1). A rational definition of the CTV should reflect the clinician’s knowledge regarding the patterns of spread for each particular cancer. This involves both local spread around the primary site and patterns of lymphatic drainage. Appropriate expansion of a GTV to a CTV minimizes the risk of local failure (i.e., marginal miss), while reducing the risk of complications by avoiding regions at low risk of involvement (Fig. 30.1).
Radiation treatment planning has undergone considerable evolution during the past 20 years. With conventional planning, the physician conceives of beam orientations and aperture shapes based on the interpretation of available clinical and diagnostic information, including three-dimensional (3D) imaging data such as computed tomography (CT) or magnetic resonance imaging (MRI). The beam is then applied to the patient using a fluoroscopy-based conventional simulator, relying on an understanding of tumor and normal tissue anatomy and its association with fluoroscopic bony anatomy and surface anatomy. Relatively generous margins are used to account for inherent uncertainties of the process.
With 3D treatment planning, anatomic information from a planning CT scan is transferred to a computer where the images are segmented to define the tumor and normal tissues. Software allows this 3D information to be displayed and viewed from any orientation. Beam orientation and shape are chosen to encompass the target, yet minimize, as much as possible, normal tissue exposure. Thus, 3D planning tools allow the 3D anatomy to be more accurately incorporated into the planning process than with conventional techniques. The computer allows the planner to use beam orientations that are nonstandard (e.g., nonaxial beams). Beam apertures are typically smaller than with conventional simulation due to reduced uncertainty in the entire process. Current technology also allows data from other imaging modalities (MRI, positron emission tomography [PET], etc.) to be fused with the planning CT dataset and hence considered in the planning process. It is advantageous to position the patient similarly during the imaging and treatment planning scans to facilitate accurate image correlation.
Intensity modulated radiation therapy (IMRT) requires the clinician to explicitly delineate target volumes, including elective nodal basins and avoidance structures. The introduction of IMRT has revolutionized radiation treatment planning, and in the process, has required clinicians to become more proficient in 3D anatomy and malignant patterns of spread. Several groups have published guidelines demarcating elective nodal stations on axial CT images, including head and neck cancer,1,2 lung cancer,3 anorectal cancer,4 and others. These stations are somewhat artificial but facilitate rational demarcation of nodal stations at risk, reporting of patterns of spread and failure, and communication with surgical colleagues.
One of the risks of 3D and IMRT is a false sense of security in the accuracy of imaging to portray the in vivo extent of disease. Further, imaging obtained at the time of initial treatment planning may not be representative of the in vivo anatomy throughout a multiweek course of therapy. Indeed, there have been several published examples of inferior outcomes with highly conformal treatment planning.5,6 Modern imaging tools are clearly not perfect. The rapid embrace of newer technologies to visualize gross tumor and to address uncertainties related to organ motion and setup errors may be counterproductive if relied on too heavily in the treatment planning process. It is likely that we have, in the past, been able to sterilize microscopic tumor at the edge of radiotherapy fields that were expanded to account for setup errors and organ motion (i.e., not expanded with the intent of covering the microscopic disease). Furthermore, the clinical history or examination and imaging can be discordant, and one needs to be careful not to be overly reliant on imaging when defining target volumes. For example, in a patient with cancer of the nasopharynx with cranial nerve deficits, the target volume should include the corresponding anatomic site of likely extension, even in the absence of an abnormality on imaging. Thus, a sound understanding of oncologic anatomy and imaging modalities is vital in the treatment planning process.
FIGURE 30.1. Gross disease identified with imaging is depicted, along with appropriate expansions to encompass surrounding microscopic disease (CTV) and setup/motion uncertainties (PTV).
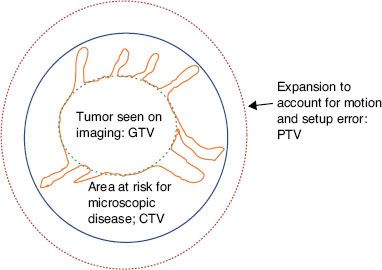
TABLE 30.1 VOLUME DEFINITIONS FOR RADIATION THERAPY PLANNING
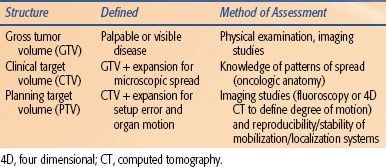
IMAGING MODALITIES
Radiologic imaging is an integral component in the management of cancer patients. Imaging is utilized in the diagnosis and initial staging of disease, treatment planning, and posttreatment surveillance. Radiation oncologists must be familiar with the available imaging modalities and understand the appropriate utilization and limitations of each, which often varies based on the tumor site and study indication. In particular, a general sense of the sensitivity, specificity, and positive and negative predictive values of an imaging study helps the clinician assimilate and interpret imaging information that can be misleading or even contradictory. A detailed review of each imaging modality and its associated physics is beyond the scope of this chapter. However a general overview of the imaging modalities most frequently utilized in clinical practice is provided, with disease-specific applications addressed in the systems-based anatomy sections.
Radiography
Conventional radiography creates a two-dimensional gray-scale image produced by the differential attenuation of x-rays that pass through soft tissues of varying density. Tissues that are very dense, such as bone, will absorb more x-rays than tissues that are less dense, such as lung. Radiographs are therefore best suited to detect pathology when a lesion differs greatly in density from adjacent structures, such as a soft tissue mass surrounded by aerated lung or a lytic lesion surrounded by dense bone. Radiographs have excellent spatial resolution: the ability to detect a small object within a given volume. However, they are suboptimal when there are only subtle differences in tissue density. Therefore, even large lesions can be missed if they are of similar density to surrounding structures. Given this and other limitations, additional imaging modalities are often obtained to supplement plain radiographs. In addition, although conventional radiography has limited utility in oncologic imaging, the basic principles underlie more advanced imaging modalities such as CT.
Cross-Sectional Imaging
CT, MRI, and ultrasound (US) generate two-dimensional cross-sectional images. The benefits of cross-sectional imaging include visualization of superimposed structures obscured on planar images, improved anatomic detail of individual organs and their precise relationship to adjacent structures, and the ability to perform multiplanar reconstructions. Furthermore, some applications provide functional in addition to morphologic information and can be acquired in real time, permitting image guidance for procedures. For these reasons, cross-sectional modalities are the mainstay of oncologic imaging.
Computed Tomography
CT generates cross-sectional images from the transmission of radiation through tissue. A patient lies on the scanner table within a gantry that houses an x-ray generator opposite multiple rows of detectors, hence the term multidetector CT (MDCT). Current generation scanners (e.g., 64 or 128 MDCT) are able to acquire high-resolution image data much faster due to improvements in the number of detectors and computer processing. As the gantry rotates, the detectors measure x-ray transmission through the rotation, or slice. The patient is moved through the scanner as the gantry rotates, resulting in a helical or spiral course at a very thin slice thickness, typically 0.625 mm. The spatial and temporal data from multiple projections are then processed by a Fourier transform mechanism generating two-dimensional axial images. The thin-slice volume dataset is isotropic, meaning that images can be reconstructed in orthogonal and oblique planes without a loss in image quality. Furthermore, thin-slice acquisition improves contrast resolution and decreases partial volume artifacts, thereby improving imaging quality and accuracy.
