Fig. 11.1
Process involved in the generation of an inhibitor of proteoglycan synthesis for the prevention of atherosclerosis. An inhibitor of growth factor-mediated elongation of glycosaminoglycan chains on proteoglycans, such as biglycan, secreted by vascular smooth muscle cells has the potential to inhibit the matrix lipoprotein interaction which results in the deposition of lipid in the vessel wall as the initiating step in atherosclerosis. Such a product would be used jointly with a statin drug (see text for details)
Biglycan expression in vascular smooth muscle cells is increased by TGF-β, which also markedly increases the size of the GAG chains on the biglycan, and accordingly there is a strong association with biglycan, TGF-β, and lipid as a causal step in the development of atherosclerosis, and this is a therapeutic target [28, 115, 116].
The most investigated area of the potential of therapeutic agents directed at proteoglycan synthesis is the signaling pathways that mediate elongation of CS/DS GAG chains on biglycan because of the direct association with increased lipid binding [26]. Many growth factors and hormones stimulate vascular smooth muscle cells to synthesize and secrete biglycan with increased GAG length [117], a phenomenon that we have termed hyperelongation, because it represents a further elongation of GAG than that seen under basal conditions [26]. Vasoactive factors that stimulate GAG elongation include seven-transmembrane G protein-coupled receptor agonists, such as angiotensin II, endothelin-1, and thrombin; protein tyrosine kinase receptor agonists such as PDGF and epidermal growth factor (EGF); and serine/threonine kinase agonists such as TGF-β (Fig. 11.1) [117]. Taking the serine/threonine kinase receptor for TGF-β, transforming growth factor-β receptor I (TβRI), as an example, there has been considerable progress in characterizing the pathways through which this receptor leads to GAG hyperelongation and in distinguishing these pathways from those that mediate increased expression of the proteoglycan core protein [32, 118]. TGF-β also stimulates a pathway, known as non-Smad, which involves the activation of the MAP kinase pathway leading to stimulation of Erk1/2 and phosphorylation of Smad in the linker region. The linker region is located between receptor Smads Mad homology domains 1 and 2. Although phosphorylation in the linker region was originally shown to inhibit Smad function [119, 120], most recent studies show that linker region phosphorylation is a positive driver of Smad responses [116, 121, 122]. There is a small group of serine and threonine residues in the linker region of Smad transcription factors, and these are linked to specific downstream pathways that regulate gene transcription, including that controlling the expression of genes which mediate GAG elongation [121]. For vasoactive agents which are mitogens, such as PDGF, which also stimulate proteoglycan core protein expression, the core protein expression and mitogenic pathways appear to be common or at least similar, including PI3K and Akt, whereas GAG elongation pathways are mediated via MAP kinases, especially Erk1/2 [32]. The highly specific biochemical targets that have been identified in this work have the potential to be used for the development of drugs to prevent GAG elongation and lipid deposition in atherogenesis.
The potential for inhibiting GAG elongation and preventing lipid deposition and atherosclerosis has been demonstrated in animal models [32, 34]. The antitumor agent imatinib (STI 571, Gleevec, Glivec) was developed for the inhibition of Abl tyrosine kinase in chronic myeloid leukemia, in which this kinase is constitutively activated [123]. Imatinib has been characterized as a tyrosine kinase inhibitor and it inhibits only a small number of kinases. In the vascular context, imatinib inhibits Abl as well as Kit and PDGF receptor kinase [124]. Imatinib is a potent inhibitor of PDGF-stimulated GAG elongation [32, 34]. GAG chains isolated from vascular smooth muscle cells treated with imatinib show reduced binding to normal human LDL, and high-fat-fed atherosclerosis-prone mice treated with imatinib show reduce lipid deposition ex vivo and in vivo [32, 34]. Atherosclerosis is associated with many growth factors and hormones, and intriguingly we have found that imatinib inhibits the GAG elongation action of not only tyrosine kinase agonists but also of seven-transmembrane G protein-coupled receptor and serine/threonine kinase agonists (Little, unpublished observation). These latter agonists have distinct signaling pathways (Fig. 11.1), so identifying the precise target of the action of imatinib might produce a therapeutically useful GAG inhibitor. Such a drug would be used in combination with a HMG CoA reductase inhibitor (statin), such that the statin would reduce circulating levels of atherogenic lipoproteins and the GAG inhibitor would render the vessel wall less sticky, with the overall effect of a reduced rate of progression of atherosclerosis [125].
