Fig. 1
Size scale of MNS as compared to biomolecules. MNS can be adapted to include biomolecules, drugs, or targeting and imaging molecules to form targeted MNS theranostic agents
Typically, MNS comprise a magnetic core and biocompatible coating and/or surface functionalization that allows integration of targeting agents and bio/chemotherapeutics (Fig. 2). Targeting agents have been coupled with MNS for both diagnostic imaging and therapy of specific tumors [5, 10–12]. The diagnostic applications of MNS stem from the MRI [13]. MRI offers clinicians the ability to noninvasively obtain anatomic and metabolic/functional information with high spatial and temporal resolution [13–15]. The technique is based on the response of water proton spin in the presence of an applied magnetic field when triggered with a RF pulse. When external magnetic field is applied, protons align in one direction. Application of the RF pulse perturbs the alignment and the protons relax to the original state via two independent relaxation processes: longitudinal (T 1) and transverse (T 2) relaxation that are used to generate the MR images. The difference in water concentration and local environment between organs and tissues results in intrinsic contrast in MR images.
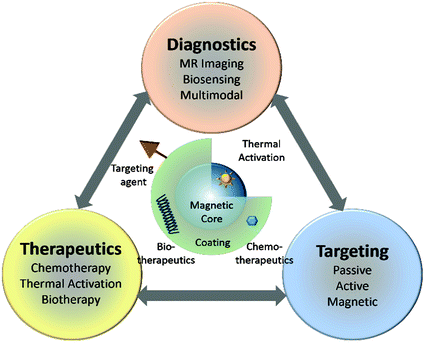
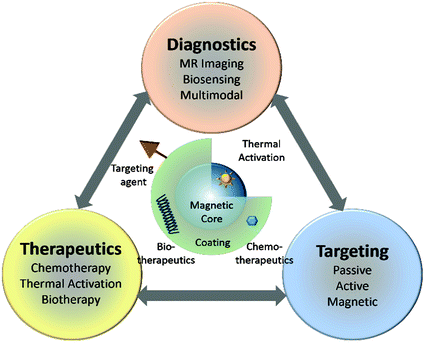
Fig. 2
Functional architecture of MNS and theranostic modalities. MNS are comprised of thermally active magnetic core and biocompatible coating and/or functionalization that allows integration of targeting agents and bio/chemotherapeutics
The spatial resolution as well as the sensitivity (S/N) of the MR images can be enhanced with the use of contrast agents. Paramagnetic molecular complexes, such as Gd(III) chelates, are used as T 1 contrast agents that increase signal intensity, i.e., higher r 1 relaxation, and appear bright in T 1-weighted images [16]. T 1 contrast agents are not covered in this chapter, however, a number of recent reviews have appeared [15–20]. MNS are used as T 2 contrast agents that decrease the signal intensity, i.e., higher r 2 relaxation, and appear dark in T 2-weighted images. When water molecules (protons, more specifically) diffuse into the periphery of the induced dipole moment by MNS, the T 2 relaxation time of the protons is shortened, which enhances the negative contrast that helps in differentiating between pathogenic targets and normal tissues in T 2 weighted MRI images (Fig. 3).
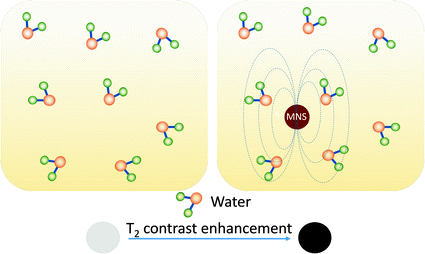
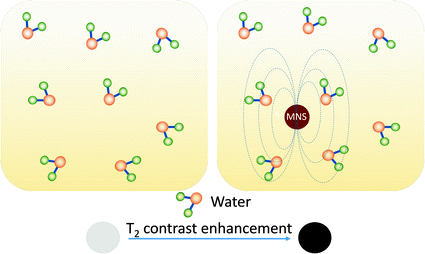
Fig. 3
T2 contrast enhancement in water due to MNS. When water molecules diffuse into the periphery of the induced dipole moment by MNS, the T 2 relaxation time of the water protons is shortened which enhances the negative contrast
Several MNS-based T 2 contrast agents (e.g., Feridex and Resovist) have been clinically approved [21, 22]. The MRI contrast enhancement effect is measured by the relaxation rate R 2 (s−1) and the relaxivity coefficient r 2, a slope of R 2 against MNS concentration. The R 2 relaxation rate of MNS is defined as
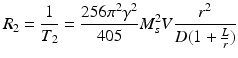
where T 2 is transverse relaxation time, γ is proton gyromagnetic ratio, M s is saturation magnetization, V is volume of MNS, D is diffusion coefficient of water molecules, r is radius of MNS core, and L is thickness of MNS surface coating. [23]. The higher relaxivity corresponds to a better contrast effect. Based on Eq. (1), MNS should have high magnetization (M s ), large volume (V), and thin surface coating (small L) for better contrast effect.
