Megakaryocyte Structure and Platelet Biogenesis
Joseph E. Italiano Jr.
John H. Hartwig
INTRODUCTION
The process of making platelets is both amazing and bewildering. In the humans, 108 megakaryocytes are born each day, as the same number of mature megakaryocytes move to the bone marrow sinusoids and undergo terminal differentiation to release 1011 platelets. The ability to culture megakaryocytes, primarily from mice, and follow their maturation and platelet production in vitro, is beginning to provide information on the sequence of events leading to platelets and the molecules that regulate and power this process. In general, proplatelet and platelet production are driven by microtubule assembly and sliding, while mature platelet-based hemostasis is an actin-controlled process. In this chapter, we summarize the current knowledge of how megakaryocytes subfragment into platelets.
HISTORY/THE PROPLATELET MODEL
The process by which platelets are generated has been studied for over 100 years. In 1869, Italian biologist Giulio Bizzozero described a “cellule gigantic” up to 45 µm in diameter. However, it was not until 1906 that James Homer Wright discovered that platelets derive from these large precursor cells called megakaryocytes.1 Wright established that platelets did not emerge in embryos until megakaryocytes appeared and demonstrated platelets coming off the cortex of megakaryocytes in the human, dog, rabbit, guinea pig, pig, and opossum. Many different theories have been proposed to explain how megakaryocytes produce platelets. Early microscopists documented that maturing megakaryocytes became filled with membranes, called demarcation membranes, and hypothesized that this membrane system divided the cytoplasm into “platelet territories” in which individual platelets were formed.2,3 Release was postulated to occur by the complete fragmentation of the megakaryocyte cytoplasm into thousands of platelets. Later, it was observed that megakaryocytes extend long processes called proplatelets whose shafts are decorated with platelet-sized beads.4,5 The classical “proplatelet theory” of platelet production was introduced by Becker and De Bruyn who first proposed that megakaryocytes extend long, sinus processes that fragment into platelets.6 Later, Radley and Haller developed the “flow model,” postulating that platelets derived from bead-like structures connected by thin cytoplasmic bridges.7,8 Proplatelets have been observed both in vitro and in vivo across species and the bulk of experimental evidence now supports the involvement of proplatelets in platelet production.9,10,11,12,13,14,15 Although the basic idea that proplatelets give rise to platelets is correct, the precise mechanism of platelet release has only recently been established.
CYTOPLASMIC MATURATION
As megakaryocytes mature in the bone marrow, they customize their cytoplasm and membrane systems for platelet formation. Before a megakaryocyte has the capability to produce platelets, it expands significantly to diameters of 50 to 100 µm and fills its cytoplasm with high levels of ribosomes producing plateletspecific proteins. Multiple rounds of endomitosis, a process that amplifies the DNA content by as much as 64-fold, facilitate this rapid cytoplasmic expansion.16,17,18 In addition to DNA expansion, megakaryocytes undergo considerable maturation as internal membrane systems, organelles, and granules are assembled during their development. In particular, their cytoplasm, except for the most cortical regions, becomes densely filled with an expansive and interconnected membranous network of flattened cisternae and tubules19 (FIGURE 24.1) This unique internal membrane system was initially called the demarcation membrane system (DMS) and as mentioned above was thought to divide the megakaryocyte cytoplasm into fields where individual, preformed platelets would assemble and release. This theory of cytoplasmic fragmentation as a mechanism for platelet biogenesis has lost support and DMS membranes are now thought to function primarily as a reservoir that continuously provides membrane for the growth of proplatelets.8,20,21
OVERVIEW OF PROPLATELET PRODUCTION
The discovery of thrombopoietin (Tpo) in 1994 has permitted major advances in the study of thrombopoiesis.22,23,24,25 Tpo has facilitated the development of methods to culture bone marrow cells committed to the megakaryocyte lineage and that have successfully reconstituted the transition of terminally differentiated megakaryocytes into functional platelets in vitro. Megakaryocytes cultured in the presence of Tpo extend numerous proplatelets, consistent with the flow model. These proplatelets are structurally similar to those seen in vivo extending into bone marrow sinusoids and within the bloodstream.9,10,26 Platelets released from megakaryocytes grown in vitro are also structurally and functionally similar to those found in vivo. Culture-derived platelets are of 2 to 3 µm diameter, disc-shaped fragments containing a microtubule-based marginal band and are responsive to platelet agonists.
