Nonthyroidal Illness Syndrome
Wilmar M. Wiersinga
Greet Van Den Berghe
Changes in the regulation of the hypothalamic–pituitary–thyroid axis and in thyroid hormone transport and metabolism are common in patients with nonthyroidal illness, collectively known as the nonthyroidal illness syndrome (NTIS). Illness in this context comprises virtually all nonthyroidal disorders, surgical and nonsurgical trauma, and inadequate caloric intake. Many patients with nonthyroidal illness also receive drugs that affect thyroid hormone regulation and metabolism, but for the sake of clarity pharmacologic interference is not considered an intrinsic part of NTIS.
The effects of illness are not confined to the thyroid axis; in fact, illness induces changes in many neuroendocrine systems (Fig. 11C.1). The changes in thyroid function are therefore only one component of the neuroendocrine response to illness and can be viewed as part of the general adaptation to stress (1). Whereas the acute stress response is considered beneficial, this is not necessarily true for the response in prolonged critical illness.
NTIS is sometimes referred to as the “sick euthyroid syndrome.” This name is less appropriate because it is unclear if patients are really euthyroid in all target tissues of thyroid hormone. NTIS occurs in humans and animals, and has been reviewed extensively (2,3,4,5,6); NTIS in animals is discussed here only to clarify aspects for which human data are lacking. Although NTIS is heterogeneous, as most evident from particular changes in patients with specific disease entities, the majority of illnesses induce a rather uniform pattern of changes.
Description of Nonthyroidal Illness Syndrome
Changes in Serum Thyroid Hormone and Thyrotropin Concentrations
NTIS in the Acute Phase of Illness
Within hours of the onset of acute stress (such as sepsis, surgery, myocardial infarction, or trauma) serum concentrations of triiodothyronine (T3) decrease and that of reverse triiodothyronine (rT3) increase (7,8,9). The fall in T3 and rise of rT3 are the most common changes in NTIS, often referred to as the low T3 syndrome. Few studies describe in detail the very early stages of NTIS. In patients who underwent elective abdominal surgery, a small significant increase of serum thyrotropin (TSH) occurs peaking at 0.5 hours after skin incision and lasting for 2 hours, followed by a transient 20% increase of serum total and free thyroxine (FT4); a sustained fall in total and free triiodothyronine (FT3) and rise of rT3 is observed as of 2 and 6 hours, respectively (10). The data support the sequence of events depicted in Fig. 11C.1.
NTIS in the Chronic Phase of Illness
The low serum T3/high rT3 pattern is found in patients with most acute and chronic illnesses, including infectious diseases, infiltrative and metabolic disorders, cardiovascular diseases, pulmonary diseases, gastrointestinal diseases, cancer, burns, and trauma (5,11,12). In general, serum FT3 falls to a lesser extent than does total T3. Serum rT3 increases to supranormal values in patients with mild illness and does not increase much more in patients with more severe illness. In contrast, serum T3 decreases further as the severity of illness increases (Fig. 11C.2). Serum T3 concentrations are low or even undetectable in patients with critical illness, and can remain persistently low in patients with chronic illnesses. The severity of the illness is in general reflected by the magnitude of the changes in serum T3 and rT3. For example, there is an inverse relationship between serum T3 and glycosylated hemoglobin values in diabetes mellitus, between serum T3 and serum creatinine in renal insufficiency, and between serum T3 and burn severity (13). Similarly, the size of myocardial infarction is inversely related to the fall in serum T3 and directly related to the rise in serum rT3 (8).
Patients with mild to moderate nonthyroidal illnesses usually have normal serum total and free T4 concentrations. Some severely ill patients have low serum T4 (Fig. 11C.2), and serum T4 concentrations are inversely correlated with the mortality rate. There has been uncertainty about serum FT4 concentrations in NTIS; the results vary depending on the assay method (see Chapter 13A). The issue is complicated by the decrease in serum T4-binding proteins that may occur in severe nonthyroidal illnesses. Furthermore, the serum of some patients with nonthyroidal illness contains substances that inhibit the binding of T4 to serum binding proteins (14). On the basis of the most accurate assays, it appears that most patients with nonthyroidal illness have serum FT4 within the normal reference range (15,16).