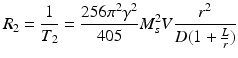
(1)
MNS can generate heat under external RF field that make them very useful in cancer therapeutics. Under an external RF field (typically a few hundred kHz), superparamagnetic MNS switch their magnetization direction along the field directions, back and forth. The frictions caused by the physical rotation of the MNS (Brownian relaxation) and the magnetization reversal within the MNS (Neel relaxation) lead to the loss of magnetic energy and the generation of thermal energy [24]. The capability of generating heat at any targeted areas can be used for directly killing cancer cells via the thermal therapy and/or as an actuator for bio/chemo therapy cargo release. The thermal activation of MNS is measured by the specific absorption rate (SAR) that is measured as the initial temperature rise of the MNS solution per unit volume or mass.
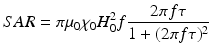
where μ 0 is vacuum permeability, χ 0 is equilibrium susceptibility, H 0 is RF field amplitude, and f is frequency of the external RF field. The higher SAR is crucial for clinical use since that would require a smaller amount of MNS to be injected into the patient. According to Eq. (2), SAR highly depends on various parameters such as the size, size distribution, shape, chemical composition and surface modification, and saturation magnetization of the particles [25]. In addition, it is clear that SAR values depend on the frequency f and the field amplitude H 0 of the applied field. However, in order to apply hyperthermia safely to patients and avoid any detrimental effect on healthy tissues due to electromagnetic radiation exposure, the H 0 f factor should not exceed a threshold that was experimentally estimated to equal 5 × 109 A m−1 s−1 [26]. Therefore, MNS with an exceptional SAR value that can generate heat under H 0 f limit is highly desirable.
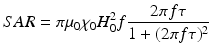
(2)
2 Synthesis and Characteristics of MNS: Prospects for Theranostics
For successful theranostic applications, MNS should be monodispersed and have uniform composition because the magnetic properties of MNS depend on the size, shape, and composition. It is clear from Eqs. (1) and (2) that the particles should possess high saturation magnetization and magnetic susceptibility, and be stable to a range of pH and salt concentrations. A key parameter for the magnetization of MNS is size. In a bulk magnetic material, all of the magnetic spins are aligned parallel to the applied magnetic field. However, in the nanoscale regime, a magnetically disordered spin-glass-like surface layer is formed. As the nanoparticle size decreases, such surface spin-canting effect becomes more pronounced and causes a drop in the saturation magnetization. While high saturation can be achieved with larger size particles, avoiding the surface-canting effect [27], the particle size should be under the superparamagnetic limit, which is typically less than ~20–30 nm for the majority of MNS. Further, the particles should have a coating or surface functional moieties that improve dispersion, biocompatibility, and provides a surface that can be functionalized. Strict attention to these parameters is essential during the design, synthesis, and formulation of MNS in order to be useful for in vivo applications.
MNS can be fabricated by either top-down (mechanical attrition) or bottom-up (chemical synthesis) approaches [9]. Since magnetic properties change with size and composition of MNS, chemical routes are preferred since they can synthesize MNS with uniform composition and size. The chemical methods include co-precipitation, microemulsion, thermal decomposition and/or reduction, hydrothermal synthesis, and polyol synthesis. Two excellent reviews describing MNS fabrication methods have recently appeared and we will provide only a brief summary here [28, 29].
The most common synthetic strategy involves aqueous precipitation of iron salts with in situ, or post-synthesis addition of surfactant [30]. This strategy has notable limitations yielding monodispersity [31]. The microemulsion method does produce MNS of narrower size distribution compared to aqueous precipitation, but suffers from low yields [32]. Recently, the thermal decomposition/reduction method has gained considerable attention since this technique offers fine control over the final particle size, shape, and crystal structure compared to other methods and is scalable [33, 34]. Monodispersed MNS are formed due to the reaction conditions that yield a quick nucleation step followed by slower growth phase. However, the reaction occurs in organic solvent containing hydrophobic stabilizers, which requires additional surface modifications to the MNS to impart aqueous stability.
