Image-Guided Radiation Therapy
Image-guided radiation therapy (IGRT) consists of a panoply of technological applications with the common purpose of maximizing target and normal tissue localization for radiotherapy. The exact subset of applications that defines IGRT is somewhat controversial. Proposed definitions of IGRT have ranged from narrow (“external beam radiation therapy with positional verification using imaging prior to each treatment fraction”1) to broad (“any use of imaging to aid in decisions in the radiotherapy process”2), the former risking the exclusion of techniques as avant garde as adaptive four-dimensional (4D) positron emission tomography (PET), and the latter risking the inclusion of techniques as banal as a staging chest x-ray. The American College of Radiology and American Society of Radiation Oncology practice guideline defines IGRT as “a procedure that refines the delivery of therapeutic radiation by applying image-based target relocalization to allow proper patient repositioning for the purpose of ensuring accurate treatment and minimizing the volume of normal tissue exposed to ionizing radiation,”3 while Greco and Ling4 defined IGRT more broadly as “the use of imaging for detection and diagnosis, delineation of target and organs at risk (OARs), determining biological attributes, dose distribution design, dose delivery assurance, and deciphering treatment response,” a so-called six-dimensional definition. For the purposes of this chapter, the authors also favor a broader perspective and define IGRT as the use of innovative imaging modalities to augment target and normal tissue localization for radiotherapy planning and delivery. This encompasses a wide range of imaging techniques used for delineation, adjusting for motion or positional uncertainty, and adapting treatment to response. Exploring IGRT in its many facets leaves one simultaneously awed by the pace and extent of technological achievements, yet daunted by the task of critically assessing their tangible benefits to patients.
RATIONALE FOR IMAGE-GUIDED RADIATION THERAPY
Increasing the accuracy and precision of radiotherapy delivery has always been a therapeutic goal. Inaccuracy refers to systematic errors that, on average, bias the treatment delivery with respect to the true target location. Systematic errors can originate, for example, from improper target delineation, poorly representative simulation, dissociation between skin marks and internal anatomy, or predictable organ motions (e.g., periodicity of a lung tumor). Imprecision, on the other hand, refers to stochastic (random) errors that introduce variance in the spatial location of treatment around the true target. Stochastic errors can originate, for example, from inevitable fluctuations in daily setup and from unpredictable target motions (e.g., uterine anteversion or retroversion). Insufficient compensation for these uncertainties leads to target underdosing and overdosing of nearby OARs, whereas overcompensation for uncertainties leads to unnecessary irradiation of normal tissue and constraints in treatment planning. This creates a tradeoff between tumor control probability (TCP) and normal tissue complication probability (NTCP) and emphasizes the role of minimizing uncertainties to enhance the therapeutic ratio of radiation.
Uncertainty in target delineation is a well-documented problem.5,6 Even among experts, reproducibly defining targets is a challenge, as both intra- and interobserver variation contribute to ambiguity in target localization, and existing guidelines for target delineation are predominantly based on qualitative judgments. Furthermore, while computed tomography (CT) and magnetic resonance imaging (MRI) have become standard for 3D planning, functional imaging techniques—particularly PET—have been increasingly incorporated into treatment planning7 to facilitate demarcation of tumor borders and characterize subregions of targets with different physiologic properties. Quantitative imaging can help raise consistency in target delineation, while automated segmentation and deformable image registration software are becoming increasingly available to facilitate and standardize treatment planning.
The use of conformal and hypofractionated radiotherapy techniques, with prolonged treatment times and steeper dose gradients, accentuates the effects of uncertainties related to target localization and the need for IGRT to compensate for them. Toxicity is often an important barrier to treatment intensification, including radiation dose escalation and intensive combined modality therapy. By mitigating toxicity, IGRT may permit implementation of more intensive, but isotoxic, treatment approaches. Furthermore, as therapies continue to improve tumor control, the importance of reducing late and chronic effects of radiotherapy becomes increasingly imperative to maximize patients’ quality of life. Determining the functional relation between IGRT, changes in tumor and OAR dose, and changes in clinical outcomes (e.g., TCP and NTCP models) are of critical importance in evaluating the effectiveness of IGRT techniques compared to standard approaches. There is a rapidly growing need for validated models to estimate the impact of IGRT on cumulative dose distributions and the corresponding effects of cumulative dose on TCP and NTCP.
Conventional radiotherapy techniques are limited due to motion and changes of both tumor and normal tissues occurring between (interfraction) or during (intrafraction) treatment. In many situations, the treatment model based on a static initial simulation is inadequate, necessitating adaptation of the initial plan. In particular, changes that occur in response to therapy could be indicative of a more or less favorable prognosis, in which case modifications to the treatment strategy could be considered. Theoretically, adaptive radiotherapy can take place either between fractions (offline) or while the patient is in the treatment position (online). Innovative strategies to monitor and optimize therapy throughout the treatment course, such as 4D PET-CT, in-room MRI, and fast online adaptive replanning, ideally will advance the quality of radiotherapy for current and future generations.
IMAGE-GUIDED TARGET AND NORMAL TISSUE DELINEATION
Positron Emission Tomography
PET has revolutionized the staging and treatment of cancer. PET scanning involves the systemic administration of a tracer labeled with a radioactive isotope, which emits positrons as it decays. The tracer accumulates in a region of interest and the emitted positron annihilates with a local negatron, releasing two 511 keV photons that propagate in 180 degrees opposite directions. The scanner is equipped with parallel mounted sensors that can detect and determine the spatial location of these annihilation events and, therefore, the regions of increased radiotracer accumulation. Most modern treatment planning systems offer tools to facilitate image registration and fusion with the planning CT to aid target delineation. Commercial software systems that incorporate deformable image registration can aid delineation by accommodating changes in patient anatomy and positioning between scans and segmenting target volumes based on quantitative methods (Fig. 11.1).6,8
FIGURE 11.1. Reductions in contour variability are observed with automatic contouring. Physician manual contours shown in blue, automatic contours modified by physicians shown in purple, and manual contours using Simultaneous Truth and Performance Level Estimation algorithm shown in brown. (From Stapleford LJ, Lawson JD, Perkins C, et al. Evaluation of automatic atlas-based lymph node segmentation for head-and-neck cancer. Int J Radiat Oncol Biol Phys 2010;77:959–966, with permission from Elsevier.)