Images are displayed within a matrix composed of voxels, each representing a volume of radiodensity that is quantified by a linear attenuation value called a Hounsfield unit (HU). Each voxel is assigned a HU in the range of –1,000 to 1,000 corresponding to a shade of gray to represent the attenuation difference between a given material and water. By convention, air is the least dense material with a HU value of –1,000, while water has a HU value of 0. Soft tissues have a range of attenuation with typical HU values as follows: fat (–120), blood (30), muscle (40), bone (>300). HU analysis is more accurate than visual assessment of tissue composition and is particularly useful in characterizing enhancement postcontrast administration, a feature critical in the assessment of many solid organ lesions.
Both intravenous (IV) and oral contrast agents may be utilized to improve spatial resolution. Oral contrast agents are routinely used for abdominal and pelvic imaging to distinguish bowel from adjacent organs, lymph nodes, and tumors. The use of an intravascular contrast agent during CT depends on the study indication, target organ, and patient status. IV contrast agents contain variable concentrations of iodine compounds that attenuate, or absorb, x-rays, which allows for enhanced detection of vascular structures. Administration of IV contrast media is required for thorough assessment of vessels (e.g., aorta, pulmonary arteries), solid organs (e.g., liver, kidneys), and characterization of lesion vascularity. Contrast-enhanced CT is often necessary to detect solid organ metastases (e.g., liver, adrenal gland, brain). Contrast is usually not necessary for routine pulmonary imaging due to the inherent contrast of solid lesions within a background of aerated lung, although it does improve the characterization of hilar lymph nodes.
Given that the administration of contrast media can alter tissue attenuation, the HU value of a lesion or tissue may differ depending on whether the study was performed with or without contrast and based on the timing of image acquisition (e.g., arterial vs. portal venous phase). HUs are used during radiation treatment planning dose calculations; therefore, contrast can affect these calculations. If indicated, the HU within a structure enhanced by contrast (e.g., the bladder when planning for prostate cancer treatment) can be set to an alternate value prior to dose calculations. A similar phenomenon often occurs when materials with a high atomic number are within the scanned volume. These materials (e.g., dental fillings, hip prostheses) can cause artifacts that can make it challenging to accurately segment the image or affect dose calculations. The latter can also be corrected by setting the HU within the affected area to the desired value.
IV contrast agents are excreted through the kidneys, are nephrotoxic, and are not typically administered to patients with impaired renal function (glomerular filtration rate [GFR] <60, creatine [Cr] <1.8) unless on dialysis or out of emergent medical necessity. IV contrast media should also not be given to patients with a known contrast allergy resulting in anaphylaxis or laryngeal edema. More minor reactions, such as pruritus, are not an absolute contraindications and contrast may be administered following a proper steroid pretreatment protocol. Alternative imaging modalities should be considered if a contrast-enhanced study is required in the setting of a severe contrast allergy. An allergy to shellfish is no longer considered a contraindication to iodinated contrast administration.7
In part due to its availability, rapid acquisition, and high-yield anatomic data, CT has become one of the most widely used medical imaging modalities in the United States, with over 70 million scans performed annually, and it serves as the core modality for oncologic imaging.8 Although CT is noninvasive, it is not entirely benign. CT utilizes radiation to generate images, and although dose modulation and optimal scanning parameters can significantly reduce patient radiation exposure, the cumulative effects of CT radiation are of clinical concern. Alternative imaging modalities should always be considered and performed in lieu of CT when appropriate.
Magnetic Resonance Imaging
MRI generates cross-sectional images without ionizing radiation. MRI utilizes strong magnets, typically 1.5 or 3.0 T for clinical applications. A 3.0 T magnet is 60,000 times greater than the earth’s magnetic field. The magnetic field uniformly aligns the nuclei of hydrogen protons within tissue. Applying a radiofrequency (RF) pulse sequence and gradient to the magnetic field disrupts this alignment and equilibrium. When the RF pulse is removed, the protons realign, or relax, within the field and emit a measurable resonance radio signal. The detected radio signals, referred to as echoes or spin echoes, are then used to generate an image. The most important tissue properties for image generation are the proton density, the spin-lattice relaxation time (T1) and the spin-spin relaxation time (T2). Different tissues have different proton density and relaxation times, absorbing and releasing radio wave energy at different rates, which in part accounts for the high tissue contrast obtained by MRI.
Different RF pulse sequences can accentuate different tissue characteristics by varying parameters such as the repetition time (TR)—the time between RF pulses in the sequence, which determines how much time protons have to realign within the magnetic field—and the echo time (TE)—the time between the RF pulse and the peak returning signal. TR and TE dramatically affect image contrast and determine which tissue properties are selected. T1-weighted images, in which fluid is dark and fat is bright, are generally good at depicting anatomy; T1-weighted images are generated by selecting short TR (typically ≤800 ms) and short TE values (≤30 ms). T2-weighted images, in which fluid is bright and fat is dark, are fluid-sensitive and can depict areas of pathology; T2-weighted images are generated by selecting long TR (≥2,000 ms) and long TE values (≥60 ms) (Table 30.2). Scan sequences are composed of variations in RF pulses and TR and TE settings. Although these vary by MRI scanner manufacturer using proprietary names, the underlying principles are similar. Common sequence techniques include:
Spin-echo (SE) sequences produce standard T1- and T2-weighted images.
Multiple spin-echo (MSE) sequences allow for faster image acquisition and are also referred to as turbo spin echo, fast spin echo, or rapid-acquisition relaxation-enhanced imaging. The signal intensity and image quality is less than that of conventional SE sequences. Furthermore, fat is bright on MSE T2-weighted images, which can limit sensitivity for detecting pathology. Fat-suppression techniques can be used to offset this limitation. Fast low-angle acquisition with relaxation enhancement and half-Fourier acquisition single-shot turbo spin-echo sequences are MSE variations.
Inversion recovery (IR) pulse sequences emphasize differences in T1 properties of tissues. A time of inversion (TI) is added to the sequence that can be set to target specific tissues. Short time of inversion recovery sequences suppress tissues with short T1 relaxation times, such as fat, and enhance tissues with high T2 properties, such as fluid, resulting in added tissue contrast. The opposite effect may be achieved with fluid-attenuation inversion recovery sequences. In addition to IR, tissue suppression may be achieved with fat or fluid saturation and opposed-imaging techniques.
Gradient-recalled echo (GRE) pulse sequences are used for rapid image acquisition, which minimizes motion artifact associated with breathing, the cardiac cycle, vessel pulsation, and bowel peristalsis. GRE sequences have low tissue contrast, with the exception of flowing blood, which is bright in signal, and are therefore ideal for cardiac and vascular applications. Fast low-angle shot (FLASH) and true fast imaging with steady-state precession (FISP) are examples of this technique.
Although the inherent tissue contrast with MRI is excellent, the administration of contrast media can further improve the detection of pathology and subtle differences in tissue properties. Gadolinium chelates are the most commonly used MRI contrast agents, which, like the iodinated CT equivalents, are confined to the vasculature and do not cross an intact blood–brain barrier. Gadolinium is a heavy metal ion with paramagnetic effects that shorten T1 and T2 relaxation times. Although there are flow-related techniques for vascular imaging that do not require contrast media (e.g., time of flight imaging), the use of a contrast agent is essential for characterizing tissue perfusion. Once considered safe for patients with impaired renal function, the use of gadolinium-based contrast agents have now been associated with nephrogenic systemic fibrosis (NSF), a debilitating and potentially fatal condition of fibrin deposition within the skin and other organs.9 Although the precise mechanism is not understood, patients with severe renal dysfunction (GFR <30) who receive gadolinium-based contrast media are at increased risk of developing NSF.