Here, we discuss different types of MNS synthesized using chemical methods and their magnetic properties. Table 1 summarizes different MNS core materials, their magnetic properties, and r 2 relaxivity.
Table 1
Summary of MNS with core diameter, surface coating, magnetic properties, and r 2 relaxivity
MNS core material | Core diameter (nm) | Surface coating | Magnetic moment (emu/g) | B 0 (T) | r 2 (mM−1 s−1) | References |
---|---|---|---|---|---|---|
Fe3O4 (Resovist) | 4 | Carboxy-Dextran | N/A | 1.5 | 186 | [35] |
Fe3O4 (Feridex) | 5 | Dextran | 45 | 1.5 | 120 | [36] |
Dy-SiO2-(Fe3O4) n | 9 | DMSA | N/A | 9.4 | 397 | [37] |
Fe3O4 | 4–12 | DMSA | 25-101 | 1.5 | 78–218 | [38] |
Fe3O4 | 12 | Nitrodopa-PEG600 | N/A | 1.5 | 396 | Unpublished |
Fe3O4 | 14 | DSPE-mPEG1000 | N/A | 0.47 | 385 | [39] |
Fe3O4 | 58 | DSPE-mPEG2000 | 132 | 1.5 | 324 | [40] |
MnFe2O4 | 6–12 | DMSA | 68–110 | 1.5 | 208–358 | [38] |
CoFe2O4 | 12 | DMSA | 99 | 1.5 | 172 | [38] |
NiFe2O4 | 12 | DMSA | 85 | 1.5 | 152 | [38] |
Zn0.34Fe0.66OFe2O3 | 5 | DSPE-PEG | 54.1 | 0.55 | 34.7 | [41] |
Zn0.4Fe0.6Fe2O4 | 15 | DMSA | 161 | 4.5 | 687 | [42] |
Zn0.4Mn0.6Fe2O4 | 15 | DMSA | 175 | 4.5 | 860 | [42] |
Fe40Co60 | 7 | Phospholipid-PEG | 215 | 1.5 | 644 | [43] |
Fe/Fe3O4 | 15 | OAm-PEG | 164 | 3 | 220 | [44] |
Fe/Fe3O4 | 16 | DMSA | 139 | 1.5 | 312 | [45] |
Fe/MnFe2O4 | 16 | DMSA | 149 | 0.47 | 356 | [46] |
2.1 Ferrite MNS
Fe3O4 MNS are extensively used in biomedicine because of their biocompatibility and ease of synthesis [47, 48]. The magnetic moment of superparamagnetic Fe3O4 MNS is dependent on the size with smaller particles producing lower magnetic moments [49]. Hence, the size of Fe3O4 MNS can be tuned by changing the reaction conditions such as reflux temperature, reflux time, and heating rate. The magnetic moment of Fe3O4 MNS was tuned from 25 to 43, 80, and 102 emu/(g Fe) with change in the size from 4, 6, 9, and 12 nm, respectively (resulting in r 2 values of 78, 106, 130, and 218 mM−1 s−1) [27]. Further, the magnetic moment of Fe3O4 MNS can be modified by doping transition divalent metal ions (Co2+, Ni2+, Mn2+, and Zn2+). By adding different metal precursors during Fe3O4 synthesis, monodisperse MFe2O4 MNS were synthesized [38]. The metal ferrite nanostructures have an inverse spinel crystal structure composed of face-centered cubic packed lattice of oxygen atoms with octahedral sites (Oh) occupied by Fe3+ and M2+ ions and tetrahedral sites (Td) occupied by Fe3+ ions (Fig. 4a). The magnetic spins of the ions at the Oh and Th sites align opposite to each other. Hence the spins of Fe3+ ions at Oh and Th cancel each other and net magnetization of MFe2O4 MNS is decided by magnetic moment of M2+ ions (Fig. 4b). The magnetization of NiFe2O4, CoFe2O4, Fe3O4, and MnFe2O4 were found to be 85, 99, 101, 110 (emu/g metal ions), respectively, depending upon the magnetic moments of the M2+ ions. This resulted in r 2 values of 152, 172, 218, and 358 mM−1 s−1 (Fig. 4c) [38].