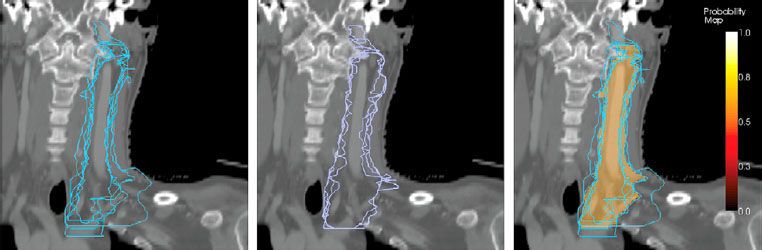
FIGURE 11.2. Fluorodeoxyglucose (FDG) positron emission tomography–computed tomography (PET-CT) scan in a patient with a right middle lobe lung carcinoma. On CT[MB15] (left), the limits of the tumor are obscured by postobstructive pneumonia and atelectasis. On the fused scan (right), the tumor is intensely FDG avid and more easily differentiated. (From Spratt DE, Diaz R, McElmurray J, et al. Impact of FDG PET/CT on delineation of the gross tumor volume for radiation planning in non-small-cell lung cancer. Clin Nucl Med 2010;35:237-243, with permission.)
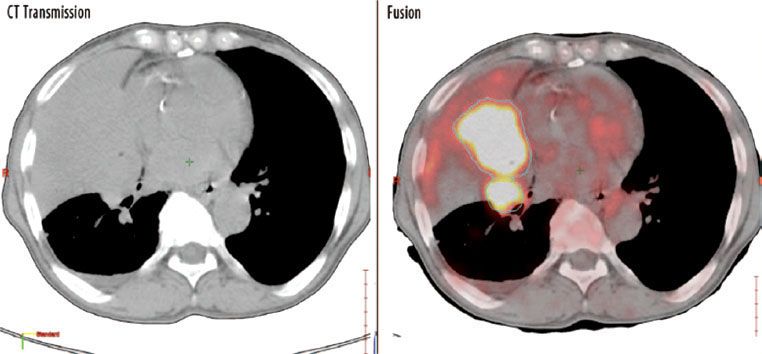
F18-Fluorodeoxyglucose
The most widely used tracer is F18-fluorodeoxyglucose (18F-FDG), which is taken into cells by active transport, then phosphorylated by hexokinase, trapping the molecule intracellularly. 18F decays to 18O, and the molecule enters the glycolytic pathway, but the metabolism of FDG is slow relative to normal glucose, accounting for the high relative accumulation in metabolically active cells, including inflammatory tissue, neurons, brown fat, bone marrow, gastrointestinal (GI) epithelium, and tumors.9 Studies in many different types of cancer have found that FDG-PET improves staging,10–11,12,13,14 leading to more appropriate risk-adapted management. Other studies have found that increased FDG uptake within tumors prior to or following treatment confers an adverse prognosis,15,16,17 indicating subsets of patients suitable for alternative treatment strategies.
Multiple studies have investigated the role of FDG-PET in radiotherapy planning for lung cancer.18–23 FDG-PET imaging alters treatment volumes in approximately 40% to 60% of non–small cell lung cancer (NSCLC) patients19,21,23,24; it appears to aid targeting for mesothelioma as well.25 It is valuable both for detection of occult nodal involvement and for distinguishing tumor from atelectasis,19,26 which can be difficult to detect on CT alone (Fig. 11.2). Vanuytsel et al.21 found that FDG PET-CT altered treatment volumes in 45 of 73 (62%) lymph node–positive patients staged by mediastinoscopy; in 16 the volume was enlarged, and in 29 it was contracted. Results of the Radiation Therapy Oncology Group’s (RTOG) study RTOG-0515, a phase II trial with 52 NSCLC patients, were recently reported22; incorporation of FDG PET-CT led to alterations in nodal volumes in 51% of the 47 evaluable patients and in general led to smaller tumor gross tumor volumes (GTV) and mean lung dose. There is no definite consensus on how PET-guided volumes should be delineated, although PET does appear to improve interobserver target definitions.18 Yu et al.24 reported that the optimal standardized uptake value (SUV) threshold correlating with pathologic specimens was 31% ± 11%. However, recent studies in both phantoms and patients indicate that gradient-based methods may be a more accurate and consistent technique for target volume contouring.8,27
Studies of FDG PET in head and neck cancer (HNC) have similarly found that planning volumes are frequently altered after incorporating PET imaging.23,28–35 In a study of 40 HNC patients, Paulino et al.30 compared IMRT plans based on FDG PET to those based on CT. In 25% of patients, CT-based plans were suboptimal in covering the PET-delineated GTV. In a prospective analysis of 20 patients, Schwartz et al.32 found that IMRT plans could be optimized with FDG PET-CT to improve parotid and laryngeal sparing and allow dose escalation up to 81 Gy. A study from Memorial Sloan-Kettering Cancer Center found significant differences in target volumes drawn with and without PET or MRI guidance, indicating complementary information comes from multiple sources, including CT and physical examination, and is necessary to optimally tailor target delineation for each patient.33 GTVs delineated by FDG PET appear to be significantly smaller than those delineated on CT alone.33–35 Some concerns exist regarding the technical aspects of PET-guided radiation therapy (RT) in HNC, including difficulties in establishing optimal image registration36 and large variability in target definition.37 Recent studies indicate that automated techniques to guide delineation in HNC can improve consistency,6,38,39 however, target delineation is still highly dependent on both segmentation and reconstruction methods, emphasizing the importance of clarifying and standardizing methodologies across institutions.40,41
FDG PET for target delineation has been extensively studied in both esophageal14,42,43–47 and rectal48,49–52 cancers. Hong et al.44 studied 25 esophageal cancer patients undergoing FDG PET-CT for radiotherapy planning; PET influenced target delineation in 21 patients (84%), with changes classified as major in 9 (34%). However, Muijs et al.14 reviewed 30 studies spanning 1,222 patients and found no conclusive evidence supporting the necessity of PET for radiotherapy planning. It is clear that PET is helpful in determining lymph node status and detecting occult metastases, but whether it is superior to other modalities for GTV delineation remains unclear. If used to delineate GTV, a threshold of 2.5 for either absolute SUV or SUV relative to liver uptake has been proposed.47 As for rectal cancer, Braendengen et al.48 compared GTV delineation with MRI versus FDG PET-CT in 77 patients; PET-guided volumes were smaller than MRI volumes, but PET-guidance appeared to complement data from MRI and led to alteration of management in 15% of patients. Dynamic FDG PET-CT is also an emerging technique50 that could play a role in the future for image-guided target delineation for rectal and other cancers.
