New Approaches for the Therapy of Bleeding Disorders, Including Gene Therapy
Gilbert C. White II
Harold R. Roberts
Better understanding of the molecular mechanisms of blood coagulation and the structure and function of blood clotting factors, along with advances in recombinant DNA technology have placed us on the threshold of remarkable new approaches to the treatment of bleeding disorders. Gene therapy is the most visible of these new approaches, but mechanisms now exist to actually make coagulation factors that are better than the native proteins. Some of these new approaches are the subject of this chapter.
HISTORICAL PERSPECTIVE
The treatment of bleeding disorders has traditionally been through the use of fresh frozen plasma or plasma-derived coagulation factor preparations. The first of these coagulation factor preparations was cryoprecipitate, which was developed by Judith Graham Pool in 1964. Cryoprecipitate was enriched in factor VIII, as well as fibrinogen, von Willebrand factor, and fibronectin, and for the first time provided a method of increasing factor VIII levels with less volume overload. Later came other coagulation factor preparations, including glycine-precipitated purified factor VIII and barium sulfate-purified prothrombin complex concentrates containing factors II, VII, IX, and X. These were produced commercially and revolutionized the treatment of hemophilia A and B, leading to home treatment. The prothrombin complex concentrates were also effective in the treatment of patients with coagulation factor inhibitors, allowing treatment of these individuals for the first time. The mechanism of action was uncertain, but activated clotting factors in the concentrates were thought to “bypass” the inhibitor block in the coagulation cascade. Although these crude plasma-derived concentrates changed the treatment of hemophilia, they contained blood-borne viruses that were transmitted to hemophiliacs. By the early and middle 1970s, hepatitis B and a new non-A, non-B form of hepatitis, later renamed hepatitis C, were becoming epidemic in the hemophilic population. Even worse, human immunodeficiency virus (HIV)/acquired immunodeficiency syndrome (AIDS) appeared in the hemophilic population in the early 1980s and eventually led to the untimely death of thousands of patients with hemophilia. In response to these blood-borne infections, the academic-industry complex developed physical and chemical methods for inactivating hepatitis, HIV, and other blood-borne viruses, used monoclonal antibodies to factors VIII and IX to generate antibody affinity-purified ultrapure forms of both factors, and developed recombinant DNA techniques for producing factors VIII and IX, resulting in synthetic forms of both factors that were essentially free of blood-borne contaminants. As a result of these and other advances, current products used to treat hemophilia are the safest ever developed.
Despite their safety, the current products have some drawbacks. They are expensive, require intravenous administration, and must be administered frequently because of the short biologic half-life of the normal coagulation factor proteins. For the average hemophiliac, who might bleed as many as 50 times a year or more, the cost of replacement therapy with current products can be as much as $50,000 per year. Prophylaxis, the treatment to prevent bleeding and the damage to joint and tissues, requires treatment two to three times a week or 100 to 150 times a year, with costs in excess of $50,000 and considerable damage to veins from frequent venipuncture. Developing technologies to make clotting factors that are cheaper, last longer after infusion into patients, or those that could be administered by alternative routes (including oral) would be advantageous. Gene therapy is another approach that has received considerable attention. This chapter reviews these new approaches to the treatment of bleeding disorders. The focus of the chapter is primarily on hemophilia A, but the concepts can be applied broadly to all bleeding disorders.
GENE THERAPY APPROACHES
Gene therapy is literally the use of genes, DNA or RNA, as treatment of a particular condition or disorder. In most cases, it is the proteins produced from the genes that have the intended effect in gene therapy, although DNA itself can have effects. For monogenic disorders like hemophilia or cystic fibrosis, the intent of gene therapy is curative. The aim is to use normal genes to express enough normal protein to correct the abnormality. One of the great promises of gene therapy is the potential ability to cure monogenic disorders such as hemophilia. For complex multigenic disorders, such as atherosclerosis, diabetes, or cancer, the aim of gene therapy is modification of the disease process. The goal might be to express a protein or gene that is toxic to a cancer cell or inhibits smooth muscle proliferation in atherosclerosis.
