Fig. 1
Schematic of some of the mechanisms of endocytosis that have so far been elucidated. The focus in this chapter will be on clathrin-mediated, caveolar-type, macropinocytosis, and phagocytosis mechanisms. Adapted from [68] with permission of The Royal Society of Chemistry
2.1 Clathrin-Mediated Endocytosis Is Normally Involved in Receptor-Mediated Uptake by Cancer Cells
The most well-known and studied uptake mechanism is clathrin-mediated endocytosis. This mechanism is responsible for the endocytosis of iron-saturated transferrin, low-density lipoproteins, the influenza virus and many types of nanoparticles in a variety of cell types including cancer cells [12]. It is also presumed to be responsible for the uptake of nanoparticles when their surface is coated with certain targeting ligands [13]. The initiation of clathrin-mediated endocytosis is hypothesized to be spontaneous, and therefore non-specific, beginning with the assembly of FCHO and AP2 (and several other proteins) on the cell membrane [14, 15]. The binding of a molecule or nanoparticle onto multiple membrane receptors can also trigger the process in a ligand specific manner by creating membrane curvature, which initiates the assembly of AP2 [14]. Following initiation, a clathrin coat forms underneath the cell membrane, and the membrane is pulled into the cell to form a pit [14, 15]. The pit and the clathrin coat can be visualized unambiguously using TEM [16]. At this point, the involvement of a cargo on a receptor is required for maturation of the pit into an endocytic vesicle [17]. Once the clathrin assembly matures, dynamin catalyzes membrane scission and separates the newly formed vesicle from the cell surface [17]. Clathrin vesicles have a minimal diameter of 31 nm as determined by the cryo-TEM of vesicles, but the upper limit may be much higher [18]. Tsuji et al. confirmed the clathrin-mediated uptake of 523 nm diameter particles by directly visualizing the clathrin coat using TEM [16]. Veiga et al. and others have reported the clathrin-dependent uptake of particles as large as 1 μm in diameter through fluorescence co-localization and siRNA knockdown techniques [16, 19].
2.2 Clathrin-Independent Endocytic Mechanisms Are Prevalent in Many Cell Types, but Are Numerous and More Difficult to Study
Nanoparticle uptake through clathrin-independent endocytosis pathways has been observed [20]. However, multiple pathways are involved and their characteristics are not completely understood because it is difficult to distinguish between them. [21]. The most commonly cited clathrin-independent pathway is caveolin-mediated uptake. Both spherical nucleic acids-gold nanoparticles and polyethylenimine—DNA complexes have demonstrated cellular uptake through this pathway [11, 22]. Caveolin is a membrane protein that is enriched in flask-shaped invaginations on the cell membrane. It was assumed that enclosed intracellular structures positive for caveolin1 as observed by TEM were endocytic vessels formed in caveolae; however, Sandvig et al. have recently argued that these structures are an artifact of sectioning and do not get internalized [21]. Nevertheless, caveolin1 is strongly associated with endocytosis perhaps through another pathway [11, 21, 23].
Unfortunately, our understanding of other clathrin-independent mechanisms is even more limited [21]. It is often difficult to distinguish these mechanisms from each other and from caveolin-mediated endocytosis [24]. One way to distinguish them is to determine their dependence on dynamin (which can be inhibited by dynasore and other agents), cholesterol (which is inhibited by methyl beta cyclodextrin and others) and other molecules essential to endocytosis. Inhibition of these pathways by small molecule inhibitors is simple to implement, but suffers from drawbacks due to the lack of their specificity for particular endocytic pathways [25]. An alternative approach is to measure the co-localization of nanoparticles with labeled proteins specific to each endocytic pathway using confocal microscopy [19]. This procedure is more expensive and time-consuming to implement. As a result, it is not commonly used for studying nanoparticle-cellular interactions.