Serum TSH is usually normal in NTIS. However, some critically ill patients who have low serum T4 concentrations also have low serum TSH indicative of a poor prognosis (17), although TSH is rarely as low as in patients with thyrotoxicosis. The serum TSH response to thyrotropin-releasing hormone (TRH) is usually proportional to baseline TSH. Critically ill patients are often treated with dopamine infusions or high doses of glucocorticoids, both of which lower serum TSH. The prevalence of low TSH in NTIS is rather low. In patients admitted to an intensive care unit who underwent invasive mechanical ventilation, low TSH was observed in 7%, low FT4 in 24%, and low FT3 in 79% (18).
NTIS in the Recovery Phase of Illness
During recovery of illness a rapid rise of total and free T4 can be observed, associated with a marked increase in serum TSH. TSH concentrations may rise transiently above the upper normal limit in some patients, but rarely exceed the value of 10 mU/L. The TSH rise consistently precedes the T4 rise, suggesting an essential role of TSH in the return of T4 to normal. Normalization of serum T3 also occurs after the TSH rise (19,20).
NTIS and Nutrition
Fasting induces a fall in serum T3 and rise in serum rT3 within 24 to 36 hours, and the values return rapidly to baseline upon refeeding (21,22). The composition of the food is relevant: Refeeding with glucose is more effective in restoring normal values than refeeding with equicaloric amounts of protein or fat (11,23,24). Serum T4 does not change during fasting in healthy subjects, but decreases in patients with protein-calorie malnutrition (25). Serum TSH falls during fasting. To which extent nutritional deficiencies contribute to NTIS in critically ill patients, is unclear.
Changes in Thyroid Hormone Kinetics
Kinetic studies of thyroid hormone production and metabolism have been conducted in normal subjects during fasting and in patients with chronic renal failure, cirrhosis, diabetes mellitus, mild illness, and critical illness. The results can be summarized as follows (26). In patients with low serum T3, the production rate of T3 is decreased, but its metabolic clearance rate is unchanged. The decrease in T3 production is due to decreased extrathyroidal deiodination of T4 to T3, which normally provides ∼80% of the daily T3 production (Fig. 11C.3). The fractional rate of transport of T3 into tissues is unaltered. For rT3, the production rate is unchanged, but the metabolic clearance rate is decreased, due to decreased extrathyroidal deiodination
of rT3 to 3,3′-diiodothyronine. For T4, the metabolic clearance rate is usually normal, but it may be increased in patients with severe illnesses who have low serum T4, due at least in part to decreased production of one or more serum thyroid hormone–binding proteins. These severely ill patients also tend to have low serum TSH, and may have low T4 production rates. The fractional rate of transport of T4 from serum to tissues is reduced to ∼50% of the normal value.
of rT3 to 3,3′-diiodothyronine. For T4, the metabolic clearance rate is usually normal, but it may be increased in patients with severe illnesses who have low serum T4, due at least in part to decreased production of one or more serum thyroid hormone–binding proteins. These severely ill patients also tend to have low serum TSH, and may have low T4 production rates. The fractional rate of transport of T4 from serum to tissues is reduced to ∼50% of the normal value.
Changes in the Hypothalamic–Pituitary–Thyroid Axis
The failure of serum TSH to increase despite marked reductions in serum T3 and sometimes T4, suggests that the sensitivity of TSH secretion to low serum thyroid hormone concentrations is decreased in NTIS. This could be due to changes in the thyrotrophs or due to decreased TRH secretion. Evidence for the latter is a decrease in the amplitude (but not frequency) of the nocturnal pulses of TSH secretion; such decreases in pulse amplitude have been documented during fasting, after surgery, and in a wide variety of nonthyroidal diseases, including chronic renal insufficiency, diabetes mellitus, acute respiratory failure, infections, cancer, and critical illness (27,28). For example, fasting for 60 hours results in disappearance of the nocturnal TSH surge and a 50% reduction in 24-hour mean serum TSH concentrations (Fig. 11C.4). Among patients with nonthyroidal illnesses, the nocturnal TSH surge is decreased in at least half, and the decrease is not closely related to changes in serum T3 or free T4 (29). The pattern of 24-hour TSH secretion in these patients is similar to that in central hypothyroidism (Fig. 11C.4). If the abnormal pattern of TSH secretion in NTIS persists for a week or longer, then the T4 production rate should decrease, as it does in patients with central hypothyroidism.