The origins of gene therapy lie in the remarkable advances in recombinant DNA technology that occurred in the middle and latter half of the last century. The ability to isolate and manipulate DNA, and the development and use of viral and nonviral plasmid vectors to introduce DNA into cells where the DNA products could be expressed were critical developments. In one
of the early reports using direct injection of DNA into cell nuclei, Jaenisch and Mintz reported injecting SV40 DNA into mouse blastocysts and finding SV40 gene sequences developing from the blastocysts in the animals.1 The notion of using viruses as vectors to introduce new genetic information into cells evolved very quickly. By 1979, several laboratories were employing the SV40 virus, using viral promoter, splicing, and polyadenylation sequences in plasmid vectors to introduce β-globin sequences into cells and drive transgene expression.2,3,4 Retroviruses, which are adept at gaining entry into cells and inserting their genetic material into the host genome, soon followed. Defective retroviruses in which the malignant transforming gene region was eliminated, were shown to retain the ability to transduce cells.5,6,7 The first gene transfer experiments in humans were carried out by Cline in 1980 in two subjects with severe β thalassemia.8 Nucleated cells, obtained by bone marrow aspiration, were transfected in vitro using a pBR vector containing a herpes simplex virus thymidine kinase (HSVtk) marker gene, and then returned to the subject intravenously. HSVtk sequences were detected at low levels 1 to 2 weeks after transfection and lasted 3 months in one subject and 9 months in the second.
of the early reports using direct injection of DNA into cell nuclei, Jaenisch and Mintz reported injecting SV40 DNA into mouse blastocysts and finding SV40 gene sequences developing from the blastocysts in the animals.1 The notion of using viruses as vectors to introduce new genetic information into cells evolved very quickly. By 1979, several laboratories were employing the SV40 virus, using viral promoter, splicing, and polyadenylation sequences in plasmid vectors to introduce β-globin sequences into cells and drive transgene expression.2,3,4 Retroviruses, which are adept at gaining entry into cells and inserting their genetic material into the host genome, soon followed. Defective retroviruses in which the malignant transforming gene region was eliminated, were shown to retain the ability to transduce cells.5,6,7 The first gene transfer experiments in humans were carried out by Cline in 1980 in two subjects with severe β thalassemia.8 Nucleated cells, obtained by bone marrow aspiration, were transfected in vitro using a pBR vector containing a herpes simplex virus thymidine kinase (HSVtk) marker gene, and then returned to the subject intravenously. HSVtk sequences were detected at low levels 1 to 2 weeks after transfection and lasted 3 months in one subject and 9 months in the second.
Hemophilia A is a monogenic disorder which, like sickle cell disease, cystic fibrosis, and muscular dystrophy, has historically been considered as an attractive target for treatment using gene transfer approaches. Primary prophylaxis studies in which clotting factor concentrates have been administered to maintain low levels of factor VIII have shown that near-continuous correction of the clotting factor defect prevents bleeding in unaffected joints and delays progression in diseased joints.9,10,11 Logically, more continuous levels of factor VIII, as might be anticipated with gene therapy, provide the rationale for the enthusiasm for this approach in hemophilia A.