2.3 Larger Particles Are Normally Taken up by Micropinocytosis in Most Cancer Cells
Although the association of defined particle sizes with specific uptake mechanisms has not been determined and is under heavy investigation, macropinocytosis is presumed to be responsible for the uptake of particles larger than 200 nm diameter in many cases. Unlike the mechanisms discussed earlier, the uptake of large volumes is usually actin-dependent since large changes to the cell membrane are required in order to engulf such particles. Macropinocytosis proceeds through the formation of extensive membrane ruffles, which non-specifically engulf nanoparticles and other entities [26]. This process requires both actin and cholesterol [12] and is distinctly observed using electron microscopy. It was shown by Herd et al. to be involved (along with phagocytosis) in the uptake of large silica particles (>200 nm diameter) and also by Iverson et al. to be involved in the uptake of ricinB-coated quantum dots (diameter of only 20 nm). These studies highlight the lack of consensus in establishing strict size limits for endocytic mechanisms [27]. It is likely that size is only one factor in determining the uptake mechanism of nanoparticles and the impact of other properties is still being evaluated.
2.4 Off-Target Accumulation of Nanoparticles Is Presumed to Be Due to Phagocytosis
Phagocytosis is thought to be responsible for the receptor-mediated uptake of particles larger than 500 nm in diameter, although strict size limits continue to be debated. Physiologically, this mechanism is used predominantly by professional phagocytes, which include monocytes, macrophages, neutrophils, dendritic cells, and mast cells. These cells are responsible for the clearance of most types of nanoparticles [28]. This mechanism is strictly receptor-mediated, but can be activated by opsonins bound to the nanoparticle surface that cause the phagocytes to engulf diverse cargo. The normal mechanism is initiated by the clustering of receptors induced by the binding of cargo to the cell surface. Endocytosis then proceeds by engulfment mediated by the assembly of an actin skeleton [12]. Since phagocytosis can operate on micron-sized particles, this process can be observed by light microscopy on live cells. Visualizing cell and particle interaction directly, Champion et al. showed that phagocytosis is sensitive to the curvature of the particle at the point of first contact and that beyond a contact angle of 45°, it is completely inhibited [8]. Phagocytosis can also be prevented by mimicking the molecules present on normal cells. Since professional phagocytes avoid ingesting healthy cells by recognizing molecular markers on their surface, such as CD47, Rodriguez et al. synthesized a peptide to mimic this marker. This “self peptide”, when functionalized on polystyrene nanoparticles improved their blood circulation half-life two-fold compared to nanoparticles functionalized with scrambled peptides (30 min compared to 15 min) [29]. Strategies that reduce the phagocytic uptake of nanoparticles may be key to reducing off-target accumulation of nanoparticles.
3 Contact with Blood/Serum
The rapid adsorption of proteins on the surface of nanoparticles occurs immediately following their exposure to biological media [30]. This layer is referred to as the protein corona and it can change the size, shape, surface characteristics, aggregation state, and subsequent biological behaviors of the nanoparticles [31–33]. It is important to understand the binding and activation of opsonins as these steps are required for the receptor-mediated phagocytosis of nanoparticles by professional phagocytes as discussed earlier. This binding and activation affects nanoparticle fate in vivo. The influence of these proteins on mechanisms of off-target and on-target uptake is explored in this section.
3.1 Adsorption of Serum Proteins Changes Fundamental Nanoparticle Properties
Protein adsorption changes a broad range of fundamental nanoparticle properties. For example, protein adsorption reduced the cytotoxicity of carbon nanotubes and protected PEGylated quantum dots from the fluorescence quenching that normally occurs in physiological buffers [34, 35]. Typically, the formation of the protein corona increases the hydrodynamic diameter of nanoparticles by 3–35 nm and may cause their aggregation into larger clusters (as shown in Fig. 2) [33, 36, 37]. In addition, they often display a slight negative charge (zeta potential between −7 and −25 mV), regardless of their original charge prior to their interaction with serum [30]. Since the size, shape, and charge of nanoparticles are known to affect the in vitro behavior of nanoparticles, the changes to these properties can drastically change observed uptake patterns.