Hyaluronan as a Therapeutic Target in Atherosclerosis
HA is a potential target for the prevention of atherosclerosis as evidenced by its involvement in the multiple early changes in the vessel wall, both structurally and as an inflammatory component [126]. To date very little progress has been made on specifically targeting components of the ECM, including HA. There are however examples of drugs influencing HA metabolism, such as anti-inflammatory steroids prednisolone, hydrocortisone, dexamethasone, and betamethasone, significantly inhibiting HA synthesis and not sulfated glycosaminoglycan synthesis [127]. The nonsteroidal anti-inflammatory drug etoricoxib, a cyclooxygenase enzyme COX2 inhibitor, blocks HAS2 production of HA [128]. The immunosuppressive drug sirolimus causes a significant reduction in HAS1-3 mRNA levels and hyaluronan synthesis [129], and the statin lovastatin reduces HA accumulation in Watanabe heritable hyperlipidemic rabbits [130]. These examples demonstrate potential beneficial therapeutic effects in the acute inflammatory phase of atherosclerosis where HA production is elevated.
Conclusions
It is very surprising that all of the research over the last two decades has not led to more novel agents for the treatment of atherosclerosis. Nevertheless this chapter on lipid-matrix interactions and other bodies of work focused on the role of inflammation in the development of atherosclerosis [37] clearly demonstrate that there are many preclinical targets which have not been fully explored for their potential as therapeutic targets. Work in these areas has been impeded by the lack of animal models which reproduce the human disease with high fidelity, so new models are urgently required. Although diabetes drives an almost two-fold increase in the rate of development of atherosclerosis and of cardiovascular disease [7], the factors that are specific to diabetes have not been identified [12], and that is an area requiring more research and new insights.
The early pre-inflammatory phase of the development of atherosclerosis occurs due to the interaction of extracellular matrix molecules in the vessel wall with circulating lipid species, which enter the vessel wall and are retained. Although the area of circulating lipids has received considerable attention, the properties of the extracellular matrix of the vessel wall and the interaction of the matrix with circulating lipids which enter the wall has been less appreciated and investigated. Perhaps therein lies the pathway to a new therapeutic agent, to work alone or more likely in combination with a lipid-lowering agent, to retard the development of atherosclerosis and cardiovascular disease. Even in the absence of knowledge of the diabetes-specific factors that accelerate atherosclerosis, such therapies will likely also be beneficial to people with diabetes.
Acknowledgements
Work described in this report was supported by a National Health and Medical Research Council Australia Project Grant (#1022800 Little and Osman) and National Heart Foundation of Australia grant-in-aid (#G10M5211 Little and Osman).
References
1.
Fowler MJ. Microvascular and macrovascular complications of diabetes. Clin Diabetes. 2011;26(2).
2.
Thorp AA, et al. Sedentary behaviors and subsequent health outcomes in adults a systematic review of longitudinal studies, 1996–2011. Am J Prev Med. 2011;41(2):207–15.PubMed
3.
Weyer C, et al. Hypoadiponectinemia in obesity and type 2 diabetes: close association with insulin resistance and hyperinsulinemia. J Clin Endocrinol Metab. 2001;86(5):1930–5.PubMed
4.
Couper JJ, et al. Weight gain in early life predicts risk of islet autoimmunity in children with a first-degree relative with type 1 diabetes. Diabetes Care. 2009;32(1):94–9.PubMed
5.
Reusch JE, Wang CC. Cardiovascular disease in diabetes: where does glucose fit in? J Clin Endocrinol Metab. 2011;96(8):2367–76.PubMed
6.
Stratton IM, et al. Association of glycaemia with macrovascular and microvascular complications of type 2 diabetes (UKPDS 35): prospective observational study. BMJ. 2000;321(7258):405–12.PubMed
7.
Haffner SJ, Cassells H. Hyperglycemia as a cardiovascular risk factor. Am J Med. 2003;115(Suppl 8A):6S–11.PubMed
8.
Roger VL, et al. Heart disease and stroke statistics-2011 update: a report from the American Heart Association. Circulation. 2011;123(4):e18–209.PubMed
9.
Brownlee M. Biochemistry and molecular cell biology of diabetic complications. Nature. 2001; 414(6865):813–20.PubMed
10.
Fruchart JC, Staels B, Duriez P. PPARS, metabolic disease and atherosclerosis. Pharmacol Res. 2001; 44(5):345–52.PubMed
11.