Researchers at Washington University have extensively studied FDG PET for cervical cancer.53–55 PET-guided targeting for cervical cancer patients with involved para-aortic lymph nodes can facilitate safe dose escalation to 60 Gy along with intensity-modulated radiation therapy (IMRT).53 Serial changes in cervical tumor volume during brachytherapy54 and external beam RT55 have been documented, but it is unclear yet how treatment should be adapted in the face of poor response. Lin et al.56 reported that FDG PET-based brachytherapy planning significantly optimized GTV coverage without increasing bladder or rectal dose. Liang et al.57 analyzed 10 patients treated with FDG PET-guided bone marrow–sparing IMRT for pelvic malignancies in a prospective trial. IMRT plans significantly reduced dose to active bone marrow, and this approach was feasible and well tolerated. Related work by Rose et al.58 has indicated that dose to metabolically active bone marrow subregions identified by FDG PET is a significant predictor of hematologic toxicity.
Studies of FDG PET for radiation planning in other disease sites have found mixed results. It has potential utility in contouring lumpectomy cavities in breast cancer,59 involved nodal or involved field radiation therapy for lymphoma60,61 and GTV for pancreatic cancer,62 but less apparent utility in treating sarcoma.63 Application of FDG PET in central nervous system (CNS) tumors is limited by high background uptake of FDG by normal brain cells, whereas its utility in prostate cancer is limited by relatively lower uptake of FDG in tumor cells. Douglas et al.64 successfully used FDG PET in 40 patients for dose escalation in malignant glioma, however, no improvement in patient outcomes was observed. As discussed below, other PET tracers have been more extensively studied in these diseases.
FIGURE 11.3. Comparison of magnetic resonance imaging, L-(methyl-11C)methionine positron emission tomography (PET_, and O-(2-[18F]fluoroethyl)-L-tyrosine PET for a patient with grade III astrocytoma. (From Grosu AL, Astner ST, Riedel E, et al. An interindividual comparison of O-(2-[18F]fluoroethyl)-L-tyrosine (FET)- and L-[methyl-11C]methionine (MET)-PET in patients with brain gliomas and metastases. Int J Radiat Oncol Biol Phys 2011;81:1049–1058, with permission from Elsevier.)
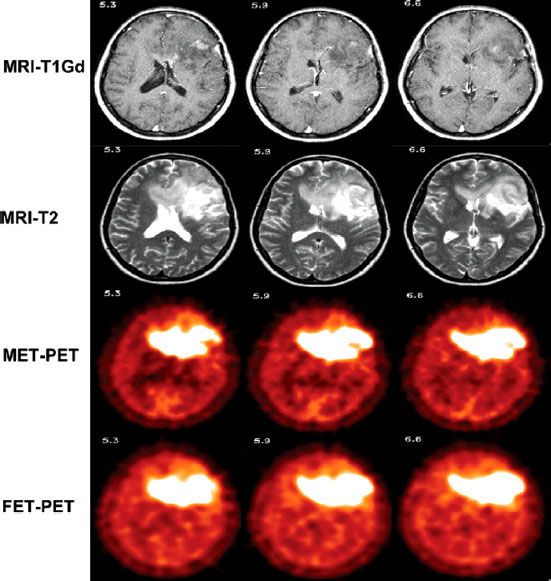
Other Positron Emission Tomography Tracers
Although most studies to date have focused on FDG, many other radiotracers have been studied, including 18F-thymidine (FLT), 18F-misonidazole (MISO), 18F-azomycin arabinoside (FAZA), 18F-fluoroethyl-L-tyrosine (FET), 18F-choline, 11C-choline, 11C-methionine (MET), 11C-acetate, and 60Cu(II)-diacetyl-bis(N4-methylosemicarbazone) (60Cu-ATSM). 15O and 13N—labeled H2O, CO2, O2, or NH3—molecules have also been used to measure blood flow, apoptosis, or hypoxia with PET.65–67 New tracers are continually being developed and tested. For further discussion of novel molecular imaging applications, refer to several reviews.65,66–68,69
Background uptake of MET in neural tissue is low, making it useful for image-guided planning of brain tumors. Grosu et al.70 analyzed 39 patients with glioblastoma multiforme (GBM); in 29 patients (74%), MET uptake extended (up to 4.5 cm) beyond the tumor identified by MRI. MET also appears to improve GTV delineation for skull-base meningiomas.71 A limitation of MET PET, however, is the short half-life of 11C (20 minutes). FET leads to different GTV compared to MRI alone72 but appears to be comparable to MET (Fig. 11.3),73 with the advantage of a longer isotope half-life. Milker-Zabel et al.74 evaluated 68Ga-(0)-D-Phe (1)-Tyr (221)-octreotide (DOTATOC) PET in 26 meningiomas patients. This technique takes advantage of high expression of the somatostatin type 2 receptor, which binds DOTATOC. In 19 patients, DOTATOC PET significantly influenced target design. Gehler et al.75 found similar results, with DOTATOC PET-CT significantly influencing target volumes in 17 of 26 patients.