Other aspects of hemophilia A add to its attractiveness as a candidate disorder for gene transfer (see Table 79.1). As a monogenic disorder, correction of the factor VIII defect should correct the phenotype. Furthermore, unlike monogenic inborn errors of metabolism, levels of factor VIII are not regulated in any way and do not require feedback or other control as, for example, insulin requires regulation by blood glucose levels. Another important feature is that complete correction of the factor VIII level is not absolutely necessary in hemophilia for gene transfer to be effective. For example, an increase in plasma levels of factor VIII by even as little as 1% to 2% could have a beneficial effect, especially in patients with severe hemophilia. Persistence of expression after gene transfer might also have beneficial effects. Given the biological half-life for current plasma products of approximately 12 hours, expression of factor VIII for even a month would change patients’ lives. Factor VIII message is detected in a number of tissues,12 but as long as the factor VIII that is expressed after gene transfer is processed correctly and has access to blood, it does not matter where the protein is produced. There is no current evidence that synthesis of factor VIII in tissues outside its site of normal synthesis is detrimental to that tissue. Therefore, it is not necessary to target the factor VIII to a particular tissue or to its tissue of natural synthesis. In addition, factor VIII is a secreted protein that would be expected to gain easy access to blood. The gene for factor VIII is well characterized and, based on homology modeling, much is known about the structure of the factor VIII protein and the function of the various structural domains.13 A low-resolution crystal structure of factor VIII is available.14 Good clinical and laboratory parameters of efficacy are available. Large and small animal models of hemophilia are available for preclinical trials.15,16 Finally, safe and effective treatments for hemophilia currently exist, and so subjects can make appropriate decisions about participation in clinical trials without the pressure engendered by the lack of adequate treatment.
Table 79.1 Hemophilia as a target for gene transfer | ||||||
---|---|---|---|---|---|---|
|
Gene Transfer Vectors*
Viral Vectors
Viruses have evolved highly efficient mechanisms for entering human cells and using the DNA/RNA replication machinery of the cell to produce viral products.17 By replacing viral coding sequences with genes of particular interest (e.g., the cDNA for factor VIII) one can use viruses as agents to introduce the gene into cells where they use the cellular machinery to produce therapeutic quantities of the protein. The obvious advantage of viruses as vectors is their native ability to get into cells; the general disadvantage is their toxicity.
Retrovirus Vectors
Retroviruses are enveloped RNA viruses that use reverse transcriptase to convert virion RNA into double-stranded DNA; the DNA is subsequently integrated into the genome of the cell. The surface of the retrovirus consists of a lipid bilayer with protruding membrane proteins, GP41 and GP120, both encoded by the env gene. The tropism of the virus, that is the host cell that is targeted by the virus, is determined by these surface proteins. For example, for HIV-1, GP120 mediates the interaction of HIV-1 with CD4 lymphocytes. For gene therapy vectors, it is common to replace the retroviral env gene with other sequences that change the tropism of the vector. This can be done to either target a particular tissue or cell or to broaden the tropism of the vector to increase the number of cells that are transduced. Two sequences that are frequently used to broaden the tropism of retrovirus vectors are the amphotropic envelope gene of
murine leukemia viruses, which is able to interact with a widely expressed phosphate transporter on human cells, and the glycoprotein of vesicular stomatitis virus, which interacts with a ubiquitous receptor on cells. Current retroviral vector production is through a three-part packaging system, consisting of a genome vector that contains the packaging signal and the cDNA of interest, an expression vector that contains the pseudotyped envelope glycoprotein, and an expression vector that expresses the viral gag and pol genes. These are assembled into vector particles in a packaging cell. The retroviral packaging systems in current use appear safe and are designed to prevent the generation of replication-competent virus. In addition, sufficient modifications are made in the viral sequence to minimize the possibility of homologous recombination with retroviral sequences in target cells. Self-inactivating (“sin”) vectors are ones in which the long-terminal repeats (LTRs) have been removed to prevent activation of downstream cellular genes.
murine leukemia viruses, which is able to interact with a widely expressed phosphate transporter on human cells, and the glycoprotein of vesicular stomatitis virus, which interacts with a ubiquitous receptor on cells. Current retroviral vector production is through a three-part packaging system, consisting of a genome vector that contains the packaging signal and the cDNA of interest, an expression vector that contains the pseudotyped envelope glycoprotein, and an expression vector that expresses the viral gag and pol genes. These are assembled into vector particles in a packaging cell. The retroviral packaging systems in current use appear safe and are designed to prevent the generation of replication-competent virus. In addition, sufficient modifications are made in the viral sequence to minimize the possibility of homologous recombination with retroviral sequences in target cells. Self-inactivating (“sin”) vectors are ones in which the long-terminal repeats (LTRs) have been removed to prevent activation of downstream cellular genes.