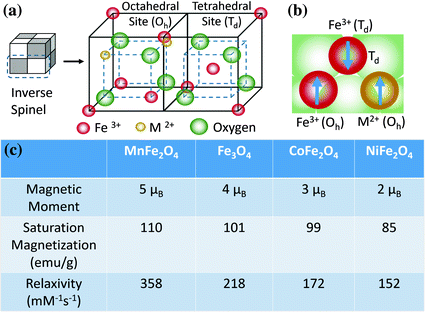
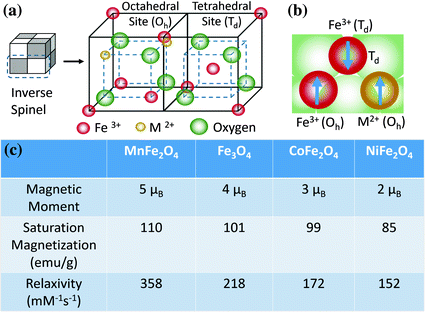
Fig. 4
MFe2O4 (where M = Mn, Fe, Co, Ni) MNS with inverse spinel structure and its magnetic spin alignments. The mass magnetization values and r 2 relaxivity values of MFe2O4 MNS are proportional to the magnetic moments of the divalent ions (M2+) [38]
Interestingly, doping of nonmagnetic Zn in Fe3O4 and MnFe2O4 MNS resulted in (Zn x Fe1−x )Fe2O4 and (Zn x Mn1−x )Fe2O4 MNS, respectively, that exhibit extremely high net magnetic moment and r 2 relaxivity [42]. The magnetization of (Zn x Mn1−x )Fe2O4 was dependent on the Zn doping level and were found to be 125, 140, 154, 166, 175, and 137 emu/g metal ions for x = 0, 0.1, 0.2, 0.3, 0.4, and 0.8, respectively, resulting in the r 2 values of 422, 516, 637, 754, 860, and 388 mM−1 s−1, respectively (Fig. 5). The r 2 value for Zn0.4Mn0.6Fe2O4 MNS is the maximum r 2 value of reported to date for MNS [42], eight times higher than r 2 of Feridex [36]. (Zn x Fe1−x )Fe2O4 MNS exhibited a similar trend, but the magnetization and r 2 values were slightly lower than (Zn x Mn1−x )Fe2O4 MNS (Fig. 5) [42].
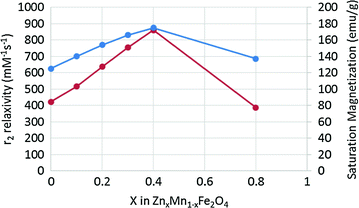
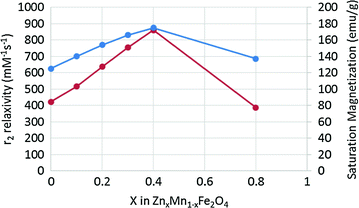
Fig. 5
Saturation magnetization and r2 relaxivity (at 4.5 T) of (Zn x Mn1−x )Fe2O4 MNS at different Zn2+ doping levels. The (Zn x Mn1−x )Fe2O4 MNS showed significantly high r 2 relaxivities compared to conventional iron oxide MNS [42]
2.2 Metallic MNS
MNS based on transition metals of Fe, Co, Ni, and their alloys have higher magnetic moments than their oxide counterparts [44, 50–53]. Saturation magnetization of bulk FeCo (240 emu/g) and Fe (218 emu/g) is particularly high compared to bulk Fe3O4 (90 emu/g). Using the same mass of metallic MNS would then produce a far greater impact than their oxide counterparts, improving the T 2 contrast enhancement and therapeutic efficacy of drug delivery. However, the metallic MNS carries their own set of disadvantages like chemical instability, leaching of the noniron elements, and toxicity that renders them questionable for in vivo applications [9, 53, 54]. In addition, these pure metal nanoparticles are also ferromagnetic at room temperature, rather than superparamagnetic. This means that once they are magnetized, they will remain that way regardless of whether an external magnetic field is withdrawn, resulting in an aggregation. A number of reports have been published demonstrating coatings that prevent aggregation and ensure chemical stability of metallic MNS. Options under consideration include inert metals, such as Au and Ag, peptide capping ligands, ferrites, graphite, and silica [43, 44, 52, 55–57]. For example, after graphitic shell coating, FeCo MNS were stable up to 1 month and showed very high magnetic moment (215 emu/g) and r 2 relaxivity (644 mM−1 s−1), which is far superior to conventional ferrite MNS [43, 52]. Crystalline Fe3O4 shell was also used to protect metallic Fe MNS that resulted in M s and r 2 of 164 emu/g and 220 mM−1 s−1, respectively [44, 56]. Co MNS were coated with Au shell to provide an inert, biocompatible and stable shell with a well-known surface chemistry. Though the bulk saturation magnetization of Co is ~160 emu/g, the measured value was found ~100 emu/g after Au coating, which was still higher than Fe3O4 MNS (75–80 emu/g) [55].