Cooper ME, et al. Mechanisms of diabetic vasculopathy: an overview. Am J Hypertens. 2001;14(5 Pt 1):475–86.PubMed
12.
Preis SR, et al. Trends in cardiovascular disease risk factors in individuals with and without diabetes mellitus in the Framingham Heart Study. Circulation. 2009;120(3):212–20.PubMedCentralPubMed
13.
Hannan KM, et al. Troglitazone stimulates repair of the endothelium and inhibits neointimal formation in denuded rat aorta. Arterioscler Thromb Vasc Biol. 2003;23(5):762–8.PubMed
14.
de Dios ST, et al. Inhibitory activity of clinical thiazolidinedione peroxisome proliferator activating receptor-gamma ligands toward internal mammary artery, radial artery, and saphenous vein smooth muscle cell proliferation. Circulation. 2003;107(20): 2548–50.PubMed
15.
Nissen SE, Wolski K. Effect of rosiglitazone on the risk of myocardial infarction and death from cardiovascular causes. N Engl J Med. 2007;356(24): 2457–71.PubMed
16.
Diamond GA, Bax L, Kaul S. Uncertain effects of rosiglitazone on the risk for myocardial infarction and cardiovascular death. Ann Intern Med. 2007; 147(8):578–81.PubMed
17.
Ghosh RK, et al. SGLT2 inhibitors: a new emerging therapeutic class in the treatment of type 2 diabetes mellitus. J Clin Pharmacol. 2012;52(4):457–63.PubMed
18.
Drucker DJ, Nauck MA. The incretin system: glucagon-like peptide-1 receptor agonists and dipeptidyl peptidase-4 inhibitors in type 2 diabetes. Lancet. 2006;368(9548):1696–705.PubMed
21.
Kolodgie FD, et al. Differential accumulation of proteoglycans and hyaluronan in culprit lesions: insights into plaque erosion. Arterioscler Thromb Vasc Biol. 2002;22(10):1642–8.PubMed
22.
Konstantinov IE, Mejevoi N, Anichkov NM. Nikolai N. Anichkov and his theory of atherosclerosis. Tex Heart Inst J. 2006;33(4):417–23.PubMedCentralPubMed
23.
Williams KJ, Tabas I. The response-to-retention hypothesis of early atherogenesis. Arterioscler Thromb Vasc Biol. 1995;15(5):551–61.PubMedCentralPubMed
24.
Ballinger ML, et al. Regulation of glycosaminoglycan structure and atherogenesis. Cell Mol Life Sci. 2004;61(11):1296–306.PubMed
25.
Tabas I, Williams KJ, Boren J. Subendothelial lipoprotein retention as the initiating process in atherosclerosis: update and therapeutic implications. Circulation. 2007;116(16):1832–44.PubMed
26.
Little PJ, Osman N, O’Brien KD. Hyperelongated biglycan: the surreptitious initiator of atherosclerosis. Curr Opin Lipidol. 2008;19:448–54.PubMed
27.
Finn AV, et al. Pharmacotherapy of coronary atherosclerosis. Expert Opin Pharmacother. 2009;10(10): 1587–603.PubMed
28.
Little PJ, et al. Proteoglycans synthesized by arterial smooth muscle cells in the presence of transforming growth factor-beta1 exhibit increased binding to LDLs. Arterioscler Thromb Vasc Biol. 2002;22(1): 55–60.PubMed
29.
Gustafsson M, Boren J. Mechanism of lipoprotein retention by the extracellular matrix. Curr Opin Lipidol. 2004;15(5):505–14.PubMed
30.
Hashimura K, et al. Androgens stimulate human vascular smooth muscle cell proteoglycan biosynthesis and increase lipoprotein binding. Endocrinology. 2005;146(4):2085–90.PubMed
31.