One of the advantages of MRI over CT is that it can provide functional in addition to anatomic information. This is particularly beneficial in oncologic imaging. MRI techniques allow for tissue diffusion and perfusion imaging, quantification of blood flow by velocity phase encoding, and magnetic resonance proton spectroscopy, which provides biochemical quantification of tissues.
As with other imaging techniques, MRI has modality-specific artifacts that can limit image quality. Motion artifact can be problematic with MRI due to long scan times. Chemical shift artifact results in a loss of signal at the interface of tissues with highly variable contrast properties. MRI is also highly sensitive to magnetic field distortions that can produce artifacts. Susceptibility artifact is one of the most common problems with MRI and is frequently attributable to objects that alter the magnetic field, resulting in signal voids or distortion of MRI images, such as metallic hardware or devices. The magnetization of such objects also presents a safety hazard as items can overheat or become displaced, resulting in serious harm or injury. Patients must be carefully screened to ensure that any medical devices or surgical hardware are MRI compliant.
TABLE 30.2 MAGNETIC RESONANCE IMAGING SIGNAL INTENSITIES (SPIN-ECHO IMAGING)
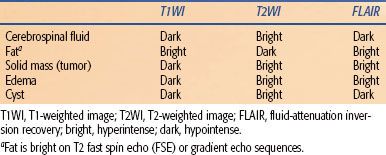
Ultrasonography
US is an imaging modality utilizing pulse-echo techniques rather than radiation to produce an image. The US transducer coverts electrical energy into a high-frequency pulse that is transmitted through tissues. The pulse interacts at tissue interfaces, generating a reflected echo signal that is detected by the transducer. The returning sound waves are transformed into a gray scale image in real time. Image quality is, in large part, determined by the pulse frequency. High-frequency transducers (5–12 MHz) produce high-resolution images but have limited ability to penetrate. Therefore, they are best suited to imaging superficial structures such as the breast or thyroid. Low-frequency transducers (1–3.5 MHz) generate lower quality images but have better tissue penetration and are most often used for imaging abdominal and pelvic organs. The degree to which tissues are visualized by US is called echogenicity. Fat is highly echogenic (bright), whereas fluid-containing structures, such as simple cysts, are anechoic (dark).
The quality of US images is highly dependent on the sonographer. Variability in user experience can be problematic in the performance, reproducibility, and interpretation of US exams. Furthermore, US is prone to artifacts. Bone almost completely absorbs sound waves, resulting in acoustic shadowing that completely obscures tissues located beyond the bone. Air is also problematic as it almost completely reflects sound waves, leaving little pulse energy to penetrate tissues deep to the air. The application of a water-soluble gel between the transducer and the patient’s skin eliminates air at the skin surface and ensures transmission of the US beam. Artifacts secondary to air markedly limit evaluation of lesions near the lung or bowel. In contrast to air, fluid readily transmits sound and makes an excellent “acoustic window” for imaging. This is the reason that patients are encouraged to drink plenty of fluids prior to a pelvic US examination—so that the bladder is fully distended.
TABLE 30.3 ENDOSCOPIC ULTRASOUND WALL LAYERS OF THE ESOPHAGUS
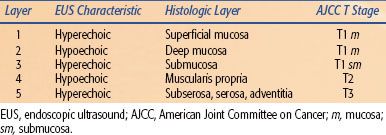
FIGURE 30.2. Axial endoscopic ultrasound image (right) and histologic specimen (left) from a normal esophagus. The endoscopic ultrasound layers and histologic layers of the esophagus are correlated (see Table 30.3). (Endoscopic ultrasound image courtesy of Dr. Frank Gress. Histologic image courtesy of Dr. Daniel Goodenough.)
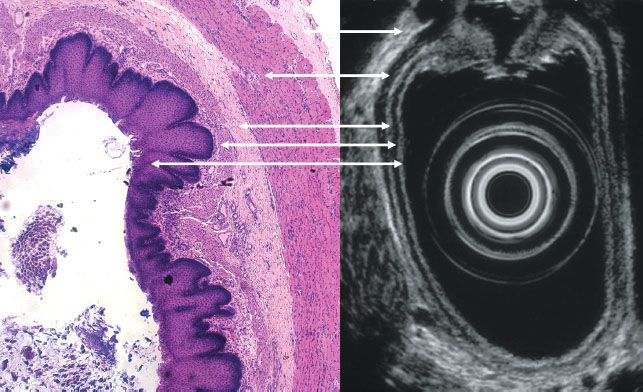
Color Doppler US is an important adjunct to conventional gray-scale sonography. The Doppler effect is a change in frequency of returning sound waves reflected by a moving object, such as flowing blood. If blood flows away from the transducer, the echo frequency decreases; whereas if blood flows toward the transducer, the echo frequency increases. The change in frequency is directly proportional to the flow velocity and produces a color overlay in areas of flow on the standard gray-scale US image. Color Doppler US is useful in characterizing blood flow within lesions and assisting in image-guided procedures.
Endoscopic ultrasound (EUS) was introduced in the early 1980 s and has become a tool important in oncologic staging. It allows for high-resolution images of internal structures not typically accessible by high-frequency transducers by passing the probe through bowel or airways. It is most widely applied in the setting of gastrointestinal (GI) malignancies, especially esophageal and rectal carcinomas. A 5 to 12 MHz transducer can readily identify five of the layers of the gastrointestinal tract (Table 30.3 and Fig. 30.2).10 Higher frequency transducers can identify additional layers, such as the muscularis mucosa and lamina propria of the esophagus, which has important staging implications. EUS is also utilized for characterization and image-guided sampling of regional lymph nodes in GI or bronchopulmonary disease. The ability of EUS to predict the tumor (T) stage is generally superior to its ability to predict the node (N) stage, although some imaging patterns of nodal involvement are recognized. Normal lymph nodes are usually ovoid, <10 mm in short axis, and have a homogeneous but variable echogenic appearance that may be isoechoic, hyperechoic, or hypoechoic to surrounding tissues. An echogenic (bright) center is common and represents the normal fatty hilum. Suspicious lymph nodes are typically round, >10 mm in short axis, have distinct margins, and are typically hypoechoic. If all four features are present, the likelihood of malignancy is 80% to 100%.11 There is, however, considerable overlap between benign and malignant features of lymph nodes on EUS in addition to wide interobserver variability. Tissue sampling is therefore recommended for accurate staging. When describing clinical T and N staging by EUS, the prefix u should be utilized (e.g., uT3N1).
Nuclear Imaging
Although radiographic and cross-sectional studies provide important anatomic information regarding pathologic processes, nuclear radiology provides physiologic information based on the distribution of an injected or ingested radiopharmaceutical. Radiopharmaceuticals consist of a radioactive substrate (radionuclide, radioisotope, or radiotracer) that is coupled with a physiologically active compound or analog. For example, technetium-99 m is a radioisotope that is coupled to pertechnetate, an iodine analog, which can enter thyroid follicular cells. The timing of imaging depends on the kinetics of absorption, metabolism, and half-life of the radionuclide. Gamma rays emitted by nuclear decay of the radionuclide are then detected using a γ-camera corresponding to radiotracer activity that is described in terms of uptake.