Vectors derived from the Moloney murine leukemia virus (MoMLV) were the first retroviral vectors used for gene transfer. MoMLV has a 9-kb cDNA expression cassette and is therefore able to accept the entire 7.1-kb factor VIII cDNA or 1.9-kb factor IX cDNA. Although MoMLV can be produced in large quantities and is able to efficiently gain entry into cells, it only transduces dividing cells,18 a disadvantage for many tissues such as liver where the rate of cell replication is low. Strategies for inducing cell division in the liver using partial hepatectomy,19,20 chemical injury,21 or hepatocyte growth factor22,23,24,25 have been proposed to increase retrovirus-mediated transduction.
More recently, vectors derived from lentiviruses, including HIV-1, have been developed.26,27 Members of this family of retroviruses include feline immunodeficiency virus, simian immunodeficiency virus, and equine infectious anemia virus.28,29,30 Like MoMLV, lentiviral vectors have a 9-kb cDNA expression cassette. These vectors retain some of the attractive features of the Moloney-based retroviral vectors, such as stable integration into the host chromosome and targeted cellular uptake through coat proteins, and they are able to infect nondividing and dividing cells.26,31 More recent observations provide greater understanding of the mechanisms of nuclear import by lentiviruses and may lead to improvements in cell transduction. During reverse transcription (RT) of HIV-1, a three-stranded DNA flap is formed that is composed of the central polypurine tract from the pol gene and central termination sequences. This flap functions as a cis-acting element to enhance nuclear import and improve gene transduction in nondividing cells.32,33,34,35 Other cis-acting DNA elements that enhance transgene expression, perhaps through a mechanism like the DNA flap, have been identified, including the matrix attachment region from immunoglobulin κ36 or from β interferon.37,38
Although retroviral integration is not sequence-specific, there is increasing evidence that it is also not completely random, as was once thought. The ability of retroviruses to successfully replicate themselves depends on integration of the viral DNA into transcriptionally active areas of the host cell chromosome. Integration into silent areas would prevent expression of viral proteins and inhibit replication. Studies to map the site of retrovirus integration demonstrate that different viruses have different patterns of integration.39,40,41 With murine leukemia viruses (MLV), for example, transcriptional start sites are favored targets. For HIV-1, active genes are preferential integration targets, especially genes that are activated by infection of the cell by the retrovirus. Regional hotspots also exist.39 Whether the predilection for active genes results from increased chromatin accessibility, locally bound transcription factors, or some other influence is not clear. In contrast, for avian sarcoma leukosis virus, an avian retrovirus, the virus does not prefer transcription start sites or active genes,41 a feature that might confer an advantage with regard to gene therapy and the risk of insertional mutagenesis (see below).
Retroviruses insert their DNA into the host chromosome and are therefore termed integrating vectors. Because of their integrating properties, insertional mutagenesis is a concern with some retroviruses. Integration of retroviral sequences near protooncogenes has been known to be capable of activating their expression, contributing to tumorigenesis in animal models.42,43 A report of T-cell leukemia in subjects with X-linked severe combined immunodeficiency syndrome treated by MLV-mediated gene transfer44 shows that insertional mutagenesis occurs in man. The subjects were among 10 receiving ex vivo retrovirus-mediated gene transfer into autologous CD34+ bone marrow cells. Approximately 3 years after treatment, the subjects developed acute T-cell lymphocytic leukemia. The leukemic T-cells showed evidence for retroviral insertion near the LMO2 proto-oncogene promoter. Because of the survival advantage that the cells expressing the retroviral γC-transgene had over the normal T-cells, it has been suggested that the insertional mutation in the LMO2 promoter region resulted in uncontrolled clonal expansion of the corrected T-cells, initially correcting the severe combined immunodeficiency and then resulting in T-cell leukemia.