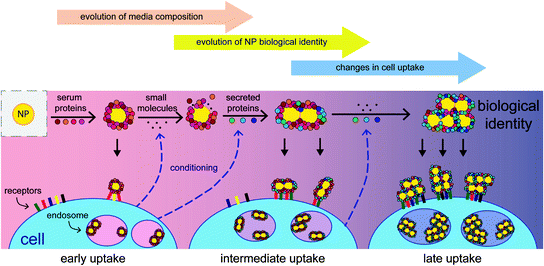
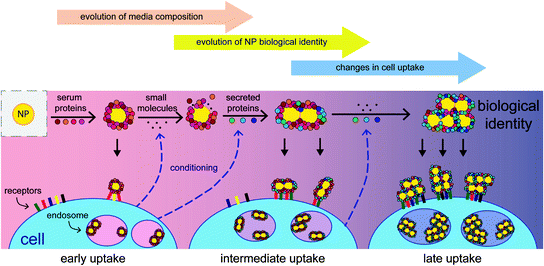
Fig. 2
Since the adsorption of serum proteins occurs on the nanoparticle surface, all subsequent surface-mediated interactions can be affected by the presence of these proteins. Reprinted with permission from [36]. Copyright 2014 American Chemical Society
3.2 Serum Protein Adsorption on Nanoparticle Surface Redirects Their Fate In Vitro
The clearest observed consequence of serum protein adsorption is the decreased uptake of highly charged particles that is observed after exposure to serum or other proteins [32, 33]. Cationic nanoparticles have been observed to have higher uptake than anionic particles in general, and this effect is retained (although less prominent) after their exposure to serum, even if both particle types have the same zeta potentials. This suggests that the difference in uptake is mediated by the proteins present on the surfaces of the nanoparticles [30]. Additionally, nanoparticles with targeting ligands on their surface may lose their binding specificity when exposed to serum, presumably due to competing mechanisms of uptake mediated by these serum proteins [38]. In fact, so many properties are modified by serum protein adsorption that the protein corona has been shown to be more predictive of the observed cell uptake of nanoparticles than any other characteristic of the construct [32].
3.3 The Mechanisms Employed by Adsorbed Proteins to Bind to the NP Surface and Redirect Their Fate Are not Fully Understood
The presumed mechanism of off-target accumulation of nanoparticles is the coating of their surface with opsonins, which mediate the uptake of these nanoparticles by professional phagocytes. Over 100 different proteins have been identified that adsorb onto nanoparticles following serum exposure, including well-known opsonins such as complement factors and immunoglobulins [39]. The mechanism employed by these proteins to bind to the nanoparticle surface is not well-understood except that charged and hydrophobic particles seem to accumulate more proteins than neutral hydrophilic particles, particularly those coated with dense layers of polyethylene glycol (PEG) [32]. The subtle changes in the nanoparticle surface chemistry (varying types of anionic functional groups) dramatically change the proteins that adsorb on their surface implying that these mechanisms may be quite complex [32]. For example, the protein adsorption on DNA-coated gold nanoparticles depends on the sequence of DNA displayed on the surface and its secondary structure [40]. Given, this apparent selectivity and complexity, we need to understand which proteins are involved in this process and how their binding can be modulated to provide greater control of the interactions of nanoparticles with cells after serum exposure.
3.4 Surface Passivation Is the Predominant Strategy to Reduce the Influence of Serum Protein Adsorption
Since the mechanisms of serum protein-mediated nanoparticle-cell interactions are not well-understood, the predominant strategy for reducing these undesirable outcomes has been to passivate the surface of nanoparticles with polyethylene glycol (PEG) moieties [41]. Certain zwitterionic surface ligands are also purported to reduce the binding of serum proteins, but more detailed experiments are required to determine how this strategy compares to PEGylation [42]. Surface passivation reduces both macrophage uptake and increases blood circulation half-life for a variety of nanoparticle types [43–45]. In addition, the loss of specificity due to serum protein adsorption and nanoparticle aggregation is also drastically reduced with appropriate passivation of the surface with PEG [37, 46]. However, proteins continue to adsorb on the nanoparticle surface even when the nanoparticle surface is decorated with a very dense layer of PEG [43]. Further, these proteins significantly affect the cell uptake of these particles [32]. Other limitations of PEG include the difficulty of obtaining a high density of PEG on many particle types as well as the fact that PEG increases the hydrodynamic size of nanoparticles which may make the nanoparticle too large for some applications. Alternatives to PEG, including the zwitterionic ligands discussed above, continue to be investigated for this purpose.