There are numerous available nuclear imaging studies that take advantage of differing radiopharmaceuticals for oncologic imaging. Many of these play a primary role in the management of oncology patients with specific malignancies. For example, indium-111 capromab pendetide (ProstaScint) can be utilized for prostate cancer and gallium-67 can be used for lymphomas—both of which will be discussed in greater detail later in the chapter. However, the primary nuclear imaging studies relevant to general oncologic imaging are PET and bone scintigraphy. These studies have broad application for many malignant processes in the diagnosis, staging, and surveillance of disease.
Positron Emission Tomography
Although several radionuclides for PET are available, the most common is 18-fluorodeoxyglucose (FDG). FDG is a glucose analog that concentrates in areas of high metabolic activity. Tumor cells are often highly metabolic, with rapid cell division and an increased number of glucose transporters. However, FDG uptake is not specific for malignancy and accumulates in any cell with increased metabolic activity, including myocardium, gastric mucosa, brain tissue, thyroid, and salivary glands, which limits evaluation of these organs. Furthermore, FDG tracer is excreted within the urinary system; therefore, activity within the kidneys, collecting system, and bladder can obscure malignancy of these structures. Notwithstanding these limitations, PET-CT has become the preferred imaging modality for clinical staging, facilitating the characterization of benign versus malignant pathology, detecting sites of unsuspected disease, identifying optimal sites for tissue sampling, assessing treatment response, and monitoring for recurrence for multiple malignancies.
Initially performed in isolation, PET is now routinely obtained in conjunction with CT. PET-CT combines the physiologic assessment of PET with the anatomic assessment of CT, resulting in improved diagnostic accuracy.12 PET and CT may be obtained independently on the same day but is more commonly performed on a combined PET-CT scanner, which more precisely aligns the two imaging datasets for fusion as the patient does not have to move between examinations. Patients must fast for 4 to 6 hours prior to scanning in order to limit metabolic activity within the GI tract. Blood glucose levels should be well controlled (<150 mg/dL) to limit glucose receptor competition with FDG, as high glucose levels can result in a false-negative scan. Speech and motion should be restricted to minimize muscle uptake, which could obscure pathology. Approximately 1 hour following FDG administration, a CT scan is performed immediately followed by PET imaging, which can take up to 60 minutes. CT and PET datasets are then reconstructed in separate axial, coronal, and sagittal series as well as fused PET-CT images.
FDG uptake is nonspecific, localizing to any tissue with increased metabolic activity. Although most malignant tumors are hypermetabolic relative to normal tissues, nonmalignant processes also concentrate FDG, including foci of infection, inflammation, and benign neoplasms. FDG uptake is quantified by the standard uptake value (SUV). Most malignant tumors have a maximum SUV >2.5, while physiologic uptake is typically <2.5. SUVs are not absolute and can be affected by the timing of imaging, improper attenuation correction, partial volume affects, patient weight, FDG dose, and factors affecting FDG uptake, as previously described. It is therefore difficult to accurately compare SUVs between scans. However, if care is taken to ensure that variables between studies are similar, such as performing the examination on the same scanner with similar patient preparation, FDG dosing, and image timing, comparing SUVs between studies may be reliable. Clinical studies to date have documented that under such uniform conditions, changes in SUV have prognostic value, indicating that most tumors responding to therapy show a 20% to 40% decrease in SUV early in course of treatment.13–15
Bone Scintigraphy
Normal bone undergoes continuous remodeling, maintaining a delicate balance between osteoblastic and osteoclastic activity. Most bone metastases originate as intramedullary lesions, having gained access to the bone through the vasculature. As the lesions enlarge, reactive osteoblastic and osteoclastic changes result in characteristic radiographic changes indicative of bone metastases (sclerotic, lytic, or mixed lesions). Rapidly growing metastases tend to produce lytic lesions, while more slowly growing metastases typically produce sclerotic (or blastic) lesions. Metastases from multiple myeloma, thyroid cancer, and renal cell carcinoma are predominantly lytic, while blastic lesions are associated with breast and prostate cancers. The primary utility of bone scintigraphy in oncologic imaging is the detection of osseous metastatic disease.
Bone scintigraphy or bone scan imaging utilizes radiopharmaceuticals composed of bisphosphonates; the most common of which is the radionuclide technetium-99 m methylene diphosphonate (99mTc-MDP). 99mTc-MDP localizes to areas of new bone mineralization, which occurs in a wide array of bone pathology and is therefore highly sensitive to osseous disease, but is not very specific. Although a 30% to 50% reduction in bone density must occur before bone metastases are detected on radiographs, as little as 5% to 10% change is required to detect such on a bone scan.16,17 Furthermore, bone scans are relatively inexpensive, convenient, and visualize the entire skeleton, including sites that are difficult to assess on plain films (e.g., ribs, sternum, scapula, sacrum). Reported sensitivities range from 62% to 100% with similar specificity rates (78% to 100%).18
Two primary patterns of radiotracer activity can be associated with malignancy: increased or decreased activity. Increased uptake occurs in areas of increased blood flow and osteoblastic activity; this is a common finding in metabolically active tumors and small sclerotic metastatic foci. Decreased radiotracer activity occurs as a “cold” area on bone scan and is associated with lytic bone disease and aggressive tumors that outgrow their blood supply. Rapidly progressing and purely lytic disease are the main causes of false-negative findings on bone scintigraphy, while false-positive findings can be related to trauma, healing, benign bone tumors, or arthritic changes.
Bone metastases are considered “nonmeasurable” using the Response Evaluation Criteria in Solid Tumors.19 Although a decrease in the intensity of radionuclide uptake is often ascribed to a response to treatment and an increase is attributed to progressive disease, several points must be considered. First, tumor response may cause a “flare phenomenon,” resulting from increased activity secondary to new osteoblastic activity concomitant with new bone formation. This may be falsely attributed to progressive disease. Similarly, lytic lesions that were previously “cold” on bone scan can transform into “hot” spots (areas of uptake) after treatment. Second, rapidly progressive disease with overwhelming bone destruction without new bone formation can be misinterpreted as stable or responding disease on bone scan.
Many patients are at low risk of harboring occult osseous metastatic disease and, in the absence of symptoms, a bone scan can be omitted from the staging workup. In prostate cancer, only approximately 1% of patients with a prostate-specific antigen (PSA) <10 will have a positive bone scan.20,21 Patients with a Gleason score ≤7 and a PSA of 10 to 20 have a similar low risk of bone metastases.22 In these patients, a bone scan should be omitted in the absence of symptoms. Similarly, a bone scan may be omitted in asymptomatic patients with node-negative breast cancer and lung cancer. Although studies have shown that MRI may be more sensitive than bone scans, especially for vertebral metastases, whole-body MRI is impractical and bone scintigraphy is considered sufficiently sensitive that MRI should be reserved for equivocal bone scans in the context of high clinical suspicion or for patients with positive bone scans but low clinical suspicion. Like MRI, PET has been shown to be more sensitive than scintigraphy for the diagnosis of osseous metastatic disease with sensitivity of 91% and 75%, and specificity values of 96% and 95%, respectively.23 FDG-PET also provides earlier detection of metastases than bone scans, attributable to the fact that increased glucose metabolism in neoplastic cells occurs prior to increased osteogenesis (required for bone scintigraphy uptake). PET also has the advantage of providing more comprehensive imaging in that it can detect soft tissue metastases in addition to bone disease, whereas scintigraphy only evaluates osseous structures. As a general rule, patients with isolated osteoblastic metastases may be monitored by serial bone scans, while other clinical scenarios are likely best suited for PET.