Adenovirus Vectors
Adenoviruses (Ads) are linear, double-stranded DNA viruses that infect a wide variety of human cells, both dividing and nondividing, including lung, liver, heart, and brain. The 36-kb viral genome consists of a number of early and late genes. Transcription and translation of the early genes modulates cell function to facilitate replication of the viral DNA, whereas the late genes are involved in the structural aspects of viral replication. The icosahedral adenoviral capsid is composed of three major components: the hexon, penton base, and knobbed fiber. Viral entry into cells is accomplished through an initial high-affinity interaction between the knobbed fiber45,46 and a widely expressed, 46-kDa coxsackie-adenovirus receptor (CAR) on the surface of cells.47 After the initial interaction with CAR, entry of the virus into the cell proceeds through clathrin-mediated endocytosis, by an interaction between the penton base and αVβ3 and αVβ5 integrins on the cell surface. Disruption of the viral capsid occurs in the endocytic vesicle and the viral genome enters the nucleus through nuclear pores. In the nucleus, the adenoviral genome does not integrate into the chromosomes of the host cell, functioning instead from an extrachromosomal or episomal template.
Adenoviral vectors characteristically elicit an intense inflammatory response. This is due in part to capsid proteins, which provoke a brisk humoral and cellular immune response.48 At the same time, Ad is designed to evade the immune response that it elicits.49,50 Products of the E1A gene interfere with nuclear factor κB (NFκB) which is a key regulator of the innate antiviral response by cells. Products of the E3 gene are also known to subvert the host immune response. Other gene products play a role, as well. One of the goals in the generation of adenoviral vectors
is to retain the high degree of efficiency that these vectors have with respect to cell infection while moderating or eliminating the inflammation that they cause. First-generation adenoviral vectors were deleted in E1 and E3, removing some of the primary mediators of the immune response while increasing the expression cassette to 6.5 kb. The E2 genes were removed in the second-generation vectors and, in recent vectors, all of the viral coding sequences have been removed, rendering the vector “gutless.”51,52 Although preclinical studies indicate that removing these proteins may reduce the cellular immune response,53,54,55 there is also evidence that the virion shell alone elicits substantial cellular responses.56
is to retain the high degree of efficiency that these vectors have with respect to cell infection while moderating or eliminating the inflammation that they cause. First-generation adenoviral vectors were deleted in E1 and E3, removing some of the primary mediators of the immune response while increasing the expression cassette to 6.5 kb. The E2 genes were removed in the second-generation vectors and, in recent vectors, all of the viral coding sequences have been removed, rendering the vector “gutless.”51,52 Although preclinical studies indicate that removing these proteins may reduce the cellular immune response,53,54,55 there is also evidence that the virion shell alone elicits substantial cellular responses.56
There are at least 47 different adenoviral serotypes, each immunologically distinct. The development of an immune response to one vector serotype generally precludes readministration of that vector. Adenoviral vectors for gene transfer are primarily Ad2 or Ad5.
The only death that was directly attributable to a gene transfer trial occurred with an adenoviral vector in an 18-year-old subject with ornithine transcarbamylase deficiency.57 The second-generation vector used in this study was based on human Ad5 and was deleted in E1 and E4. Following administration of the vector in the right hepatic artery, altered mental status and jaundice were noted 18 hours later, and the ensuing clinical course was marked by a systemic inflammatory response syndrome with high levels of interleukin-6 (IL-6) and IL-10, biochemically detectable disseminated intravascular coagulation, and multiple organ system failure, leading to death 98 hours following gene transfer. The story of Jesse Gelsinger has been well-chronicled in the news media.58
Adenovirus-Associated Virus Vectors