Video microscopy has revealed the spatial and temporal changes leading to the generation of proplatelets26 (FIGURE 24.2A-C). The production of platelets from megakaryocytes involves an elaborate process that converts the megakaryocyte cytoplasm into long, branched proplatelets upon which platelets are assembled. The process begins at a single position
on the megakaryocyte plasma membrane. From this region, a large pseudopod forms, initially as a thickening of the plasma membrane. Over 4 to 8 hours, the pseudopod continues to extend and narrow into a tubule with an average diameter of 2 to 4 µm. Multiple bulges, termed swellings, each similar in size to a platelet, form along the length of the proplatelet, giving them the appearance of beads connected by thin bridges (FIGURE 24.2D). A teardrop-shaped tip develops at the proplatelet terminus and is believed to be the primary site of platelet maturation. Formation of additional proplatelets continues from the original erosion site and spreads in a wave-like manner throughout the remains of the cell until the megakaryocyte cytoplasm is entirely converted into an extensive and complex network of interconnected proplatelets. The multilobed “naked nucleus” remains in the megakaryocyte cell body as it is transformed into proplatelets. The events involved in platelet release from proplatelets are described below.
on the megakaryocyte plasma membrane. From this region, a large pseudopod forms, initially as a thickening of the plasma membrane. Over 4 to 8 hours, the pseudopod continues to extend and narrow into a tubule with an average diameter of 2 to 4 µm. Multiple bulges, termed swellings, each similar in size to a platelet, form along the length of the proplatelet, giving them the appearance of beads connected by thin bridges (FIGURE 24.2D). A teardrop-shaped tip develops at the proplatelet terminus and is believed to be the primary site of platelet maturation. Formation of additional proplatelets continues from the original erosion site and spreads in a wave-like manner throughout the remains of the cell until the megakaryocyte cytoplasm is entirely converted into an extensive and complex network of interconnected proplatelets. The multilobed “naked nucleus” remains in the megakaryocyte cell body as it is transformed into proplatelets. The events involved in platelet release from proplatelets are described below.
THE CYTOSKELETAL MECHANICS OF PLATELET PRODUCTION
The Cytoskeleton of the Resting Platelet
As discussed in Chapter 23, resting blood platelets have a welldefined microtubule cytoskeleton that functions to maintain the discoid shape of the resting platelet, as well as a robust filamentous (F)-actin cytoskeleton that both stabilizes the resting cells and is reorganized to generate the active shapes of spread platelets.27,28,29 The microtubule skeleton is composed of an approximately 100 µm long microtubule and multiple dynamic short microtubules that shape the platelet into its discoid form by coiling around the edge of the cell.30,31 In megakaryocytes, this microtubule cytoskeleton is the “engine” of platelet production (FIGURE 24.3). In the resting platelet, an actin-based membrane skeleton also plays a major role in stabilizing the discoid structure and in organizing the topology of surface receptors.32,33 Hence, to understand the ultimate steps in platelet release, knowledge of the structure of the actin membrane skeleton in the resting platelet is essential.
Although the actin cytoskeleton of cells is complex, four proteins are critical in defining the architecture of the platelet membrane: spectrin, filamin, the von Willebrand factor receptor (vWfR), and F-actin. Spectrin forms a planar network linked together by the ends of F-actin to support the plasma membrane.33,34,35 Disruption of spectrin tetramers (the functional unit of spectrin) prevents proplatelet elaboration and destabilizes released forms. Filamin links the GP1bα subunits of the vWfR to underlying F-actin.32,36,37 The importance of the filamin-vWfR interaction has been established in both humans and mice where loss of expression of either molecule in platelets results in macrothrombocytopenia and bleeding.38,39
Two isoforms of filamin are expressed in platelets.40,41,42,43 The most abundant isoform, filamin A (flnA), is expressed at a concentration of 5 µM (12,000 molecules per platelet). FlnA is in 20-fold molar excess over the second isoform filamin B.44 Expression of FlnA increases dramatically in megakaryocytes in culture on days 2 to 4, particularly on day 4 when proplatelet formation begins. FlnA and FlnB are large elongated homodimers whose monomers are assemblies of an amino-terminus high-affinity actin-binding site followed by 24 Ig-like repeats, each composed of seven runs of β-structure.45,46 Repeats 15 and 16 and repeats 23 and 24 are interrupted by hinge regions that are sites for calpain cleavage. Repeat 24 self-associates to dimerize the subunits.