BRAIN AND SPINE
Oncologic Anatomy
The Brain
The central nervous system consists of the brain and spinal cord. Both are covered with three meningeal layers—the dura mater, arachnoid mater, and pia mater. Meningiomas are the most common tumors arising from the meninges. They arise from arachnoid cells but are typically affixed to the underside of the dura mater. They are well-circumscribed tumors that do not typically invade the underlying brain parenchyma. Thus, when planning a course of radiation therapy, only minimal margins are required to account for surrounding microscopic disease extent in the direction of the brain parenchyma. Many meningiomas will have a linear area of enhancement on MRI, extending from the tumor along the dura. This so-called dural tail is generally not felt to represent direct extension of tumor but rather vascular congestion within the dura, leading to the characteristic enhancement.24,25 Thus, enlarging the target volumes to include this linear area of enhancement is probably unnecessary unless other imaging abnormalities are apparent (e.g., nodularity).
The arachnoid mater and pia mater are considered the leptomeninges with the intervening space (subarachnoid space) filled with cerebrospinal fluid (CSF). Cancer can breach the CSF space through several routes, including hematogenous spread (via the Batson venous plexus or the arterial system) or by direct extension. Once cancer breaches the subarachnoid space, the entire craniospinal axis is at risk, and tumor deposits can lead to increased intracranial pressure, cranial nerve deficits, radiculopathies, and seizures. Leptomeningeal involvement occurs most frequently with pediatric brain tumors (e.g., medulloblastoma), acute lymphoblastic leukemia, and some solid cancers (e.g., breast cancer, melanoma, and lung cancer in particular).26
The major units of the brain include the cerebral hemispheres, diencephalon, cerebellum, and brainstem. The cerebral hemispheres consist of the frontal, parietal, temporal, and occipital lobes, basal ganglia, and lateral ventricles. The diencephalon includes the thalamus, hypothalamus, and third ventricle. The brainstem consists of the midbrain, the pons, and the medulla oblongata. The fourth ventricle lies between the pons and the cerebellum. Although more than half of pediatric brain tumors arise in the posterior fossa (cerebellum and brainstem), the vast majority of primary brain tumors in adults arise in the cerebral hemispheres or diencephalon.
Cranial Nerves
The cranial nerves consist of 12 paired nerves whose nuclei (with the exception of CN I) are located in the brainstem and upper spinal cord. They are termed cranial nerves because they exit the cranium through foramina in the base of skull and are encased by sheaths formed from the meninges. Nerve pathways connecting the cerebral cortex with the cranial nerves are complex. Although spinal motor neurons are innervated by the corticospinal tracts, many of the lower motor neurons in the brainstem are innervated by the corticobulbar tracts. These upper motor neurons innervate the cranial motor nuclei bilaterally (with some exceptions), in contrast to the nerves within the corticospinal tracts, which largely cross in the medulla leading to contralateral innervation.
Multiple tumors, both benign and malignant, commonly involve the cranial nerves, leading to serious neurologic deficits. Some of the more common include meningiomas involving the optic nerves (CN II), vestibular schwannomas (CN VIII), parotid gland tumors (CN VII), nasopharyngeal carcinoma (multiple), and brainstem gliomas (multiple).
The classic presenting symptoms of diffuse pontine gliomas illustrate the intricate anatomy of the pons. A unilateral lesion in the ventral pons with involvement of the pyramidal tracts (upper motor neurons) might cause contralateral motor weakness in the arms or legs. In addition, cranial nerves within the pons might also be affected, unilaterally, without complete paralysis because of bilateral innervation of the cranial nerves by fibers in the corticobulbar tracts. Ataxia, denoting impairment of coordination without weakness, can be caused by involvement of fibers projecting from the pons to the cerebellum within the middle cerebellar peduncle. Through this pathway, the cerebellum receives a copy of the information for muscle movement that the corticospinal tracts relay to lower motor neurons, facilitating the complicated act of coordination.
The cranial nerves should also be carefully examined when evaluating a patient with nasopharyngeal carcinoma (NPC). One-fifth of patients with NPC present with symptoms of cranial nerve involvement (Table 30.4). The most commonly involved cranial nerves are the abducent nerve (CN VI) and the trigeminal nerve (CN V). CN VI originates in the ventral aspect of the brainstem, ascends on the clivus, and crosses the internal carotid artery near the superior aspect of foramen lacerum before entering the cavernous sinus. It then exits the skull through the superior orbital fissure. The motor and sensory nerve roots of CN V exit the pons, pass underneath the free edge of the tentorium cerebelli into Meckel’s cave, forming the trigeminal (Gasserian) ganglion. From the ganglion, V1 and V2 enter the cavernous sinus and subsequently exit the skull through the superior orbital fissure and foramen rotundum, respectively. V3 exits the skull through foramen ovale.
The nasopharynx is in close proximity to foramen lacerum, rotundum, and ovale, explaining the frequent tumor involvement of CN V and VI. Invasion superiorly to the foramen lacerum and involvement of the trigeminal ganglion could lead to dysfunction in all three branches of CN V. In fact, most patients with NPC and CN V involvement have isolated deficits of V2 or V3. This occurs due to extension into foramen rotundum and ovale, respectively (Fig. 30.3). After gaining access to the middle cranial fossa, NPC may extend superiorly into the cavernous sinus. Four cranial nerves, including two branches of CN V, pass through the cavernous sinus. Within the sinus, the oculomotor nerve (CN III) is located most superiorly, while the maxillary nerve (CN V2) is located most inferiorly. One would suspect that cranial nerves located more superiorly in the cavernous sinus would be involved less frequently than those located closer to the base of skull. This is consistent with what is observed clinically (see Table 30.4).
Lower cranial nerve involvement can occur without intracranial extension. The fossa of Rosenmüller is the most common site of origin of NPC. The lateral border of the fossa of Rosenmüller is the pharyngeal space. Direct tumor extension laterally into the parapharyngeal space or lymphatic metastases to high parapharyngeal lymph nodes can affect the cranial nerves exiting the jugular foramen (CN IX, X, and XI), and hypoglossal canal (CN XII). This may result in loss of the gag reflex (CN IX), hoarseness or dysphagia (CN X), atrophy or paralysis of trapezius and sternocleidomastoid (CN XI), as well as tongue deviation (CN XII).
Spine
The vertebral column typically consists of 33 bones (7 cervical, 12 thoracic, 5 lumbar, 5 sacral, and 4 coccygeal). The sacral and coccygeal vertebral bodies are fused. Although all individuals have seven cervical vertebral bodies, variations in the number of the other vertebral levels are occasionally observed. The spinal cord is housed within the spinal canal and encased by the meninges. Spinal cord levels do not correspond with levels of the vertebral column. In adults, the spinal cord typically ends at the L1-2 interspace (termed the conus medullaris). In an infant, the spinal cord terminates at L2 or L3. However, the spinal nerves continue to descend within the spinal canal (termed the cauda equina). The subarachnoid space, containing CSF, typically extends to the second sacral vertebral body. At this level the meninges fuse together and extend caudally as the filum terminale, which anchors the spinal cord to the coccyx. These anatomical issues have implications when evaluating a patient with spinal cord compression, designing craniospinal fields, and when setting up palliative spine fields.