2.3 Multifunctional MNS
Hybrid MNS with two or more different functional units, such as Au–Fe3O4, FePt–CdS, and Fe2O3–carbon nanotube can be synthesized through seed mediated growth. In such a heterogeneous nanostructure, each unit exhibits its unique magnetic, optical, or electronic properties [10, 58–60]. Au–Fe3O4 nanostructures were prepared that preserved the optical property of Au (plasmonic absorption at ~530 nm) as well as the magnetic property of Fe3O4 MNS (M s = 80 emu/g) [61]. This approach was extended to prepare semiconductor–metal alloy [62], semiconductor–metal oxide [63], and carbon nanotube–metal oxide complex [64]. MNS have been coupled with a wide range of fluorophores for multimodal imaging applications [65–67]. Lastly, T 1/T 2 MRI agent were prepared by conjugating Gd(III) based chelating agent with MNS [68].
3 Coating and Functionalization of MNS
In order to apply MNS in vitro and subsequently in vivo, the surface needs to be functionalized so that it; (i) protects against agglomeration; (ii) provides biocompatibility and chemical handles for the conjugation of drugs and targeting ligands; (iii) limits nonspecific cell interactions; and (iv) enhances MNS pharmacokinetics [69]. A diverse group of organic and inorganic coatings has been investigated including DMSA [27], PEG [70, 71], dextran [72], chitosan [73], liposomes [74], gold [75], and silica [76]. MNS coating can be achieved via a number of approaches, including in situ coating, post-synthesis adsorption, and post-synthesis end grafting [15]. Here, we discuss some of the most common coatings, their methods of attachment, and examples in cancer targeting.
3.1 Poly(Ethylene Glycol) (PEG)
PEG is a neutral and amphiphilic polymer that has been used clinically as excipients in FDA approved pharmaceutical formulations [77]. PEG coating of MNS improves their dispersion in biological media and increases blood circulation time since they are not readily recognized by the reticuloendothelial system (RES) [78]. Lutz et al. demonstrated in situ coating of PEG onto Fe3O4 MNS under aqueous conditions [79] while PEG grafting was achieved by single-point chemical anchoring through different functional groups including silanes [70], phosphate derivatives [80] and dopamine [81]. Peng and Sun reported ligand exchange with bifunctional PEG with dopamine [56]. Most recently, nitrodopamine has been proposed as an ultrastable chemical anchor for MNS [82]. We have developed MNS with high buffer stability by coating Fe3O4 with bifunctional PEG conjugated with nitrodopamine and carboxylate terminal groups [83]. The nirodopamine was covalently attached to the Fe3O4 surface by one end and the carboxylate group at the other end was kept open to functionalize with targeting ligands or therapeutic agents.
3.2 Dextran
Dextran is a branched polysaccharide comprised of glucose subunits and is widely used for MNS coatings because of its biocompatibility and polar interactions (chelation and hydrogen bonding). Addition of dextran during synthesis of Fe3O4 via the co-precipitation method resulted in dextran coated Fe3O4 MNS [84]. Subsequent iterations of this method produced clinically approved ferumoxtran-10 (AMI-277) and ferumoxides (AMI-25) [85–90]. These two structures have cores of ~5 nm, but differ significantly in dextran coating thickness (20–40 nm vs. 80–150 nm) which results in varying blood circulation times (24 h for ferumoxtran and 2 h for ferumoxides) [35]. Since the dextran molecules are adhered nonspecifically through hydroxyl interactions with the iron oxide core, there is always a possibility of desorption [91]. In order to prevent this, the dextran polymers were chemically cross-linked on MNS surface [92]. Using this strategy, clinically approved ferumoxytol [93] and ferucarbotran [22] have been synthesized.