In addition to binding and cross-linking F-actin, filamin molecules are scaffolds for the binding of additional proteins, including GP1bα, β-integrin tails, FilGAP, ralA, migfilin, and Syk.47 The interaction of flnA with GP1bα is now understood at the atomic level. Binding occurs between a short run of
β-strand in the GP1bα cytoplasmic tail (amino acids 566 to 577) and two of the β-strands in flnA repeat 17; the GP1bα site binds in an antiparallel fashion between a groove formed between the C and D β-strands of FlnA.36,37,48 FlnB shares the identical GP1bα binding site. Tight binding between these two proteins requires each GP1bα subunit in a vWfR to be bound to each repeat 17 in a filamin molecule. As there are 25,000 GP1bα chains expressed on the surface of a platelet, the stoichiometry for this interaction is ideal, and biochemical experiments have demonstrated that 90% to 95% of GP1bα is bound constitutively to flnA.49 The stoichiometry of GP1bα binding to filamin is 1:1. Moreover, biochemical experiments indicate that 90% to 95% of GP1bα is bound constitutively to flnA.49
β-strand in the GP1bα cytoplasmic tail (amino acids 566 to 577) and two of the β-strands in flnA repeat 17; the GP1bα site binds in an antiparallel fashion between a groove formed between the C and D β-strands of FlnA.36,37,48 FlnB shares the identical GP1bα binding site. Tight binding between these two proteins requires each GP1bα subunit in a vWfR to be bound to each repeat 17 in a filamin molecule. As there are 25,000 GP1bα chains expressed on the surface of a platelet, the stoichiometry for this interaction is ideal, and biochemical experiments have demonstrated that 90% to 95% of GP1bα is bound constitutively to flnA.49 The stoichiometry of GP1bα binding to filamin is 1:1. Moreover, biochemical experiments indicate that 90% to 95% of GP1bα is bound constitutively to flnA.49
In turn, filamin is linked to underlying F-actin. This interaction organizes the topologic arrangement of the vWfR on the surface of mature platelets, and presumably on the surface of maturing proplatelets and preplatelets, as well. The final result is a linear alignment of vWfR on the platelet surface, a process that stabilizes and adheres the spectrin membrane skeleton to the plasma membrane.50 The ends of actin filaments are bound on their sides at multiple points by flnA and on their ends by the spectrin tetramers where they cross-link the spectrin strands into a two dimensional lamina under the cytoplasmic surface of the plasma membrane. Curiously, platelets express both erythroid and nonerythroid spectrins.33,34,35 This maybe due, in part, to the common stem cell origin of platelets and red blood cells or to the necessity of coping with membrane deflections during blood flow in similar fashion to erythrocytes; however, heterodimers of nonerythroid spectrin have lower affinity for one another compared to the nonerythroid molecules. This allows them to undergo dynamic associationdissociations in situ.