TABLE 30.4 MAJOR FORAMINA AND OTHER APERTURES IN THE CRANIAL FOSSAE AND THEIR PRIMARY CONTENTS AND CLINICAL ASSOCIATION WITH NASOPHARYNGEAL CANCER
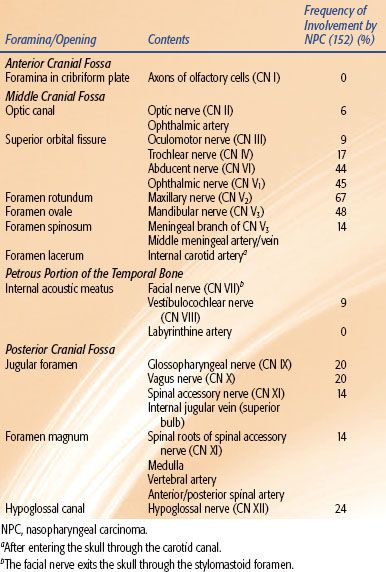
FIGURE 30.3. Coronal T1 magnetic resonance image with contrast demonstrating nasopharyngeal carcinoma extending through foramen ovale into Meckel’s cave.
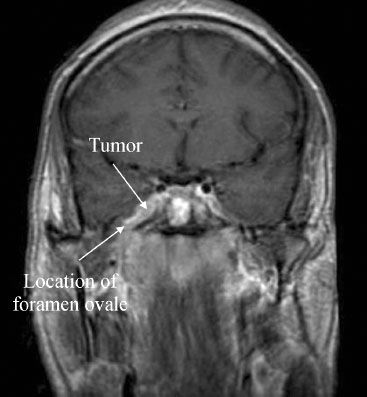
FIGURE 30.4. Axial T1 magnetic resonance image with contrast demonstrating a 2.5-cm melanoma brain metastasis in the left frontal lobe. Note the prior resection cavity in the right frontal lobe.
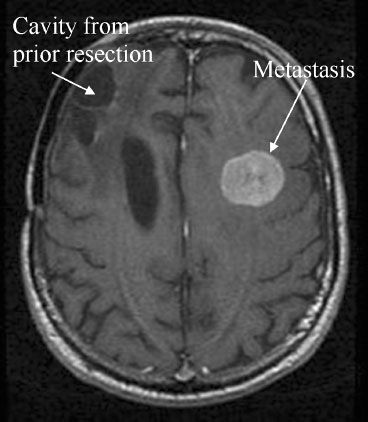
Oncologic Imaging
Intracranial Metastases
Approximately 50% of adult intracranial neoplasms are metastatic, with lung, breast, melanoma, renal, and colon cancers being the most common, in order of decreasing frequency. Among individual primaries, melanoma is associated with the highest frequency of brain metastases.27 Most metastases gain access to the brain through the vasculature and typically arise at the junction of the gray and white matter,28 presumably because the caliber of blood vessels decreases at this point, acting as a trap for tumor emboli. Approximately 80% of brain metastases are found in the cerebral hemispheres, with less frequent involvement of the cerebellum (15%) and brainstem (5%), reflecting their smaller volume and blood flow.
The detection of brain metastases is an important part of initial staging. Furthermore, due to improved treatment strategies and overall cancer survival, the overall incidence of brain metastases is increasing. The preferred imaging modality for the diagnosis of intracranial metastasis is contrast-enhanced MRI. Brain metastases are typically well circumscribed and avidly enhance (Fig. 30.4). They are also typically associated with a disproportionate amount of surrounding edema best depicted on T2-weighted sequences. MRI is more sensitive than CT in detecting intracranial metastases, and approximately 20% of patients with solitary metastatic lesions by CT show multiple lesions on MRI.29 Furthermore, for patients with a single brain metastasis detected with a conventional single-dose MRI-contrasted study, triple-dose studies will depict additional metastases in up to 25% of patients.30 The detection of multiple lesions aids in directing appropriate treatment and helps to distinguish metastases from primary brain tumors, which are more commonly solitary.
Conventional PET-CT has limited utility in the assessment of brain metastases due to the high metabolic rate of normal brain tissue. However, delayed phase PET, performed approximately 3.5 hours following FDG injection, allows for washout of FDG from normal brain cells, while abnormal tissue retains FDG. Delayed phase PET has been shown to be beneficial in detecting both primary and metastatic brain lesions as well as differentiating between residual or recurrent tumor and radiation necrosis following treatment.31,32 The utility of delayed phase PET may be further enhanced through fusion with MRI.
Primary Brain Malignancy
Primary brain tumors account for approximately 50% of adult intracranial masses, of which half are malignant. The most common malignant primary brain tumor in adults is glioblastoma multiforme (GBM), which usually arises in the cerebral hemispheres. Compared with CT, MRI is more accurate in delineating the local gross extent of disease. In a series of 52 patients with primary brain tumors, the MRI signal abnormality was larger than that identified by CT in 62% of patients. Furthermore, 10 patients with equivocal CT scans had clear abnormalities on MRI.33 GBMs are characterized by an expansile mass that is iso- to hypointense to surrounding tissue on T1-weighted images, with central necrosis and peripheral ring enhancement postcontrast administration. T2-weighted imaging shows a heterogeneous mass with high signal of the tumor nidus and surrounding T2-signal abnormality corresponding to vasogenic edema, in which malignant cells are known to frequently reside.34 Central areas of low signal reflect a combination of blood products, necrosis, and vascular flow voids.
GBM most commonly grows along white matter tracts and does not typically involve the dura or skull. GBM is one of two entities that may spread into the contralateral cerebral hemisphere via tracts of the corpus callosum; central nervous system lymphoma is the other. Other potential routes of spread, including subependymal extension with CSF contamination or hematogenous dissemination, are possible but unusual.
Advanced imaging techniques are also beneficial in characterizing intracranial malignancy. Magnetic resonance spectroscopy (MRS) provides metabolic and biochemical information about tumors in relation to normal brain tissue by quantifying five metabolite peaks: choline (Cho)-containing compounds, Cr, N-acetylaspartate (NAA), lactate, and lipid: the Cho peak reflects cell membrane turnover, Cr is a surrogate for energy synthesis, and NAA is a marker exclusive to neuronal cells. Lactate is detected in necrotic tumors and infarcted tissue and results from anaerobic metabolism. Lipid peaks are produced by cellular and myelin breakdown products. The hallmark spectroscopic pattern of brain tumors is an increase in Cho-containing compounds and a decrease in NAA relative to normal brain tissue. MRS can also aid in the evaluation of tumor type and grade. High-grade gliomas tend to exhibit higher Cho:Cr and Cho:NAA ratios. High-grade gliomas also typically have higher lipid and lactate peaks as the result of necrosis.35 MRS can also distinguish metastatic disease from high-grade gliomas, particularly when combined with perfusion MRI.36 Differentiating radiation necrosis from tumor is also possible using MRS; however, newer techniques, such as dynamic susceptibility-weighted contrast-enhanced perfusion MRI, may be even more accurate and are currently being studied.37
Spinal Metastases
Osseous metastases are common in many malignancies, and the vertebral bodies are frequently involved due to the vascular distribution to the spine. MRI is more sensitive at detecting early vertebral body metastases than both radiographs and CT as it can image changes within the marrow space before cortical destruction occurs. Given that PET has similar sensitivity as MRI for osseous metastatic disease, is performed as part of routine staging, and can image the entire body, MRI is reserved for troubleshooting, such as determining extent of invasion into adjacent tissues. One notable exception is the use of urgent MRI for identification of cord compression in the setting of acute neurologic compromise. In this situation, it is often the T2-weighted sequences that are most useful: the high-signal CSF provides contrast to identify disease encroaching into the spinal canal. Spinal cord edema can also be identified. One should consider screening the entire spine when evaluating for cord compression.