Adenovirus-associated viruses (AAV) are small, nonenveloped, single-stranded DNA viruses, which require helper virus to facilitate efficient replication. In the absence of helper virus-mediated replication, the wild-type virus persists in the host cell in a latent state. The 4.7-kb genome of AAV consists of two inverted terminal repeats (ITRs) and two open reading frames that code for the rep and cap proteins. There are four rep proteins that function in regulating AAV replication and three cap proteins that form the protein coat of the virus. Wild-type AAV stably integrates into the host genome at the AAVS1 region of chromosome 19q13.3-qter.59 Two of the rep gene products, rep68 and rep78, are believed to direct integration to the AAVS1 site.60 Nine different AAV serotypes have been identified, AAV1 to 9, each with distinct tissue tropism.61 AAV8 targets primarily liver, whereas AAV1 and AAV7 transduce muscle with high efficiency. The cellular receptor for AAV2, the serotype most commonly used for gene transfer studies, is heparan sulfate, a glycosaminoglycan that is variably present on the surface of most cells.62 Slow-twitch (slow myosin-expressing) skeletal muscle fibers have higher concentrations of heparan sulfate proteoglycan on their surface and are better transduced by AAV2 than fast-twitch fibers.63 Interestingly, AAVS1, the site of integration, is closely linked to the slow skeletal troponin T gene, TNNT1,64 raising an interesting correlate with the preference of AAV for slow myofibers. The integrin αVβ5 and fibroblast growth factor receptor 1 are coreceptors for AAV2.65,66
Typical AAV gene transfer vectors have been derived from AAV2. The ITRs are retained but the rest (96%) of the viral genome has been removed, including the rep gene. As a result, AAV vectors currently in general use are unable to integrate into the host chromosome in a site-specific manner, and persistence as integrated sequences appears to be a low-frequency event, if it occurs at all. Efforts to quantify in vivo integration in skeletal muscle and liver suggest that at least 99.5% (skeletal muscle) or at least 90% (liver) of persisting AAV vector DNA was episomal, present in large concatemers.67,68
The AAV expression cassette is approximately 5 kb, too small to accommodate the whole factor VIII cDNA but large enough to accommodate the factor IX cDNA. Several groups have separately packaged factor VIII light and heavy chain sequences under the control of minimal transcriptional regulatory elements into AAV vectors and demonstrated expression in vivo after coinfection.69,70,71,72
AAV are not associated with any known disease and are not pathogenic.73 On the basis of this nonpathogenicity, the toxicity associated with AAV has been expected to be low. In preclinical studies, AAV has been well tolerated with no effects on hematopoiesis, liver function, or other organs. Reports of late tumors in mice treated with AAV vectors74 have not been confirmed and the chromosomal breaks that have been observed in cells following AAV integration75 are of uncertain significance, especially because integration by AAV vectors lacking the rep gene is infrequent. However, in liver-directed human trials for hemophilia B, transient liver enzyme abnormalities have been reported and were associated with loss of transgene expression.76
Recent advances in AAV technology include the production of self-complementing (sc) forms of the vector that overcome the rate-limiting complementary-strand DNA synthesis step that is the primary barrier to transduction in vivo and lead to faster onset of protein expression and higher transduction efficiency.77,78 This is accomplished through deletion of the terminal resolution site from one of the AAV terminal repeats (TRs) thereby enabling, after entry into the cell and uncoating, intramolecular base pairing of the viral DNA within the mutant TR that then proceeds to formation of a double-stranded molecule. The disadvantage of scAAV is the reduced insert size. A second advance has been the continued evolution of the identification of naturally occurring AAV serotypes and the idea of crosspackaging vector genomes from one AAV serotype into the capsid proteins of other AAV serotypes, generating “pseudotyped” vectors. This pseudotyping permits novel targeting strategies and can be used to reduce immune neutralization of vector.79,80,81
Plasmid Vectors
Nonviral approaches for delivering therapeutic genes as naked plasmid DNA are attractive because plasmid DNA is relatively simple and inexpensive to produce, does not engender cell-mediated immune responses, and does not result in humoral immune responses against the DNA vehicle that would limit the opportunity for repeated delivery. Until recently, most plasmid DNA applications have resulted in short-lived (days to weeks) transgene expression. Recent attempts to use electrical82 or hydrodynamic83 approaches to augment target cell uptake of plasmid DNA have resulted in expression of clotting factors lasting months after delivery. By rapidly treating mice through the tail vein with factor VIII expression sequences under the control of transcriptional regulatory elements optimized for liver expression, in large fluid volumes, supraphysiologic factor VIII levels were obtained.83 Immune responses that are species independent have been reported.84 Despite the attractive features of
nonviral approaches, current technical limitations reduce the likelihood of human use in the near future.
nonviral approaches, current technical limitations reduce the likelihood of human use in the near future.