Several investigators have explored PET-guided RT using MISO76,77 and FAZA.78 The Trans-Tasman Radiation Oncology Group correlated hypoxia identified on MISO PET with outcomes in 45 stage III or IV HNC patients undergoing chemoradiotherapy, with or without the hypoxic cytotoxin tirapazamine.77 Baseline hypoxia and residual hypoxia (detected on MISO PET scans at week 4 or 5 of treatment) were correlated with higher rates of locoregional failure. Four of six patients with residual hypoxia recurred locally compared to 4 of 23 patients without residual hypoxia. 60Cu-ATSM has attracted attention for hypoxia imaging due to its potential biokinetic advantages and better resolution. 60Cu-ATSM PET-guided hypoxia imaging has been investigated in HNC and cervical cancer.79,80 In a pilot study in 14 cervical cancer patients, 60Cu-ATSM appeared to provide good prognostic discrimination; 5 of 5 patients with hypoxic tumors developed recurrence versus 3 of 9 with normoxic tumors.80
Both 11C-choline and 18F-choline have been studied in prostate cancer81,82; however, the utility of this approach in routine settings is unclear. SUV at 60% of the maximum value appears to correlate well with histopathologic specimens as a threshold for contouring dominant intraprostatic lesions.81 FLT PET has shown utility in some settings, such as esophageal cancer, where its positive predictive value for involved nodes may be higher than for FDG PET.51 In a study of five NSCLC patients undergoing serial baseline and on-treatment FLT PET, reductions in FLT uptake within both tumor and bone marrow were observed.83 However, its value for tumor and nodal delineation in rectal cancer and HNC appears more limited.84,85
In summary, a wide body of literature supports the utility of PET for image-guided treatment planning. Further research efforts are needed to standardize approaches and determine the impact of PET-guided planning on patient outcomes.
Magnetic Resonance Imaging
The utility of MRI in RT planning, particularly for CNS, HNC, and pelvic malignancies, is well known.86–94 In addition, MR simulators and MRI-only planning approaches are becoming more widely available (Fig. 11.4).95 Increasingly, quantitative MRI techniques have been used to improve RT planning. For example, functional MRI (fMRI) has been used to reduce radiation dose to normal functioning brain during planning for CNS tumors.96–100 Aoyama et al.99 evaluated the use of magnetoencephalography and anisotropic diffusion weighted MRI to plan 20 patients, 15 of whom had arteriovenous malformation (AVM). In 15 patients, targets were modified with significant reduction in the volume of sensitive regions receiving more than 15 Gy. Fast imaging employing steady-state acquisition can facilitate visualization of the trigeminal nerve during radiosurgery planning.101,102 The 1H MR spectroscopy (MRS) has also been used to guide planning in gliomas.103,104 Underdosing of 1H MRS-delineated metabolically active areas has been associated with worse outcomes in GBM.104
In patients with prostate cancer, van Lin et al.105 have reported the feasibility of escalating doses to 90 Gy to dominant intraprostatic lesions identified by 1H MRS. MR lymphography with intravenous ferumoxtran-10 has also been used to identify pathologic nodal involvement in prostate cancer (Fig. 11.5).106,107 In a study of 47 patients treated with salvage RT for rising postprostatectomy prostate-specific antigen (PSA), 79% were found to have at least one aberrant positive lymph node, including 10 of 18 (61%) with a PSA less than 1.0 ng/mL. MR lymphography may therefore be useful in helping to define nodal boost volumes in prostate cancer.
FIGURE 11.4. Integration of magnetic resonance (MR) imaging and radiotherapy, with trolley solution and specialized docking device for smooth transfer between MR and linear accelerator. (From Karlsson M, Karlsson MG, Nyholm T, et al. Dedicated magnetic resonance imaging in the radiotherapy clinic. Int J Radiat Oncol Biol Phys 2009;74:644–651, with permission from Elsevier.)
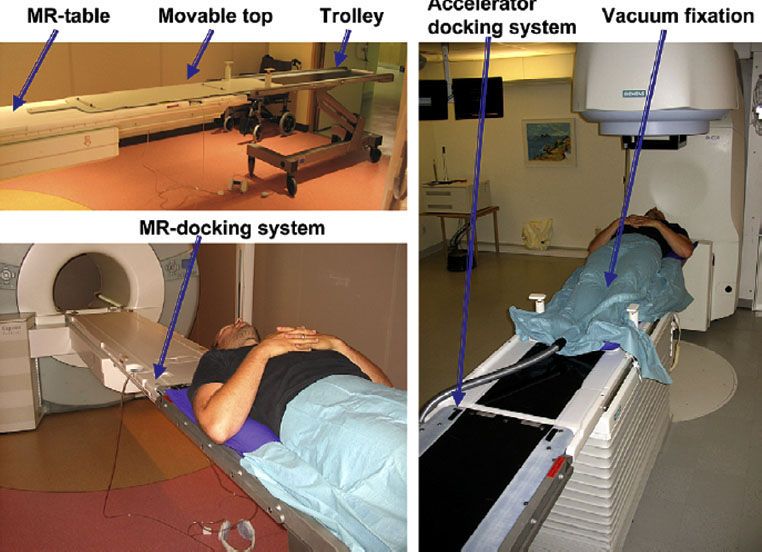
FIGURE 11.5. Fusion of magnetic resonance (MR) lymphography (upper right and left and lower left) and computed tomography (CT) (lower right). With the help of MR lymphography, the node identified on CT is identified as pathologic. (From Meijer HJ, van Lin EN, Debats OA, et al. High occurrence of aberrant lymph node spread on magnetic resonance lymphography in prostate cancer patients with a biochemical recurrence after radical prostatectomy. Int J Radiat Oncol Biol Phys 2012;82(4):1405–1410; with permission from Elsevier.)