3.3 Silica
Silica coating on MNS is popular because of the ease of synthesis and aqueous stability. By hydrolyzing silica precursors in basic solution, a uniform and thickness-controllable silica coating on MNS was obtained [76, 94, 95]. The silica shell have been used as a carrier for anticancer drugs (e.g., paclitaxel) and fluorescent molecules (e.g., fluorescein isothiocyanate (FITC)) [96]. Imparting additional functionalities to the silica coating have enabled targeting and labeling functionality. By addition of 3-aminopropyl-triethoxysilane (APS) to the silica precursors, we have coated silica shells with primary amine groups with controlled thickness [97]. Similarly, by reacting APS with isothiocyanate functionalized fluorescent dyes, Lu et al. were able to develop fluorescent MNS, a multimodal diagnostic agent [94]. Currently, silica coated MNS is available as ferumoxil (AMI-121), an orally ingested T 2 contrast agent for delineation of the intestinal loops from adjacent tissues and organs [21].
4 Pharmacokinetics and Biodistribution
The two most important factors that determine MNS pharmacokinetics are their surface characteristics and hydrodynamic size [98, 99]. Interplay of these properties with the reticuloendothelial system (RES) clearance determines plasma lifetime (blood circulation time). In RES clearance, circulating opsonin proteins adsorb to MNS surface (opsonization) that are recognized and removed from the bloodstream by tissue macrophages (Fig. 6). It has been shown that MNS with a hydrodynamic diameter of 10–100 nm are pharmacokinetically optimal for in vivo applications [100]. MNS smaller than 10 nm are subject to tissue extravasation and renal clearance, whereas those larger than 100 nm are quickly opsonized and eliminated from the circulation via the RES [31]. Decuzzi et al. produced models suggesting that within this range, smaller size nanostructures have longer blood circulation time [101].
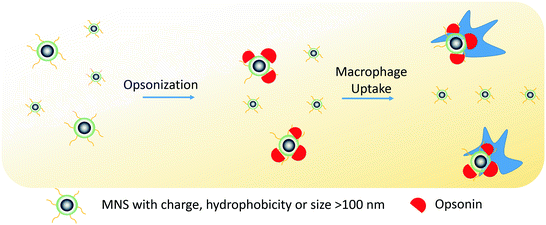
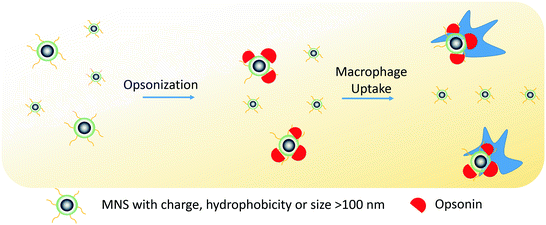
Fig. 6
Schematic illustration of RES clearance of MNS. MNS larger than 100 nm are absorbed by circulating opsonin proteins that are recognized by macrophages and removed from the bloodstream
With respect to the surface properties, charge can affect pharmacokinetics of MNS by enhancing their interactions with the plasma proteins and nontargeted cells, resulting in short blood circulation time [102]. In addition, hydrophobic groups on the surface of MNS induce the agglomeration of the MNS upon injection, leading to rapid removal by the RES. The rate of clearance, however, can be reduced by modification of MNS surfaces with coatings that resist RES interactions. As mentioned, surface modification with molecules such as the hydrophilic PEG has been a hallmark solution to many pharmacokinetic problems, including MNS [103, 104]. PEG chains linked to MNS reduce opsonization and macrophage uptake processes through steric repulsion, prolonging their circulation times [78, 105].
MNS biodistribution and cell uptake is significantly influenced by their physicochemical properties [99, 106, 107]. For example, it has been reported that MNS smaller than 150 nm accumulates in the bone marrow, heart, kidney, and stomach [108] while MNS larger than 150 nm are found in the liver and spleen [109]. Villanueva et al. showed that the charge and nature of surface functionalizing molecules on MNS affected their uptake of cancer cells [110]. They found that cells had effective uptake of positively charged aminodextran-MNS, minimal uptake of neutral charged dextran coated MNS, and low uptake of negatively charged DMSA-coated MNS [110]. Chouly et al. have found that negatively charged MNS gets opsonized quicker than neutral MNS and had greater liver uptake [99].
5 Targeting of MNS to Localized Cancer Tumors
The targeting of MNS for selected tumor tissues is critical in both diagnostic imaging and therapeutics [5, 10, 11]. Since nonspecific cell binding can place healthy tissue at risk, MNS have been engineered to target tumor tissues through passive and active targeting approaches.