Spinal cord compression is often considered an oncologic emergency, because the longer the duration of symptoms, the less likely the patient will regain function. Thus, therapy is often instituted emergently in these cases (e.g., with radiation, steroids, surgery, or sometimes chemotherapy in sensitive tumors). There is often discordance between the imaging and clinical examination findings. It is the clinical findings that determine the acuity of the situation rather than the imaging findings. A radiologic finding of cord compression certainly warrants an evaluation but does not necessarily warrant emergent intervention.
HEAD AND NECK
Oncologic Anatomy
The anatomy of the head and neck is complex, and it is important that oncologists have an intimate understanding of the location, function, and radiographic appearance of the various subsites. The potential for significant morbidity associated with local tumor progression and recurrence in the head and neck region, as well as the associated late effects of curative treatment, necessitates attention to detail. The impact of anatomy is such that the Accreditation Council for Graduate Medical Education mandates cadaver lab experience for otolaryngology residency.
Lymphatic Drainage of the Head and Neck
The lymphatics in the head and neck region largely proceed in an orderly fashion from sites in the upper aerodigestive tract into the common jugular chains, which eventually empty into systemic circulation near the junction of the internal jugular and subclavian veins. There are notable exceptions to this orderly drainage that can influence radiation treatment planning. In order to discuss this in a systematic fashion, the lymphatic basins of the neck are divided into six distinct levels, with surgical and radiographic boundaries.38
Level I is defined as both the submental basins (level IA) and the submandibular basins (level IB) and receive drainage from the oral cavity, although this basin may also be at risk from other sites in the setting of advanced nodal disease (N2b or greater). Level II contains the upper jugular nodes, extending from the C1 vertebral body to the hyoid bone, including the contents of the carotid sheath and the space deep to the sternocleidomastoid muscle (SCM). Level II is subdivided into anterior (level IIA) and posterior (level IIB) regions, as defined by the posterior border of the jugular vein. Level II contains the jugulodigastric node at the level of the jugular vein as it crosses the posterior belly of the digastric muscle, and is a common lymphatic pathway for the majority of the upper aerodigestive tract. Level III follows the carotid sheath and space posterior to the SCM from the hyoid bone to the cricoid and receives efferent lymph drainage from level II. Level IV continues to follow the same jugular chain to the level of the clavicle. Level V contains the posterior triangle of the neck, boarded by the trapezius posteriorly, the SCM anteriorly, and the clavicle inferiorly and is at particular risk for nasopharyngeal primaries. Level VI are the prelaryngeal lymph nodes (Delphian nodes), which extend from the inferior edge of the thyroid cartilage to the sternal notch, bounded laterally by the sternal heads of the SCM. Level VI is at risk in laryngeal cancers with subglottic or transglottic extension and for hypopharyngeal cancers with esophageal extension.39
In addition, there are two other regions not included in this level classification that are worthy of consideration. Just superior to level II, following the carotid sheath to the skull base are the junctional or retrostyloid nodes, which may be at risk when there is ipsilateral nodal disease. Medial to these nodes and to level II lie the retropharygeal nodes (or nodes of Rouviere), which lie in the regions anterior to the prevertebral fascia, which in turn surrounds the longus capitis and the longus colli muscles. The retropharyngeal nodes extend superiorly to the skull base and inferiorly to the hyoid bone and are at risk of cancers arising from the nasopharynx, posterior pharyngeal wall, and the pyriform sinus.40
The Oral Cavity
The oral cavity encompasses three major subsites: the oral tongue, the floor of mouth, and the buccal mucosa. Carcinomas originating from the oral tongue have potential to spread locally through the intrinsic and extrinsic muscles of the tongue, inferiorly to the floor of mouth, and posteriorly to the anterior tonsillar pillar. For the floor of mouth, the genioglossus, geniohyoid, and root muscles of the tongue are at risk for local disease spread, as well as levels IA and IB of the neck, laterally to the alveolar ridge and mandible. Tumors of the buccal mucosa may extend superiorly to the infratemporal fossa, inferiorly to the submandibular region, anteriorly to the lip commissure, and posteriorly to the retromolar trigone.40
The Oropharynx
The oropharynx anteriorly is bounded by the base of tongue, laterally by the tonsils, superiorly by the soft palate, and posteriorly by the posterior pharynx. Tumors arising from the base of tongue are often difficult to assess for extent of disease; therefore, the entire base of tongue, proximal oral tongue, and vallecula are at risk for subclinical disease. MRI may aid in delineation of the gross tumor volume for this site.41 Tonsillar cancers may involve the base of tongue, palate, and buccal mucosa, although in locally advanced cases, they may also involve the nasopharynx, parapharyngeal space, and pterygoid muscles. Soft palate tumors may extend laterally to the tonsillar pillars and superiorly to the pterygopalatine fossa.
Nasopharynx, Oropharynx, and Hypopharynx
The nasopharynx is bounded superiorly and posteriorly by the sphenoid sinus, clivus, and the prevertebral fascia of C1 and C2. The parapharyngeal space lies laterally to the nasopharynx, which in turn is bounded laterally by the medial pterygoid muscle. The parapharyngeal space offers few anatomic barriers for the direct invasion of tumors superiorly and laterally to the base of skull, resulting in cranial nerve deficits as noted above. The eustachian tube empties into the nasopharynx at the torus tubarius; just posterior to the torus is the pharyngeal recess (or fossa of Rosenmüller), a fold of mucosa that is a frequent site for primary malignancies of this region.
Carcinoma arising from the posterior and lateral pharyngeal walls may spread superiorly to the nasopharynx and inferiorly to the hypopharynx. Similarly, primaries of the hypopharynx may spread superiorly through the oropharynx and nasopharynx. Malignancies of the pyriform sinus may also place the ipsilateral larynx at risk.