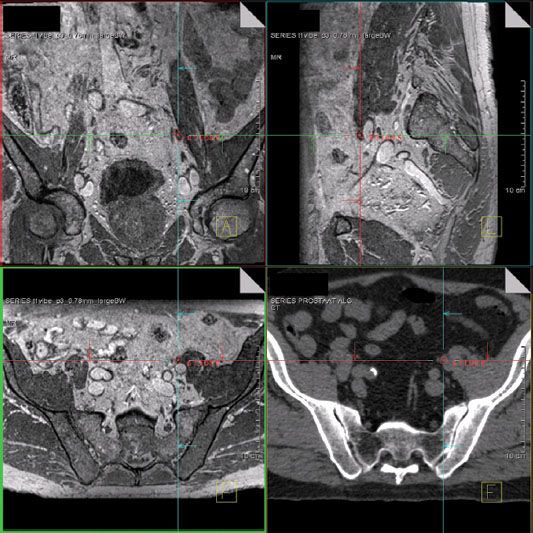
FIGURE 11.6. Axial iterative decomposition of water and fat with echo asymmetry and least-squares estimation magnetic resonance imaging scans of the pelvis in a gynecologic cancer patient undergoing chemoradiotherapy. Scans were acquired at baseline (left), midtreatment (middle), and posttreatment (right) and show a steady increase in fraction of fat relative to water within the pelvic bones, indicated by conversion to progressively higher signal.
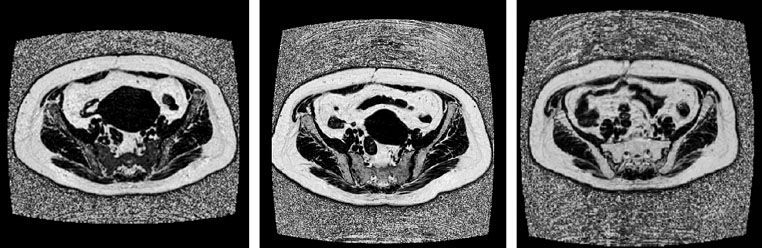
Dynamic contrast-enhanced (DCE) MRI has been investigated for RT planning in a variety of tumors including HNC, lung, rectal, and cervical cancers.108–110,111 Mayr et al.111 studied 102 cervical cancer patients treated with DCE MRI. Patients with a low total volume of tumor voxels with low DCE signal had significantly worse tumor control and disease-specific survival. Liang et al.112 used an MRI technique called iterative decomposition of water and fat with echo asymmetry and least-squares estimation to study fractional changes in fat content of pelvic bone marrow during pelvic chemoradiotherapy (Fig. 11.6). Conversion of bone marrow from low fat, high cellularity to high fat, low cellularity during RT is readily observed, enabling noninvasive quantitative methods to analyze the impact of local changes in radiation dose.
In summary, novel and quantitative or functional MRI techniques have been increasingly implemented for RT planning. Ongoing research is seeking to define the clinical benefits of MRI-based IGRT techniques.
Single Photon Emission Computed Tomography
Single photon emission computed tomography (SPECT) is a relatively inexpensive functional imaging technique, with a wide range of potential tracers. Although PET is generally more quantitatively accurate than SPECT in determining in vivo radioactivity distribution,69 SPECT tracers often have a longer half-lives and release less energy, leading to favorable dosimetry and utility for studying slower biological processes.69 Nonetheless, SPECT appears to have more limited utilization than PET in RT planning.7
Several studies of 111In-capromab pendetide radioimmunoscintigraphy (RIS) have found it useful in planning both external beam RT113,114–115 and brachytherapy116,117 for prostate cancer. Jani et al.114 reported that RIS influenced RT volumes and decision making in a significant proportion of patients undergoing postprostatectomy salvage RT. Of 54 evaluable patients, 18.5% had treatment plans altered by RIS, including 4 who were not offered RT based on the RIS findings. In a multivariate analysis of 107 patients (53 planned with RIS), RIS was associated with an improved 3-year biochemical failure-free survival (bFFS).115 A similar analysis of 82 patients undergoing RIS for salvage therapy, however, did not reveal a clear benefit of RIS.118 Ellis et al.116 treated 80 low-intermediate risk prostate cancer patients with RIS-assisted brachytherapy. Regions of the prostate showing increased RIS uptake were prescribed 150% of the standard dose. The overall 4-year biochemical failure-free survival was 97.4%.
Other applications of SPECT-guided treatment planning have been studied, including 123IMT (123I-alpha-methyl-L-tyrosine) SPECT for gliomas119–121 and meta-123iodo-benzylguanidine scans for neuroblastoma.122 Krengli et al.121 studied 21 patients with high-grade gliomas using fused 99mTc-MIBI SPECT and MRI. Similar to findings of Grosu et al.,119 target volumes were significantly augmented by SPECT, with an average increase of 33% over MRI alone, particularly in resected cases.
SPECT has also been used to guide normal tissue avoidance. In patients with NSCLC, Christian et al.123 used 99mTc SPECT to identify functional lung to avoid using inverse RT planning, and showed the V20 of functioning lung could be reduced without compromising target coverage. Roeske et al.124 used 99mTc SPECT to identify active bone marrow subregions to reduce hematologic toxicity in patients receiving pelvic chemoradiotherapy.
IN-ROOM IMAGE-GUIDED RADIOTHERAPY TECHNIQUES
Numerous studies have found that motion and setup errors for various disease sites can be quite substantial,125 leading to inaccurate or suboptimal treatment plans and potentially poorer tumor control.126–129,130 For example, in a study of 127 prostate cancer patients treated without daily prostate localization, de Crevoisier et al.130 found that significant rectal distension resulting in anterior displacement of the prostate at simulation was an independent risk factor for biochemical failure. Strategies to address motion have included wide margins, elaborate immobilization techniques, resimulation and replanning, and portal radiography. IGRT approaches take advantage of more frequent and sophisticated imaging to setup the patient and localize the target with greater accuracy, ostensibly improving treatment delivery and allowing reduction of margins.
This section will describe various IGRT technologies developed to address both interfraction and intrafraction motion. Particular attention is devoted to clinical applications of in-room IGRT technologies and data supporting their use. Although some of these technologies have been available for many years, others have only recently been introduced, yet appear to have been adopted rapidly by clinicians.131 Many others are still under development and have not yet been implemented clinically.