In a study in deceased patients with nonthyroidal illness, the TRH messenger RNA (mRNA) content in the paraventricular
nucleus was directly correlated with serum TSH and T3 obtained just before death, but not with serum T4 or free T4 (Fig. 11C.5). In another study, the T3 content of the hypothalamus and anterior pituitary were, respectively, 64% and 46% lower in patients who died as a result of nonthyroidal illness, as compared with patients who died suddenly (30). Furthermore, the TSH that is secreted in patients with nonthyroidal illness is less glycosylated, and therefore less biologically active, than normal, a change that also occurs in patients with overt hypothalamic disease and TRH deficiency (31,32).
nucleus was directly correlated with serum TSH and T3 obtained just before death, but not with serum T4 or free T4 (Fig. 11C.5). In another study, the T3 content of the hypothalamus and anterior pituitary were, respectively, 64% and 46% lower in patients who died as a result of nonthyroidal illness, as compared with patients who died suddenly (30). Furthermore, the TSH that is secreted in patients with nonthyroidal illness is less glycosylated, and therefore less biologically active, than normal, a change that also occurs in patients with overt hypothalamic disease and TRH deficiency (31,32).
Little is known about the function of the thyroid gland itself in NTIS. The increase of serum TSH induced by exogenous TRH or that occurs during recovery from illness is followed by an increase in serum T3 and T4, indicating that the thyroid gland can respond normally to TSH. Thyroid weight is lower and thyroid follicular size is reduced in deceased chronically ill patients, as compared with subjects with sudden death (33), possibly related to decreased TSH secretion in severe nonthyroidal illness.
Table 11C.1 Similarities and Dissimilarities between Changes in Intermediary Metabolism and Peripheral Tissue Function Tests during Fasting, Hypothyroidism, and Illness | ||||||||||||||||||||||||||||||||||||||||||||||||||||
---|---|---|---|---|---|---|---|---|---|---|---|---|---|---|---|---|---|---|---|---|---|---|---|---|---|---|---|---|---|---|---|---|---|---|---|---|---|---|---|---|---|---|---|---|---|---|---|---|---|---|---|---|
|
Changes in Peripheral Thyroid Hormone Metabolism
Thyroid hormone is taken up in target tissues via thyroid hormone transporters, notably monocarboxylate transporter 8 (MCT8). Intracellular thyroid hormone is deiodinated via three iodothyronine deiodinases: Type 1 (D1) catalyzes inner- and outer-ring monodeiodination, type 2 (D2) catalyzes only outer-ring deiodination (converting its preferred substrate T4 into T3), and type 3 (D3) catalyzes only inner-ring monodeiodination (degrading its preferred substrate T3 into T2). Bioavailability of the active hormone T3 for binding to its nuclear T3
receptors (TR) is thus regulated to a large extent locally (34), and changes in each of these regulatory pathways have been described in NTIS.
receptors (TR) is thus regulated to a large extent locally (34), and changes in each of these regulatory pathways have been described in NTIS.
In critically ill patients, increased MCT8 but not MCT10 gene expression is observed in liver and skeletal muscle as compared with patients undergoing acute surgical stress (35). MCT8 mRNA was reduced in subcutaneous adipose tissue of patients with septic shock relative to patients undergoing knee or hip surgery (36); its significance is unknown.
In biopsies taken from intensive-care-unit patients immediately after death, liver D1 activity was decreased; D3 activity in liver and skeletal muscle (not detectable in healthy subjects) was induced, particularly in disease states associated with poor tissue perfusion (37). D1 and D3 mRNA levels corresponded with enzyme activities, suggesting regulation at pretranslational levels. Observed changes in D1 and D3 correlated with a low premortem serum T3/rT3 ratio. No changes in D1 and D3 mRNA were found in subcutaneous adipose tissue (36). Upregulation of D2 mRNA and activity occurred in skeletal muscle of prolonged, but not acute, critically ill patients (38), possibly in an attempt to reverse longstanding low T3 levels.
Changes in iodothyronine deiodination in NTIS also affect the serum concentrations of diiodothyronines: 3,5-T2 becomes elevated but 3,3′-T2 remains normal (34). Nondeiodinative pathways of iodothyronine degradation are frequently increased in NTI. Sulfated iodothyronines do not bind to TR, and sulfation mediates the rapid and irreversible degradation of iodothyronines by D1. Serum concentrations of T4 sulfate (T4S), T3S, and T2S are all increased in NTIS (39,40). Liver D1 in critical illness is strongly negatively correlated to serum T4S and with the T4S/T4 ratio, suggesting low D1 activity is involved in the rise of T4S. There may be increases in the products of ether-link cleavage, such as diiodotyrosine, and in the products of oxidative deamination of the alanine side chain of T4 and T3, which are, respectively, tetraiodothyroacetic acid and triiodothyroacetic acid.