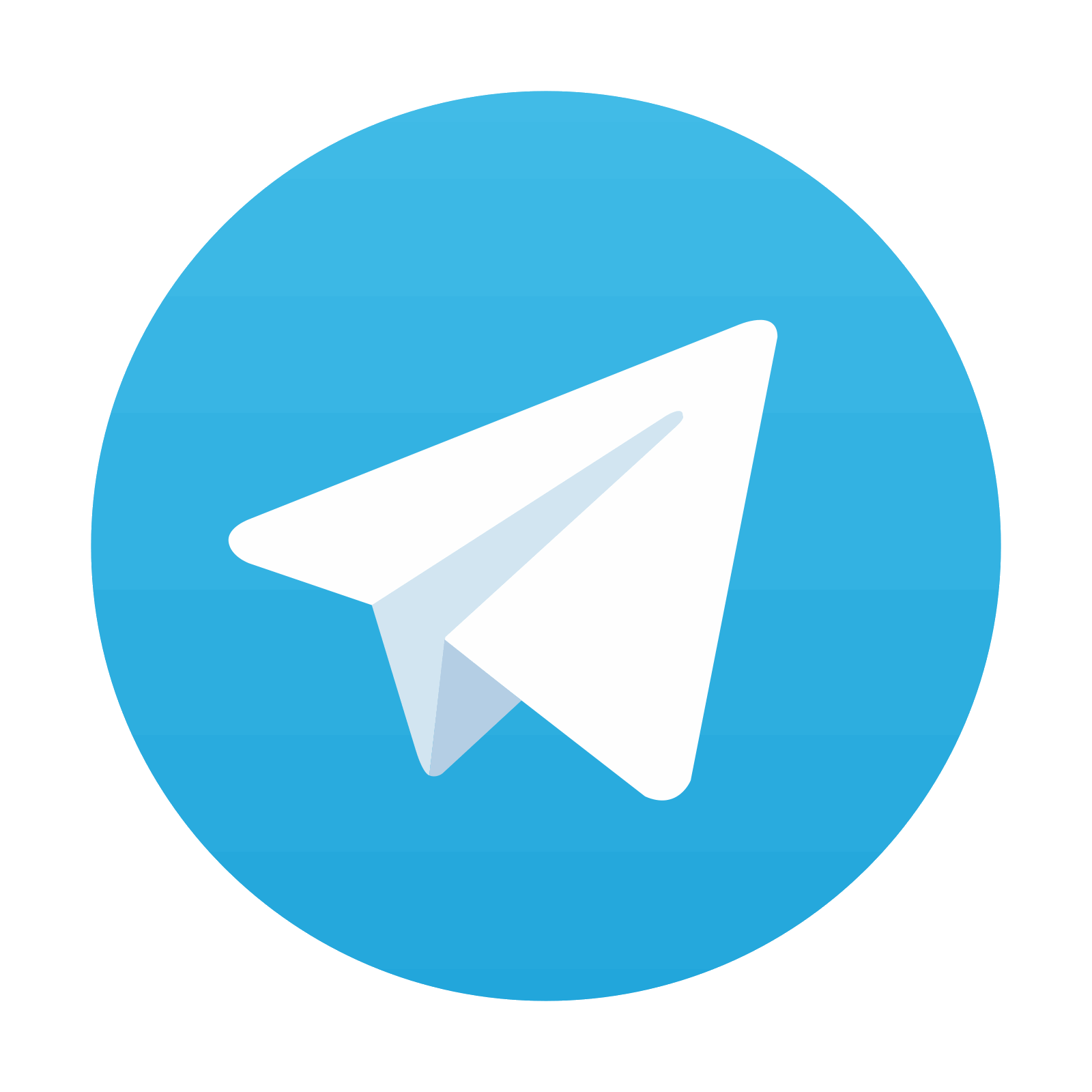
Stay updated, free articles. Join our Telegram channel
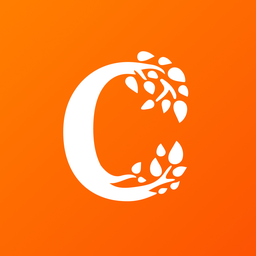
Full access? Get Clinical Tree
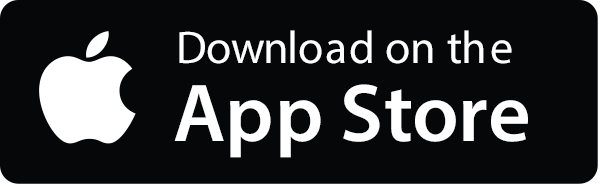
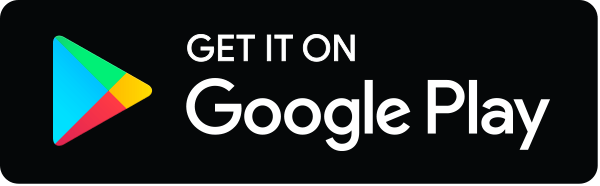