5.1 Passive Targeting
Passive targeting uses the predetermined physicochemical properties of MNS to specifically migrate to selected tissues. The most common example of passive targeting is the enhanced permeability and retention (EPR) effect where MNS smaller than 200 nm can accumulate in many tumor tissues passively in solid tumors [111]. The compromised vasculature of a solid tumor facilitates passive MNS extravasation from the circulation into the tumor interstitium (Fig. 7) [112]. By contrast, endothelial cells of normal tissue vessels are closely packed and present a barrier for MNS penetration. However, passive targeting is limited to specific tumors since success of EPR effect depends on a number of factors such as lymphatic drainage rate, degree of capillary disorder, and blood flow which varies in different tumor types [113, 114].
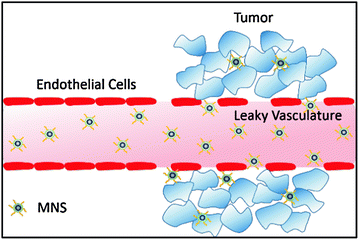
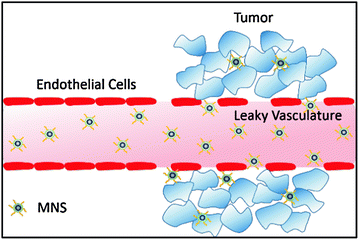
Fig. 7
Passive targeting of MNS via enhanced permeability and retention (EPR) effect. The compromised vasculature of a solid tumor facilitates extravasation of MNS of size less than 200 nm from the circulation into the tumor interstitium, while endothelial cells are closely packed and present a barrier for MNS penetration
5.2 Active Targeting with Targeting Agents
Because passive targeting is available for only certain types of tumors and does not necessarily guarantee internalization of MNS by targeted cells, MNS can be modified with tumor-selective agents to employ active targeting [115, 116]. These agents are complementary to unique receptors that are overexpressed or present on tumor cells. A variety of targeting agents have been used for MNS, depending on the specific target, and these are reviewed elsewhere [5, 10]. Some of the studies include: small organic molecules [115, 117, 118], peptides [119], proteins [120], and antibodies [27]. The density and molecular organization of these ligands significantly influence MNS binding to target cells due to the multivalency phenomenon [121]. Some of the targeting agents can be used to facilitate MNS internalization into cells, primarily via endocytosis [5]. However, synthesis of these targeting agents is expensive and involves complicated chemistry. Therefore, the process of scaling up the synthesis is challenging and may be a hurdle for clinical applications.
5.3 Active Targeting with External Magnetic Field
Accumulation of MNS can be realized by applying external magnetic field on the target site, a unique feature for MNS. Magnetic targeting has been studied for a number of tumor models [122, 123]. This technique was successfully implemented in a clinical trial to deliver the chemotherapeutic, doxorubicin, to hepatocarcinoma cells [124]. David et al. have explored magnetic targeting to brain tumors with PEI functionalized MNS [102, 122]. While successful, the efficacy of magnetic targeting is limited to target tissue that is close to the body’s surface, since the magnetic field strength decreases with the distance from the magnetic source.
6 MNS for Diagnostic Imaging of Cancer
The diagnostic imaging applications of MNS have been realized as T 2 contrast agents in MR imaging over the past 20 years [13–15, 28, 125]. Owing to their significant deposition in liver, several MNS-based clinically approved T 2 contrast agents (Feridex I.V.®, Resovist®, and Gastromark®) have been used for liver imaging of humans [21, 22]. In order to extend MR visibility to image tumor at other parts, targeting agents have been coupled to MNS [85]. For example, Artemov et al. used Fe3O4 MNS conjugated with biotinylated Her-2/neu antibody Herceptin to generate strong T 2 contrast in breast cancer cell lines (AU-565, MCF-7, and MDA-MB-231) overexpressed with tyrosine kinase Her-2/neu receptors [126]. Contrast observed in MR images was found to be proportional to the expression level of kinase her-2/neu receptors for the given cell lines. Gao et al. successfully performed targeted MR imaging of human colon carcinoma xenograft tumors in mice by conjugating a cancer-targeting antibody (rch 24 mAb), to an 11 nm Fe3O4 MNS [127]. T 2 and T 2*-weighted MR images acquired before and after injection showed that the tumor site turned dark as early as 10 min after the injection of the rch 24 mAb conjugates and became darker and bigger until 24 h. In contrast, the Fe3O4 MNS without rch 24 mAb showed nearly no variation after injection [127]. Sun et al. reported c(RGDyK) peptide-coated Fe3O4 MNS and demonstrated their in vivo tumor-specific targeting capability [128]. When administrated intravenously in a mice bearing U87MG tumors, the c(RGDyK) peptide-coated Fe3O4 MNS accumulated preferentially in the integrin αvβ3-rich tumor area resulting in a significant drop in the tumor MR signal intensity [128].