The thyroid hormone content of tissues is decreased in NTIS. The mean T3 concentrations in the cerebral cortex, liver, kidney, and lung were lower by 46% to 76% in patients who died of nonthyroidal illness, as compared with those who died suddenly, but the values in heart and skeletal muscle were similar (30). The mean T4 concentration in the liver was 66% lower in the patients with nonthyroidal illness, but the values in the cerebral cortex were similar in the two groups. Tissue T4, T3, and rT3 levels in liver and skeletal muscle of critically ill patients are positively correlated with their respective serum levels. Whereas MCT8 expression was not related to the ratio of serum over tissue concentration, tissue rT3 and T3/rT3 ratio were correlated with tissue D1 and D3 activities (41).
There are few data on nuclear thyroid hormone receptors (TR) in NTIS. In liver biopsies from critically ill patients who died in the intensive care unit, the ratio of TRα1/TRα2 mRNA was higher in patients with more severe disease, due to a relative increase of TRα1 mRNA (42). A lower TRβ1 mRNA was found in skeletal muscle and subcutaneous adipose tissue of patients with septic shock in comparison with patients undergoing elective surgery (36). TR expression at the protein level was similar in normal livers and livers from patients with hepatic disease (43).
Most, if not all of the described changes would seem to indicate that nonthyroidal illness leads to reduction in the production of T3 and to lower bioavailability of T3 in tissues. This raises the important but still incompletely answered question of what the level of thyroid hormone action is in the tissues of patients with nonthyroidal illness. During fasting, oxygen consumption and protein breakdown decrease, so that energy is saved and organ function may be preserved. The lower T3 production rate during fasting might contribute to this useful metabolic adaptation; similar changes occur in hypothyroidism. Prolongation of the Achilles tendon reflex relaxation time in patients with anorexia nervosa, and of the pulse-wave arrival time during hypocaloric feeding are compatible with a hypothyroid-like state in undernutrition (Table 11C.1). In contrast, the illness-induced changes in glucose, protein, and fat metabolism are opposite to those that occur in hypothyroidism. Whether the occurrence of the low T3 syndrome in protracted critical illness can still be viewed as a beneficial adaptation to illness, remains doubtful.
Pathogenesis of Nonthyroidal Illness Syndrome
It has become clear that NTIS is a rather heterogeneous entity. The expression and pathophysiology of NTIS differs between starvation and illness, between acute and chronic stages of illness, and between mild and more severe illness (Fig. 11C.6). Animal models of NTIS have helped enormously to better understand the various pathophysiological mechanisms (for recent reviews see 44 and 45). Although animal models may not always reflect accurately what happens in humans, similarities in pathophysiology between animal and human NTIS are striking. Changes in the hypothalamic–pituitary–thyroid (HPT) axis and in peripheral thyroid hormone metabolism develop independent of each other (46). It follows that the factors mediating the various changes are operative simultaneously both centrally and peripherally.
Pathogenesis of Changes in the Hypothalamic–Pituitary–Thyroid Axis
Hypothalamic TRH and pituitary TSH secretion are under negative feedback control of serum thyroid hormones. The absence of a rise in serum TSH despite low serum T3 and T4 concentrations as observed in NTIS thus indicates an altered setpoint of the HPT axis. This is further suggested by the smaller than normal increase in serum TSH in response to small decreases in serum FT4 and FT3 induced by administration of inorganic iodide that occurs in patients with nonthyroidal illness (47).
Hypophysiotropic TRH neurons in the paraventricular nucleus (PVN) of the hypothalamus are one of the main determinants of the setpoint of the HPT axis (48). These neurons express MCT8, D3 (but not D2), and TR. The thyroid hormone transporters OATP1C1 (organic anion transporting polypeptide 1C1) and D2 are prominently expressed in astrocytes of
the median eminence and the infundibular nucleus (the human homologue of the arcuate nucleus in rodents, located outside the blood–brain barrier). They are also expressed in tanycytes that line the inferolateral borders and floor of the third ventricle. These cells are at the interface of the cerebrospinal fluid and the vascular system through long cytoplasmic projections that contact portal vessels and envelop hypothalamic blood vessels (49,50). It is thought that the prohormone T4 is taken up in these glial cells and converted via D2 into T3; T3 is then transported to TRH neurons in the PVN where it may bind to TR (thereby decreasing TRH) or may be degraded via D3 into T2 (thereby increasing TRH). Glial cell–derived T3 indeed is capable of activating neuronal gene expression by paracrine signaling (51).
the median eminence and the infundibular nucleus (the human homologue of the arcuate nucleus in rodents, located outside the blood–brain barrier). They are also expressed in tanycytes that line the inferolateral borders and floor of the third ventricle. These cells are at the interface of the cerebrospinal fluid and the vascular system through long cytoplasmic projections that contact portal vessels and envelop hypothalamic blood vessels (49,50). It is thought that the prohormone T4 is taken up in these glial cells and converted via D2 into T3; T3 is then transported to TRH neurons in the PVN where it may bind to TR (thereby decreasing TRH) or may be degraded via D3 into T2 (thereby increasing TRH). Glial cell–derived T3 indeed is capable of activating neuronal gene expression by paracrine signaling (51).