Larynx
The larynx is subdivided into three anatomic regions: the supraglottis (containing the epiglottis, the arytenoid and aryepiglottic folds, and the ventricular bands or false cords), the glottis (a 1-cm plane extending inferiorly from the lateral margin of the ventricle, including the true vocal cords and commissures), and the subglottis (from the inferior border of the glottis to the inferior aspect of the cricoid). The true cords have essentially no lymphatic drainage, allowing for focal treatment of a limited primary tumor without elective nodal treatment. In contrast, the supraglottic larynx has a rich and bilateral lymphatic system, requiring either dissection or elective nodal radiotherapy for even early primary tumors. The subglottis is a rare site of primary tumors and may invade locally into soft tissue as well as metastasize to laryngeal and tracheal lymphatics.42
Oncologic Imaging
For cancer of the head and neck, staging relies on careful physical examination, fiberoptic and direct laryngoscopy, directed biopsies, and integration of imaging modalities. Contrast-enhanced CT is the standard imaging modality for carcinomas of the head and neck region (Fig. 30.5). MRI may be a superior imaging modality for evaluating the extent of primary tumors of the oral cavity, oropharynx, and nasopharynx.43 Novel approaches with MRI have shown promise in evaluating tumor responses to treatment by measuring physiologic changes within the tumor. Dynamic contrast-enhanced MRI may give insight into the vascular permeability (k-trans) in the tumor microenvironment; k-trans was found to be significantly higher in complete responders to concurrent chemoradiation therapy in one series.44 Diffusion-weighted MRI may also provide information about the cellularity of a tumor mass or involved lymph node; an increase in the apparent diffusion coefficient (corresponding to a decrease in cellularity) during chemoradiation has been correlated with improved 2-year local control in another series.45
FDG-PET imaging for head and neck cancer has been useful as an adjunct to MRI and CT, particularly in clarifying the significance of intermediate lesions on MRI or CT. In one series, the addition of FDG-PET imaging improved the accuracy of tumor delineation from 40% to 70% with MRI or CT alone to 97% to 100%, altering the management of 23% of the patient under study.46 Another series found FDG-PET to have a sensitivity of 88% in determining the primary tumor and a sensitivity of 82% and specificity of 100% for lymph node metastases.47 However, in initial staging, no noninvasive imaging modality has a high enough negative predictive value to forgo appropriate dissection or elective nodal irradiation when the characteristics of the primary tumor suggest a significant risk of spread. That is in contrast to the value of FDG-PET after chemoradiation for the detection of residual nodal disease. The sensitivity for detection of persistent disease was 96% with a specificity of 72% in a posttreatment series, with a negative predictive value in some series as high as 99%, allowing for the abandonment of routine posttreatment neck dissections in patients with a complete clinical and PET response.48,49 Novel PET tracers such as Cu64-ATSM and F18-misonidasole, designed to evaluate hypoxia within the tumor, may also prove to be clinically useful.50
THE BRACHIAL PLEXUS
Oncologic Anatomy
The brachial plexus originates from the primary rami of the C5 through the T1 vertebral levels and joins to form three nerve trunks (upper, middle, and lower) in the neck. These trunks divide as they course beneath the clavicle, forming three cords (lateral, medial, and posterior) in close approximation to the axillary artery, posterior to the pectoralis minor. The cords then form the three primary terminal nerves for the arm (the median, ulnar, and radial nerves) as well as the musculocutaneous nerve. These peripheral nerves are of particular importance due to the significant morbidity associated with injury, either from tumor invasion or from treatment-related toxicity. As the plexus traverses the neck and axilla, it is of particular relevance when treating tumors of the head and neck, upper thorax, and breast.
Oncologic Imaging
Identifying the brachial plexus is difficult on CT imaging and may be most directly visualized on MRI.51 However, one may accurately contour the position of the trunks, divisions, and cords on noncontrast CT imaging based on bony, muscular, and vascular landmarks.52 The method put forward by Hall et al.52 begins contouring at the origination of the vertebral foramina of C5 through T1. The anterior and middle scalene muscles are then identified, with the trunks lying in the space between these muscles. The middle scalene will end at the superior aspect of the first rib just as the divisions of the plexus will be joining with the axillary artery as a neurovascular bundle. The artery then can be followed laterally as a surrogate for the remainder of the cords into the upper arm. Of interest to breast treatment, the course of the plexus will be brought superior and lateral with the upper arm with abduction, though still constrained by its course underneath the clavicle (Fig. 30.6).
FIGURE 30.5. Axial computed tomography image demonstrating a squamous cell carcinoma involving the right aryepiglottic fold with anterior extension into the pre-epiglottic space.
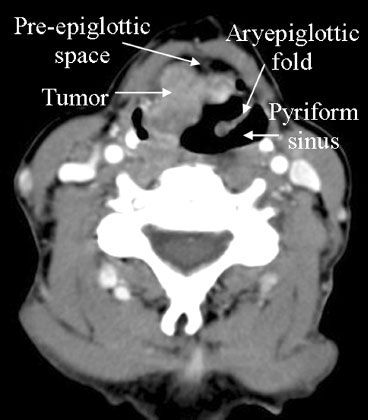
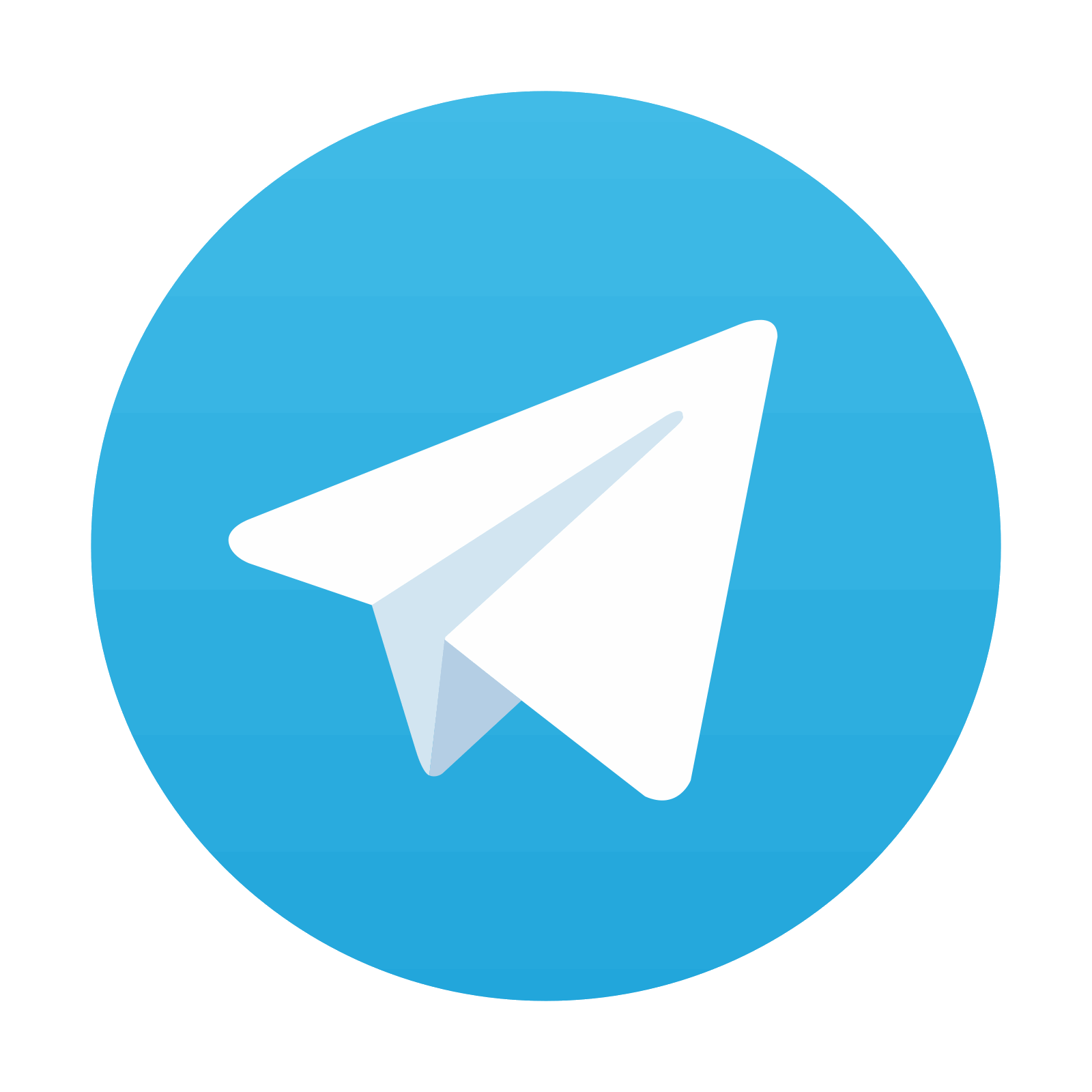