3.5 Serum Protein Adsorption Can Be Exploited to Create Novel NPs
Despite the daunting complexity of the interactions of serum proteins with nanoparticles, some efforts have been made to exploit these interactions in order to simplify the design of nanoparticles. Since the adsorption of proteins is essentially inevitable, Prapainop et al. used a small molecule to induce the binding and misfolding of the protein apolipoprotein B to trigger the specific receptor-mediated uptake of these particles in a macrophage cell line [47]. Although nanoparticles are efficiently taken up by macrophages in the absence of specific targeting ligands, this strategy has the potential to be a new direction for creating particles that are easier to synthesize (due to the greater stability of small molecules compared to protein-based targeting ligands) and that display specific targeting in the presence of serum proteins. Hamad-Schifferli’s group has controlled the deposition of serum proteins on gold nanorods to create agglomerate protein corona nanoparticles with high payload capacities [48]. These particles were capable of encapsulating both small molecules (Doxorubicin) and DNA that slowly leach over multiple days; the release of such cargo can also be triggered using the near-IR excitation of the gold nanorods. Unfortunately, both approaches are still preliminary, and it remains to be seen if either strategy can be used for directing the specific uptake of nanoparticles in tumors. This is likely to be the focus of future research.
3.6 The Impact of Serum Protein Adsorption Needs Be Acknowledged in Future Studies About Nanoparticle-Cell Interactions
Despite the increased popularity of research on nanoparticle serum protein adsorption in recent years, it remains unclear how this issue should be addressed. New strategies, in addition to PEGylation, are needed to mitigate the effects of serum protein adsorption on nanoparticle behavior. Nevertheless, the research presented here can be applied in many studies involving nanoparticles. To begin with, researchers should study the effects of nanoparticle-cell interactions in the presence of serum since nanoparticle behavior is known to be dramatically altered by serum protein adsorption [49]. Secondly, nanoparticles that undergo aggregation or large changes in size and shape due to protein adsorption should be avoided in place of more stable particles to improve the reproducibility of observed interactions with cells. Lastly, a greater focus on exploiting the unique behavior of protein adsorption and assembly on nanoparticle surfaces should be pursued since these proteins likely mediate many of the interactions that nanoparticles experience.
4 Endocytosis Is Dependent on Nanoparticle Properties
After entering blood circulation and interacting with serum proteins nanoparticles are subject to two competing processes: clearance through renal or phagocytic pathways and accumulation into the tumor microenvironment. Both processes occur simultaneously and the overall biodistribution and pharmacokinetics observed for nanoparticles is dependent on their relative rates. Although systemic factors, such as the perfusion rate of the liver and kidneys compared to that of the tumors and the leakiness of the tumor vasculature, play a major role in dictating these rates, the focus of this chapter will be on cellular factors, specifically the rates of cell uptake in cancer cells and macrophages. The dimensions of nanoparticles, in particular , play a major role in determining their rate of uptake into cells as illustrated by Fig. 3.
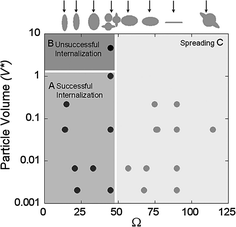
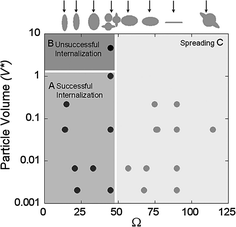
Fig. 3
Effects of geometric parameters on the phagocytosis of polystyrene particles. Reproduced with permission from [8]. Copyright (2006) National Academy of Sciences, U.S.A
4.1 Endocytosis of Nanoparticles into a Variety of Cancer Cells Is Sensitive to Nanoparticle Size
Nanoparticle size determines the curvature of the contact surface between the nanoparticle and the cell membrane and thus dictates the nature of the interaction between these two species. Since membrane curvature has been shown to initiate clathrin-mediated endocytosis as well as phagocytosis it is expected that cell uptake would be dramatically affected by nanoparticle geometry [12, 14]. Indeed, for polymer nanoparticles (PEG-acrylate derivatives) Gratton et al. showed that the efficiency of uptake by HeLa cells (cervical adenocarcinoma) decreased as the particle diameters increased from 200 nm to 5 μm among particles with aspect ratios of 1 [50]. Several groups also explored the uptake of spherical nanoparticles smaller than 100 nm in diameter. Chithrani et al. showed that gold nanoparticles ~50 nm in diameter exhibited greater levels of uptake in HeLa cells than other nanoparticles between 15 and 100 nm [51]. Lu et al. and Varela et al. also obtained similar results using mesoporous silica nanoparticles (maximum uptake at 50 nm diameter) and polystyrene nanoparticles (maximum uptake at 40 nm diameter) despite employing varying cell types [52, 53]. Recently, some of these results have been criticized because increasing particle size also favors sedimentation over diffusion thereby increasing the uptake of gold particles that are 50 nm in diameter or larger [54]. Sedimentation also affects polymer particles (PEG-diacrylate) but becomes significant only for particles with diameters greater than 325 nm (disc shaped with height of 100 nm) due to their reduced density compared to gold nanoparticles [55]. Presumably sedimentation applies to many other particles and may complicate the interpretation of current results but this has not been characterized for most particle types. However, the overall trends observed across a variety of particle types still suggest that particles with diameters around 40–50 nm exhibit more rapid endocytosis than particles of other sizes.