Other than target-specific molecular imaging, MNS-based contrast agents have been used in MRI for cell-based therapy since cells must be tracked in vivo to optimize cell therapy [129–134]. MRI-based immune cell tracking using MNS has been applied to many types of preclinical studies, such as tumor targeting of cytotoxic T cells and natural killer cells [135, 136], organ-specific targeting of autoimmune T cells [137], and neural stem cells [138]. De Vries et al. have shown that in vivo magnetic resonance tracking of MNS labeled dendritic cells is feasible in humans in conjunction with detailed anatomical information in melanoma patients. In contrast to scintigraphic imaging, MRI allowed assessment of the accuracy of dendritic cell delivery and of inter- and intranodal cell migration patterns [139–143]. Recently, Bulte et al. evaluated the long-term clinical tracking of MNS labeled stem cells after intracerebroventricular transplantation in an 18-month-old patient with global cerebral ischemia [144]. Twenty-four hours post-transplantation, MRI was able to detect hypointense cells in the occipital horn of the lateral ventricle. The signal gradually decreased over 4 months and became undetectable at 33 months.
To improve T 2 contrast for advanced MR imaging, researchers have been studying various parameters that affect the r 2 relaxivity of MNS. In the following sections, we discuss the parameters that have improved T 2 contrast of MNS for cancer diagnostic imaging. They have been divided into four categories; (i) size, shape, and composition control; (ii) nanoassembly of MNS; (iii) coating of MNS; and (iv) MNS with metal core.
6.1 Size, Shape, and Composition Control
According to Eq. 1, the R 2 of MNS is proportional to saturation magnetization and volume. Since the saturation magnetization of an MNS is proportional to its size due to surface spin-canting effects, the r 2 value of MNS can be increased by increasing the size of MNS. However, for biological imaging applications, the hydrodynamic size of MNS should be below 100 nm in order to have longer circulation times and to avoid nonspecific uptake [145]. Cheon et al. investigated the size effect where Fe3O4 MNS of diameter 4, 6, 9, and 12 nm resulted in the r 2 relaxivity of 78, 106, 130, and 218 mM−1 s−1, respectively (Fig. 8). The MR contrast changed from light gray to black or from red to blue in color-coded images (Fig. 8) [27]. The 9 nm Fe3O4 MNS conjugated with Herceptin was used to image a breast cancer cell line, SK-BR-3, which possesses overexpressed HER2/neu cancer markers. Herceptin was selected due to its specific binding properties against a HER2/neu receptor. In the T 2-weighted MR images, treatment of 9 nm Fe3O4 MNS-Herceptin probe conjugates to the SK-BR03 breast cancer cell lines resulted in the significant negative contrast of the MR images compared to nontreated cell lines [27]. Chen et al. studied the size effect of polyvinylpyrrolidone (PVP)-coated Fe3O4 MNS (core size 8, 23, 37, and 65) on MRI of hepatic lesions in vivo [146]. PVP-Fe3O4 MNS with core size 37 and 65 nm showed higher r 2 relaxivity (239 and 248 mM−1 s−1, respectively) compared to other sizes. When administered in nude mice bearing orthotopic Huh7 liver cancer, PVP-Fe3O4 MNS with 37 nm core size showed higher contrast change compared to Feridex in T 2 and T 2* weighted MR images [146].
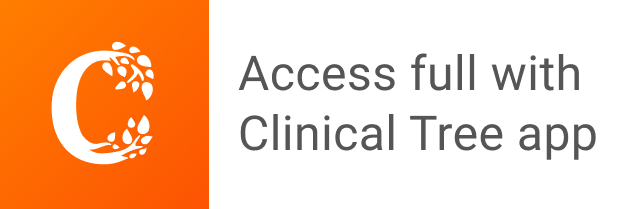