TRH secreting neurons in the PVN are also innervated by two anatomically distinct and functionally antagonistic populations of neurons from the arcuate nucleus: α-melanocyte-stimulating hormone (α-MSH) producing neurons that co-express cocaine and amphetamine-regulated transcript (CART), and neuropeptide Y (NPY) producing neurons that co-express agouti-related protein (AgRP). α-MSH stimulates but NPY and AgRP inhibit firing activity of TRH neurons in the PVN through a postsynaptic mechanism (52).
Hypophysiotropic TRH neurons project to the median eminence where mature TRH peptide is released into the portal capillary system and then binds to TRH receptors in pituitary thyrotrophs, influencing TSH production. Thyrotrophs are capable to further modulation of TSH secretion by converting T4 into T3 via D2.
Changes in HPT-axis During Fasting
The adipocyte-derived hormone leptin has been recognized as an important mediator of neuroendocrine changes during illness. Leptin communicates information about long-term energy stores. In starved animals, the fall in serum leptin is associated with a decrease of TRH mRNA in the PVN, and administration of leptin reverses the decrease in TRH mRNA (53). These effects are mediated via the arcuate nucleus, because ablation of the arcuate nucleus prevents the decrease in hypothalamic TRH and serum T4 during fasting and prevents the reversal of these changes by leptin (54). D2 activity in the mediobasal hypothalamus is upregulated during fasting, dependent on glucocorticoid levels: The D2 increase is prevented in adrenalectomized animals, and restored by glucocorticoid replacement but not by leptin or thyroid hormone administration (55). The release of T3 from tanycytes during fasting upregulates uncoupling protein 2 in arcuate nucleus neurons, leading to regulation of NPY (56). Leptin receptors are expressed on both αMSH/CART and NPY/AgRP neurons in the arcuate nucleus. It is thought that leptin prevents the inhibitory effect of NPY/AgRP but enhances the stimulatory effect of α-MSH on TRH gene expression in the PVN (57,58). Leptin also directly increases firing activity of TRH neurons in the PVN (52). The low leptin levels in starvation thus indirectly and directly may reduce TRH secretion by the PVN, altering the setpoint of the HPT-axis and explaining the fall in serum TSH.
In humans, however, administration of leptin only partially prevents the starvation-induced decrease in serum TSH, and does not prevent the fall in serum T3 and rise in rT3 (59). In subjects with low serum leptin (as in hypothalamic amenorrhea or weight-reduced state), leptin therapy is able to elevate FT4 and FT3 to pre-weight-loss levels (60,61).
Changes in HPT-axis During Illness
In animal models of NTIS, acute illness induced by bacterial lipopolysaccharide (62), chronic illness induced by turpentine injection in the hind limb (63), and prolonged critical illness due to burn injury (64) are all associated with an increased D2 activity in the mediobasal hypothalamus (notably in tanycytes), whereas D3 expression is decreased in the PVN (63,65). Together, these local changes in D2 and D3 (which occur independent of the fall in circulating thyroid hormones), will increase T3 content of TRH neurons in the PVN, explaining the observed decrease in TRH mRNA and in serum TSH. The explanation is in good agreement with human data showing a direct relationship between TRH mRNA in the PVN and serum TSH (Fig. 11C.5). The low or normal hypothalamic T3 content measured in humans (30) and animals (64) does not necessarily contradict this explanation as the PVN was not harvested selectively.