Ivey ME, Osman N, Little PJ. Endothelin-1 signalling in vascular smooth muscle: pathways controlling cellular functions associated with atherosclerosis. Atherosclerosis. 2008;199(2):237–47.PubMed
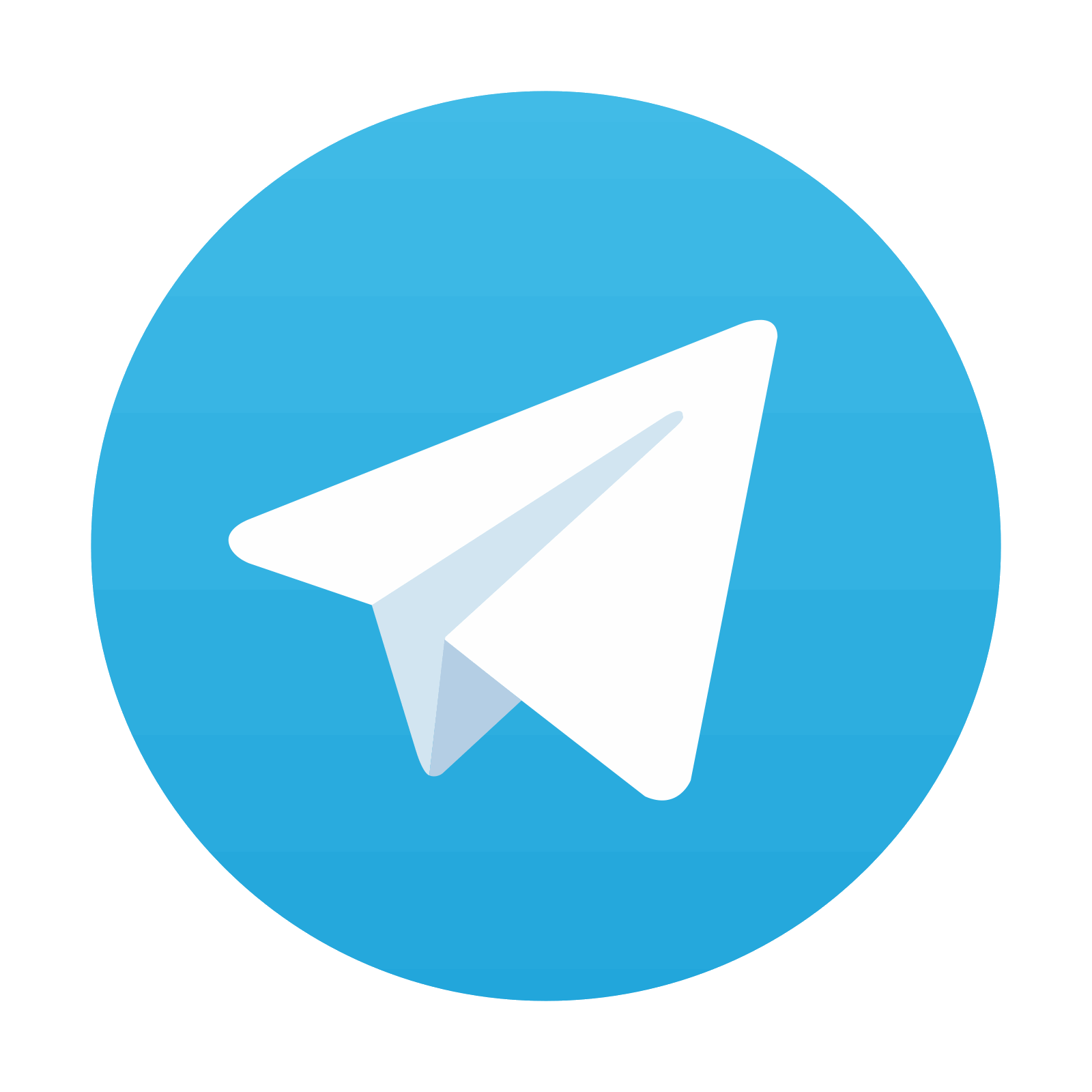
Stay updated, free articles. Join our Telegram channel
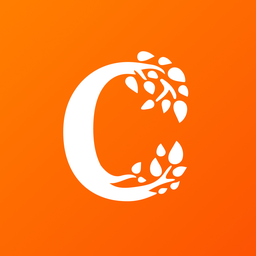
Full access? Get Clinical Tree
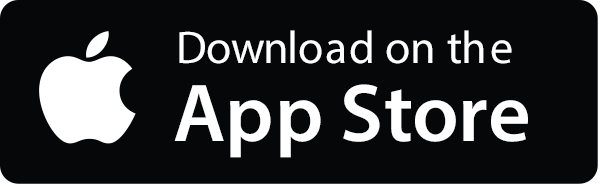
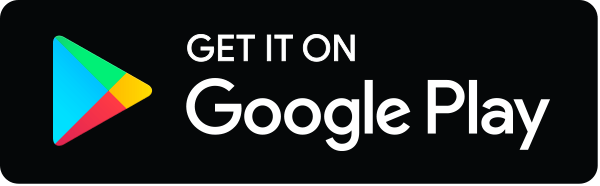