Ultrasound
Ultrasound (US) is one of the most common IGRT approaches in practice, particularly for prostate cancer.131 It involves emission of high-frequency sound waves to produce images of internal anatomy, consisting of a transducer encased in a probe applied to the skin surface, reflecting sound waves back as echoes when a change in impedance is encountered due to density differences between tissues. The time an echo takes to return is used to calculate the depth of the tissue interface. Image information is obtained along the beamline of the probe, with a complete image created by sweeping across the region of interest. Although three operational modes are available, B (brightness) mode is the primary one used. Readers interested in a more complete description are referred elsewhere.132
Several US products are currently available. All have a system to map the image coordinate system to both the linear accelerator (LINAC) coordinate system and the simulation images. This can be achieved either by tracking the position of a stereotactic arm or using an infrared imaging system to detect the probe position. The target location can be determined in the room prior to treatment, with the necessary shifts conducted to bring the anatomy into position. A widely used US system is the B-mode acquisition and targeting (BAT) transabdominal system (NOMOS, North American Scientific, Chatsworth, CA). The probe is registered to a stereotactic arm on the LINAC gantry, allowing its position to be tracked. Prior to treatment, transverse and sagittal images are generated and the target and normal tissue contours from the planning CT scan are overlaid on the US images. If the target is displaced, the CT structures are maneuvered on a touch screen and the necessary 3D couch shifts are calculated. Another system is SonArray (Varian Medical Systems, Palo Alto, CA), which combines US localization with an optical guidance system to track the position of the probe in the treatment room.133,134 A similar system is available from BrainLab (Heimstetten, Germany). The I-Beam system (Computerized Medical Systems Inc, St. Louis, MO) uses a machine vision pattern recognition technique to calibrate the probe relative to the gantry. Clarity (Elekta, Stockholm, Sweden) incorporates structure-based tissue matching and segmentation tools to facilitate contouring.
At experienced centers, the additional time required to implement US-based IGRT is reported to be 5 minutes or less.135,136 Additional time may be necessary, however, when the technology is first adopted or if moves need to be checked online by a physician. Increased skin-to-prostate distances, increased thickness of tissue anterior to the bladder, and less prostate gland present superior to the symphysis can reduce image quality.137 However, reproducibility and image quality are generally reported to be high for prostate localization.137–139 Probe-induced prostate motion is also a consideration, as displacements up to 1 cm have been observed,140 although generally the magnitude of displacements is 3 mm or less.133,135,138,140,141
Numerous investigators have compared US systems versus conventional setup techniques (i.e., external skin markers) for prostate localization.133–135,136,137,138,139,140,141–144,145 In a review of nine series, Kuban et al.145 reported that shifts from the initial setup were greatest in the anterior-posterior (AP) direction, with standard deviations in the AP, superior-inferior (SI), and right-left (RL) directions ranged from 2.7 to 6.4 mm, 2.8 to 7.3 mm, and 2.1 to 4.6 mm, respectively, with maximum values of 29.8, 30.3, and 34.9 mm, respectively. Several investigators have evaluated shifts in prostate patients undergoing daily portal imaging,138,140 removing the impact of patient setup uncertainty. In a study of 35 patients using BAT, Little et al.140 reported mean shifts of –1.3, –1.6, and –0.89 mm in the AP, SI, and RL directions, respectively. Trichter and Ennis138 reported that the margins necessary to encompass the prostate at the 95% confidence level using daily portal imaging alone (without US) were 9.2, 14.6, and 10.2 mm in the RL, SI, and AP directions, primarily due to organ motion rather than setup error.
Although US-based localization accounts for interfraction organ motion, it does not address intrafraction motion. However, the magnitude of such motion in patients with prostate cancer appears to be small. In a study of 20 patients undergoing pre- and posttherapy US, Huang et al.146 noted mean shifts of 0.2 ± 1.3 mm, 0.1 ± 1 mm, and 0.01 ± 0.4 mm, in the AP, SI, and RL directions, respectively. Trichter and Ennis138 similarly noted small mean intrafraction shifts using pre- and posttherapy US; however, large maximum shifts of 8.1, 20.4, and 8.3 mm in the AP, SI, and RL directions, respectively, were noted.
Several authors have compared prostate localization with CT136,145,147,148 and implanted fiducial markers (144,149,150). Lattanzi et al.136 found average disagreements between the modalities were small: –0.09 mm (AP), –0.03 mm (SI), and –0.16 mm (RL). O’Daniel et al.148 compared four target alignment techniques: skin marks, bony registration, US, and in-room CT. Direct alignment with US and CT provided better target coverage compared to the other methods. Scarbrough et al.150 compared US with fiducial markers in 40 patients and found that US was associated with significantly greater systematic and random errors than fiducials. Similarly, Gayou and Miften151 found that US was associated with a higher percentage of shifts greater than 5 mm compared to cone beam CT (CBCT).
Limited data exist regarding the impact of US IGRT on patient outcomes. Indirect support is garnered, however, from the excellent outcome of prostate cancer patients treated using daily US guidance.152,153–154 Kupelian et al.152 reported on 100 patients undergoing short-course IMRT, using daily BAT. Margins around the target were 4 mm posteriorly, 8 mm laterally, and 5 mm in other directions. With a median follow-up of 66 months, the 5-year bFFS was 85%, with 5% of patients developing grade 2 or 3 rectal sequelae. Similarly, Zerini et al.153 treated 25 low- to intermediate-risk patients to 70 Gy in 30 fractions with daily BAT. With a mean follow-up of 45 months, one patient had biochemical relapse and no patients developed grade 3 or higher late rectal toxicity.
Jani et al.155 evaluated acute toxicity in patients treated with (n = 50) versus without (n = 49) daily BAT, reporting that patients treated using BAT experienced less rectal toxicity. They also separately analyzed late sequelae in patients treated with and without BAT.156 Although less toxicity was observed in patients treated with BAT, there was no significant correlation between BAT usage and toxicity on multivariate analysis. Bohrer et al.157 also reported that patients treated using BAT had less rectal toxicity compared to patients treated prior to BAT implementation. However, no differences in bladder toxicity or PSA control were seen between the two groups. US may also be useful in the postprostatectomy setting.158,159 Chinnaiyan et al.159 evaluated SonArray in six post-prostatectomy patients. The average shifts from the initial setup were 5 ± 4 mm, 3 ± 4 mm, and 3 ± 3 mm, in the AP, SI, and RL directions, respectively.