Spliceosome-Mediated Pre-mRNA Transsplicing
A specific repair mechanism that may be especially applicable to the common inversion mutations that cause approximately 30% of hemophilia A is an approach termed spliceosome-mediated mRNA transsplicing.85 This method is used to repair messenger RNA, rather than DNA. In the final steps of transcription, introns are removed and exons are spliced together to form mature, functional mRNA. The site of splicing is directed by 30 to 40 nucleotides at each end of the intron that form consensus binding sites for the spliceosome, a large ribonucleoprotein complex composed of small nuclear ribonucleoprotein particles that catalyze the splicing reaction. Spliceosome-mediated pre-mRNA transsplicing takes advantage of this normal cellular process to effect RNA repair. The method involves synthesis of a pretranssplicing molecule (PTM) that contains the corrected mRNA fused to a 30 to 40 nucleotide sequence that targets a specific pre-mRNA. The PTM binds to the pre-mRNA, allowing specific transsplicing of the corrected mRNA through normal mechanisms.
Chao et al.86 used a transsplicing approach to correct the hemophilic defect in factor VIII knockout mice. The repair PTM consisted of a 125-nucleotide binding domain that was complementary to intron 15 of the gene, a spacer sequence, and a strong 3′ splice acceptor site, linked to exons 16 to 26 for the mouse factor VIII cDNA. Correctly transspliced factor VIII was detectable genetically and functionally in the treated mice, with up to 17% factor VIII activity observed. It was estimated that conservatively between 1.6% and 6.3% of mutant transcripts were being repaired.