4.2 Aspect Ratio Is also Important in Determining the Rate of Endocytosis
The aspect ratios of nanoparticles also affect the contact areas between the particles and the cells and therefore affect endocytic rates across a variety of particle types. This was illustrated by Gratton et al., who observed higher uptake of polymer nanorods with dimensions of 450 × 150 × 150 nm compared to particles with dimensions of 200 × 200 × 200 nm in HeLa cells [50]. However, Agarwal et al. recently reported that polymer nanodiscs (PEG-diacrylate particles) [dimensions of 220 × 220 × 100 nm and 325 × 325 × 100 nm] were taken up faster than nanorods of the same material [dimensions of 400 × 100 × 100 nm and 800 × 100 × 100 nm] by HeLa, HEK293, HUVEC, and BMDC cells [55]. One difference between these studies is that the surfaces of the nanoparticles used by Gratton et al. were positively charged and charge also affects the interactions of nanoparticles with cells. To address these concerns, more studies are needed to decouple the effects of aspect ratio, particle size, core material composition, and surface capping ligands in order to establish design parameters that can be employed to control the endocytic rates of nanoparticles. These results will be promising since aspect ratio allows a second degree of freedom (in addition to particle size) as a means to design nanoparticles and manipulate their behavior in vivo.
4.3 Decorating the Nanoparticle Surface with Targeting Ligands Improves Endocytic Specificity by Increasing the Binding and Uptake of Nanoparticles into Target Cells
Another major challenge to nanoparticle design for in vivo applications is to manipulate the relative endocytic rates between cell types such that nanoparticle accumulation occurs more rapidly in target cell types and more slowly in off-target cell types. By decorating the nanoparticle surface with peptides, proteins, aptamers, or small molecules that specifically bind target cell types, the binding affinity and kinetics of binding of nanoparticles to the cell surface can be increased. Often, the binding affinity of the nanoparticle exceeds that of the free ligand. Wiley et al. modified the surface of gold nanoparticles with varying densities of transferrin and observed that the dissociation constant (K d ) of the nanoparticle from Neuro2A cells was between 4.9 and 0.014 nM, indicating that the nanoparticles bound these cells much more strongly than free transferrin (K d of 144 nM) or nanoparticles without transferrin (K d too large to measure reliably) [56]. Because this enhanced binding is due to the multivalent interaction to multiple cellular ligands, such functionalization may also directly trigger endocytosis by crosslinking surface ligands and initiating the assembly of a clathrin pit [57, 58].