Fewer studies have reported on the utility of US in other tumor sites. US can be useful to confirm bladder volume and position in gynecologic patients undergoing pelvic RT, which is known to be volatile.160 In intracavitary brachytherapy planning, US is a valuable tool for both detection and prevention of perforations.161,162 Several investigators have also recently evaluated US-based IGRT to define and verify position of the lumpectomy boost cavity in breast cancer.163,164 Boda-Heggeman et al.165 used US guidance for frameless stereotactic radiosurgery (SRS) for liver metastases, using active breathing control to reduce tumor motion. Fuss et al.166 evaluated US-based IGRT in 62 patients with upper abdominal malignancies, predominantly pancreatic cancer. The mean shifts in the AP, SI, and RL directions were 6 ± 5.31 mm, 6 ± 6.7 mm, and 4.9 ± 4.35 mm, respectively. Meeks et al.167 performed US-guided extracranial SRS in 16 patients. Single-fraction doses ranging from 12.5 to 24.0 Gy were delivered without significant acute complications. US-guided extracranial SRS appears safe for treatment of GI malignancies as well.168–170 For example, in a series of 10 gallbladder cancer patients treated to a median dose of 59 Gy with daily US localization, all but one experienced grade 2 or less acute toxicity.168
As newer IGRT approaches are introduced in the clinic, the future role of US remains unclear. Declining utilization was noted in a recent survey.131 Nonetheless, an advantage of US is that it does not involve additional ionizing radiation, making it likely that it will always play a role in clinical practice.
Video and Surface Imaging
Video-based techniques for patient positioning have been used for over 25 years. Connor et al.171 described a close-circuit television camera and monitor system plus a videodisc recorder, which reduced positional errors to less than 1 mm. The recorder stored a reference image of each treatment setup and was superimposed, in reverse color, on the live camera image. Investigators at the University of Chicago developed an online video “subtraction” setup system, consisting of wall- and ceiling-mounted charge-coupled device cameras linked to a computer equipped with a frame grabber.172,173 After optimal positioning, a reference image is obtained and, on subsequent days, is subtracted in real time from live video images. Subtraction images are displayed on an in-room monitor and used to interactively realign the patient. Milliken et al.172 reported high levels of accuracy in both 2D and 3D repositioning using this system. Johnson et al.173 performed a clinical study of this system in five HNC patients undergoing twice daily RT. Conventional setup was used in the morning, with the video used simply to record the final patient position. In the afternoon, patients were first aligned with conventional techniques and then live subtraction images were used for setup correction. Although the standard deviation of setup error using room lasers was σ = 3.9 mm, it was reduced by 56% (σ = 1.7 mm) using video setup. The entire process generally required approximately 1 minute.
Several investigators have evaluated video-based setup techniques in breast cancer patients. Baroni et al.174 developed a video system based on optoelectronics and close-range photogrammetry that captures in real time the position of markers on the patient that are used to monitor and adjust the patient position. Bert et al.175 investigated a commercial stereovision surface imaging system (AlignRT, Vision RT Ltd, London, UK) for setup of partial-breast irradiation patients, which uses close-range photogrammetry to generate a 3D image of the patient’s surface. The resultant image is compared to an image generated at simulation or of the patient’s external surface generated from a CT dataset. Phantom studies found that the system was capable of identifying translational shifts and rotations of less than 0.1 degree. The AlignRT system is currently being used clinically in the treatment of breast cancer patients undergoing adjuvant whole breast RT (Fig. 11.7).176
A novel use of the AlignRT system is in the setup and monitoring of patient positioning for those undergoing cranial SRS using minimal immobilization. Cerviño et al.177 used AlignRT to monitor positioning in patients immobilized with only a head mold that leaves the face exposed. Using anthropomorphic head phantoms and volunteers, the motion inside the head mold was small and could be accurately detected by real-time surface imaging. These investigators recently presented their initial experience using this approach in 23 patients undergoing SRS.178 The average setup time for both surface imaging and CBCT was 26 minutes, with surface imaging requiring on average 14 minutes. The mean time from initial setup on the table through the last delivery was 40 minutes. Overall, eight patients (35%) required repositioning during treatment. Others have similarly used video surface imaging in patients undergoing cranial radiosurgery.179
Li et al.180 and Djajaputra and Li181 developed a real-time video-guided IMRT approach in breast cancer patients using a camera capable of capturing full-frame 3D surface images through a single snapshot. Patient setup parameters are determined semiautomatically, and the IMRT leaf segments are modified in real time. Unlike other video approaches, this system compensates for changes in surface topology by modifying the treatment plan rather than adjusting the patient position. This system is also being applied to patients undergoing fractionated stereotactic RT.182
Overall, video and surface imaging approaches are among the least commonly used IGRT technologies in the clinic today, with only 3.2% of responding physicians reporting its use on a recent survey performed in the United States.131 As new commercial systems and new applications are introduced (e.g., real-time positioning monitoring for frameless radiosurgery), utilization of such technologies may increase in the future.
FIGURE 11.7. AlignRT for breast cancer. A: Alignment of patient surface to planning computed tomography using video cameras. B: Surface monitoring showing the region of interest of the breast in pink and the tolerance level displayed on the left in dark blue.