Enhanced Translational “Read Through”
In prokaryotes and eukaryotes, aminoglycoside antibiotics cause ribosomes to read through stop codons during translation.87,88 As a result, these antibiotics can suppress nonsense genotypes, increasing expression of the full-length protein and partially restoring the normal phenotype. In tissue culture cells, restoration of gene expression to as much as 20% of wild-type levels has been observed after aminoglycoside treatment.87 Clinical trials of gentamicin in cystic fibrosis showed that translational “read through” could be obtained in patients who were both homozygous and heterozygous for stop mutations with a reduction in basal potential difference in nasal mucosa and an increase in response to chloride-free isoproterenol solution.89 Approximately 9% of hemophilia A and hemophilia B mutations are nonsense mutations that might respond to aminoglycoside treatment. Several small preliminary studies have examined the responses to aminoglycoside antibiotics in patients with hemophilia A and B and nonsense mutations with inconsistent results.90,91,†
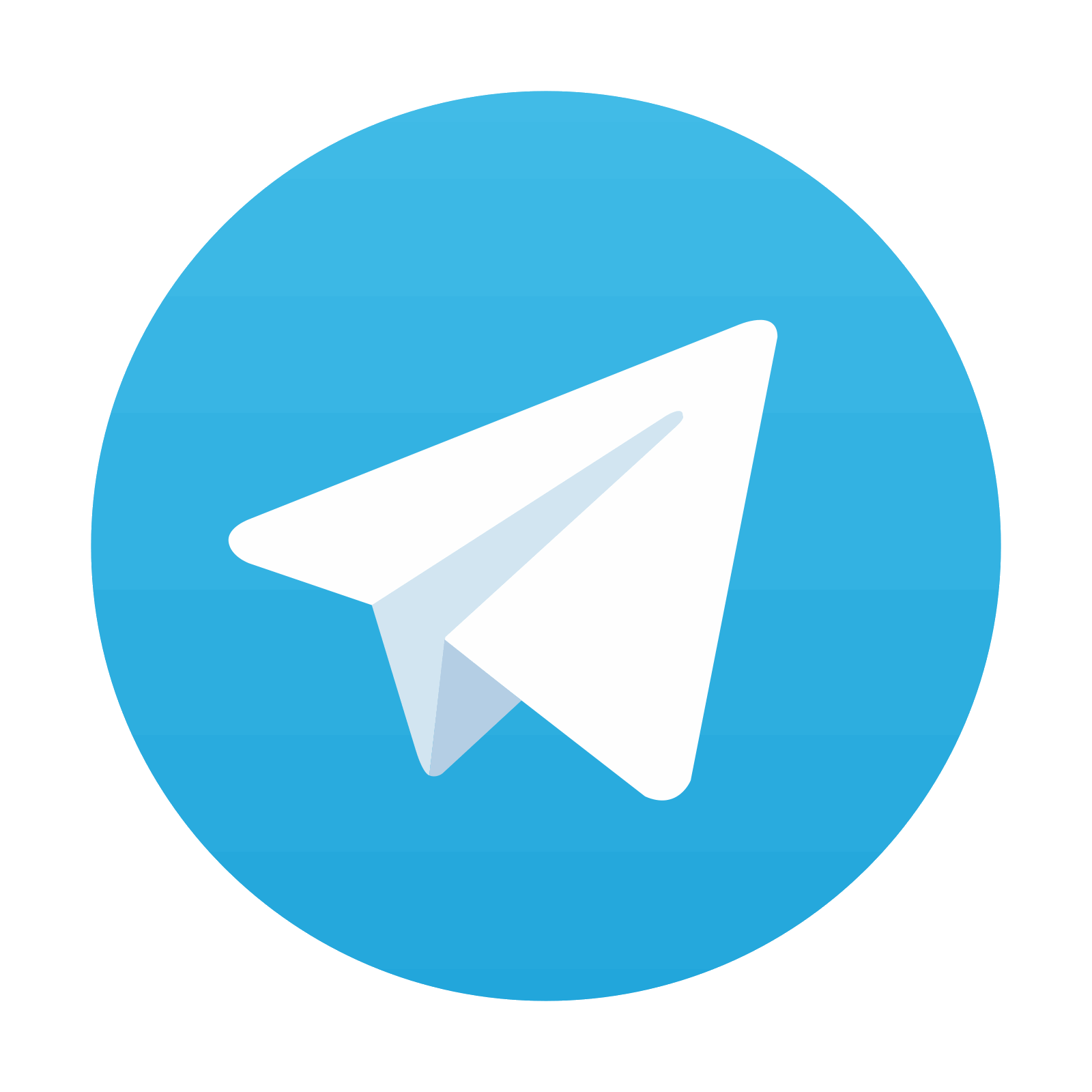
Stay updated, free articles. Join our Telegram channel
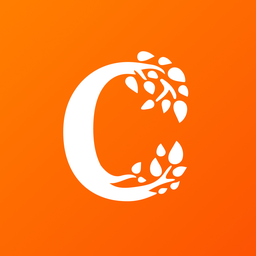
Full access? Get Clinical Tree
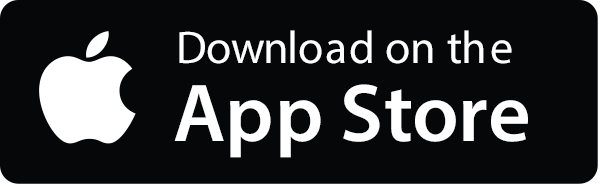
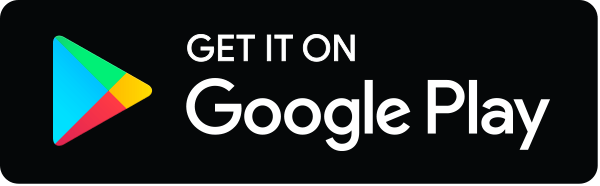
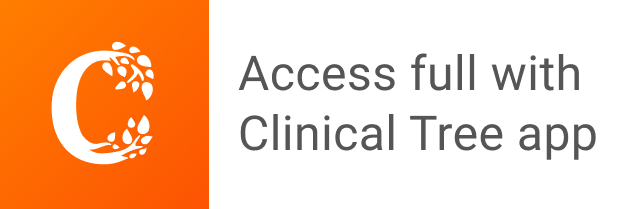