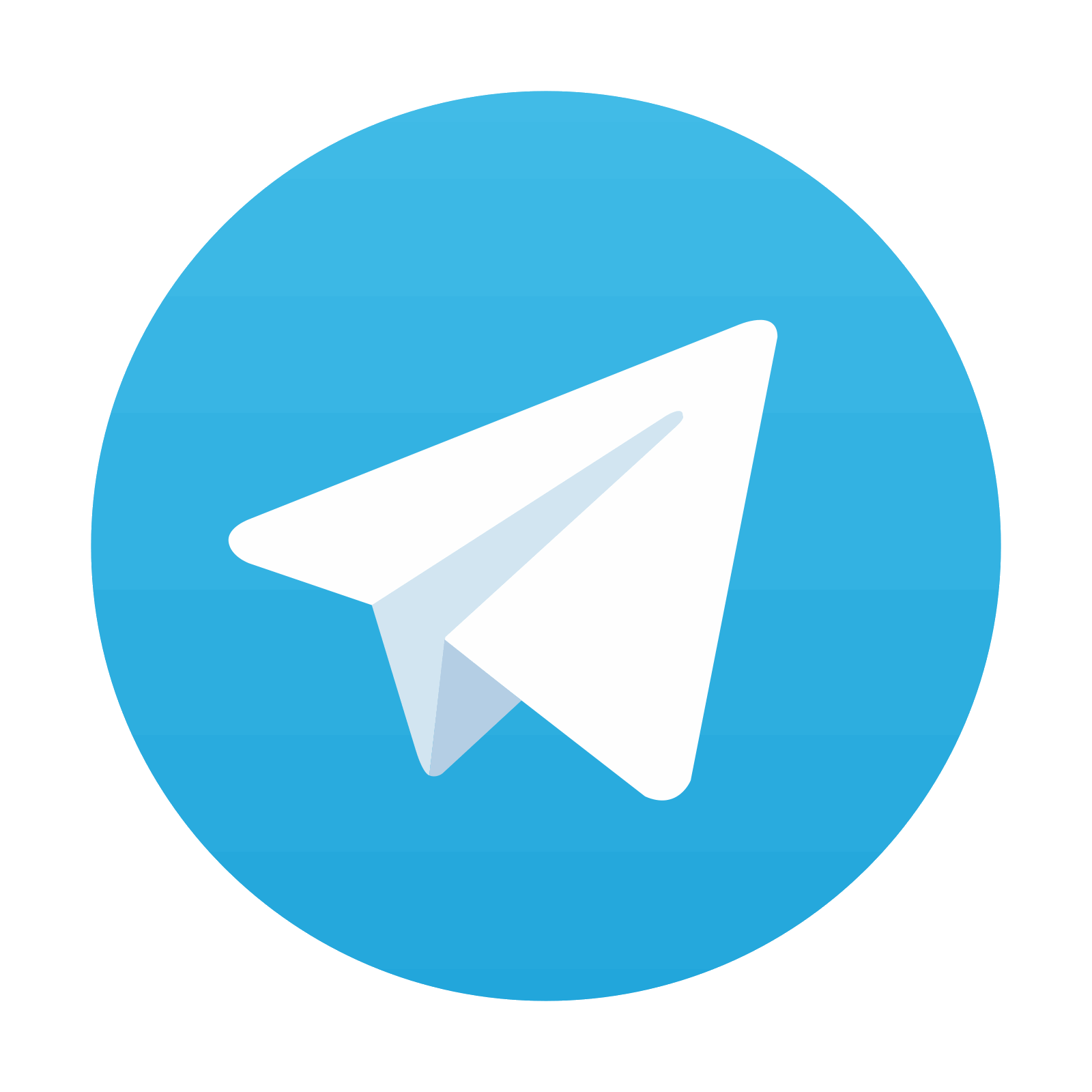
Stay updated, free articles. Join our Telegram channel
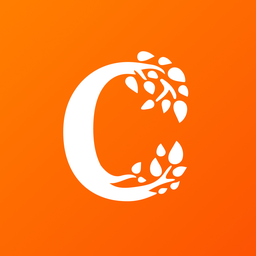
Full access? Get Clinical Tree
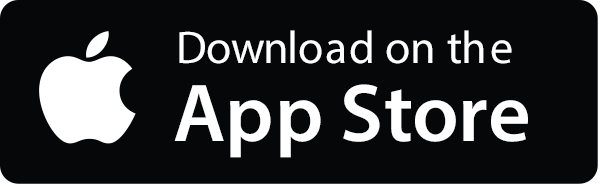
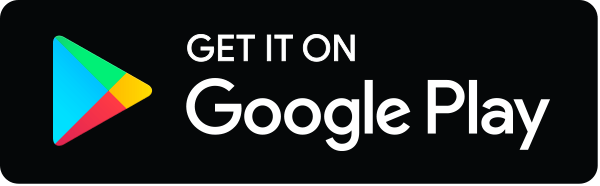