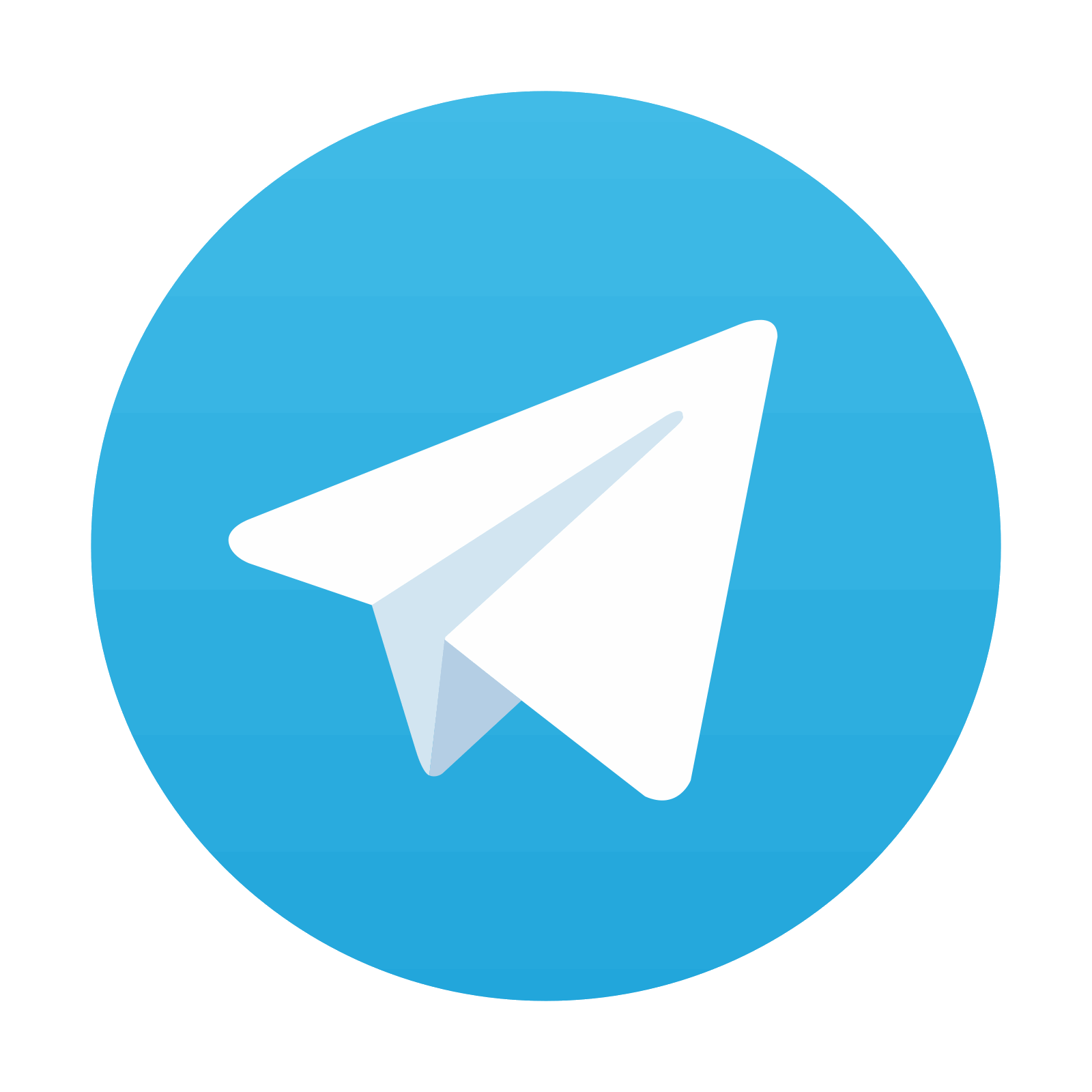
Stay updated, free articles. Join our Telegram channel
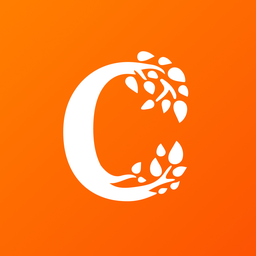
Full access? Get Clinical Tree
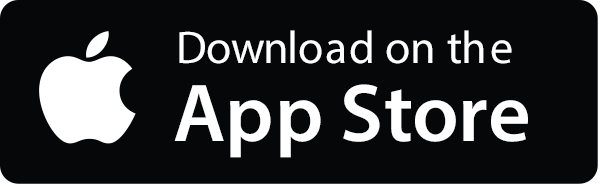
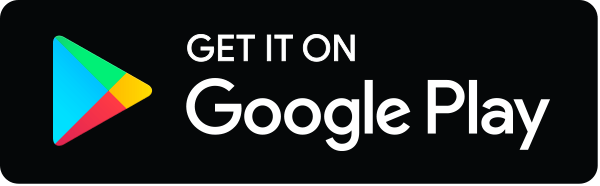