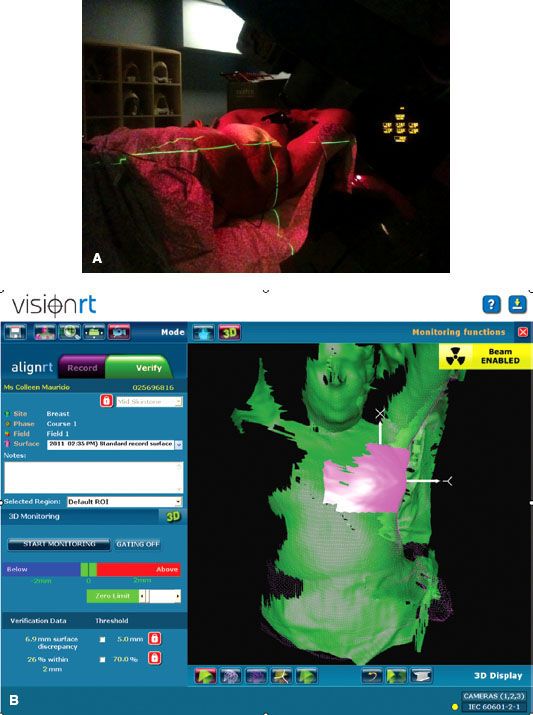
Planar Imaging
Planar imaging approaches, which include both megavoltage (MV) and kilovoltage (kV), are the most common in-room IGRT approaches used today. In a national survey from 2009, the percentage of respondents using megavolt- and kilovolt-planar systems were 63% and 58%, respectively.131 These systems were used in nearly all disease sites, particularly CNS tumors and prostate cancer (together with implanted fiducial markers). MV-planar systems were adopted earlier, with the majority of users (53%) having implemented them by 2004. The adoption of kilovolt-planar–based systems occurred later, with the majority of users (54%) having adopted them by 2006.
Electronic Portal Imaging Devices
Electronic portal imaging devices (EPIDs) provide a means of generating an electronic image of a treatment field with the patient on the treatment table. Similar to conventional portal imaging, EPIDs produce images using the therapeutic (MV) beam. However, EPIDs overcome many of the limitations of conventional port films, including delays due to image processing. Moreover, EPID images can be digitally processed for better visualization of the relevant anatomy and stored for offline review. Numerous EPIDs have been introduced including video-based, liquid ion chamber, and solid-state systems. Most commercial systems in use today are based on flat-panel amorphous silicon (aSi) detectors. With this method, a scintillator first converts x-rays to visible light. A photodiode array then converts the light to electrons, which in turn activate pixels in a layer of aSi. The pixels are then read out in successive rows, processed, and displayed on a computer screen for viewing. Clinical studies illustrating the benefits of EPID-based IGRT approaches initially appeared in the early 1990s,183–184,185 and since then it has been studied in many disease sites.186–192 Readers interested in an overview of EPID technologies are referred elsewhere.193,194
Concerns over increased workload and excess dose have increased interest in on offline EPID approaches. One approach is the so-called shrinking action level strategy.195 Initially, EPID images are obtained on a given number (Nmax) of consecutive days. The 3D setup deviation is calculated offline, and the length of the deviation vector is compared to a predetermined “action level.” If exceeded, a setup correction is performed at the next session. The feasibility of this approach was demonstrated in a multi-institutional prostate cancer trial.196 Favorable results have also been reported in lung cancer197 and HNC.198 An alternative approach is the “no action level” strategy, whereby the mean setup error over a fixed number of fractions is calculated and always corrected for.199
EPID is useful for prostate localization in conjunction with implanted seed markers.200,201 Pouliot et al.202 presented an overview of the prostate seed marker protocol developed at University of California–San Francisco (UCSF) using EPID. Prior to simulation, three gold markers were inserted (two laterally on each side of the prostate and one in the apex). A planning CT scan was performed, the location of each marker was contoured, and a digitally reconstructed radiograph (DRR) was generated. Prior to treatment, a lateral EPID image was obtained to assess SI and AP shifts, requiring approximately 0.02 Gy of dose. Comparison of the center of mass of the markers with their expected position on the DRR was used to evaluate the need for repositioning. If shifts were greater than 3 mm, the couch was adjusted. Most investigators report excellent marker visualization,200–201,202,203,204 particularly when gold markers are used with a minimum diameter of 0.9 mm.201 At least two gold markers are typically visible,203 and high reproducibility has been reported using this approach.204,205 Although marker migration is a potential concern, several investigators have reported minimal migration of implanted markers.200–201,202,203,204 Kupelian et al.206 evaluated seed marker position throughout the course of treatment in 56 prostate cancer patients. Of 2,037 alignments, the average directional variation of all intermarker distances was –0.31 ± 1.41 mm. Only two markers (1%) showed frequent changes in position, most likely caused by prostate deformation. Of note, others have reported marker movement in patients undergoing hormonal therapy as the prostate involutes.207
Limited data exist for EPID and implanted markers in other tumor sites.208 EPID has been compared with CBCT nongenitourinary (GU) sites, with some studies reporting superior setup accuracy with CBCT.209–211 Topolnjak et al.210 compared the two modalities in 20 breast cancer patients undergoing adjuvant RT, noting that EPID underestimated the bony anatomy setup error by 20% to 50%.
Several investigators have reported outcomes of patients treated with EPID-based IGRT. Nichol et al.212 treated 140 stage T1 or T2 prostate cancer patients to 75.6 Gy with daily EPID setup corrections based on bony anatomy. Overall, late grade 2 or higher GI and GU toxicities were noted in 2% and 1% of patients, respectively. Others have reported favorable results using EPID and the shrinking action level approach.213 Ost et al.214 compared acute GI and GU toxicity in 196 prostate cancer patients treated with postoperative salvage RT. Overall, patient position was corrected using EPID (prior to 2006, n = 116) or CBCT (after 2006, n = 80). Patients treated with CBCT verification had less grade 1 or 2 GU toxicity compared to those treated using EPID. No differences were seen in GI or high-grade GU toxicity between the two groups.
EPID has long been among the most common in-room IGRT technologies used clinically. In the national IGRT survey,131 EPID was the most commonly used IGRT technology across nearly all disease sites. With the proliferation of newer technologies, notably CBCT, however, its use may decrease in the future.
CyberKnife
CyberKnife (Accuray Inc, Sunnyvale, CA) consists of a compact X-band 6 MV linear accelerator coupled to a multijointed robotic manipulator with 6 degrees of freedom (Fig. 11.8).215 The current generation of CyberKnife technology consists of two precisely calibrated diagnostic x-ray tubes fixed to the ceiling of the treatment vault and two nearly orthogonal aSi flat-panel detectors. After coarse alignment, projected images from the cameras are automatically registered with the DRRs from the planning CT. Changes in target position are relayed to the robotic arm, which adjusts pointing of the treatment beam. During treatment, the robotic arm moves through a sequence of positions (nodes). At each node, a pair of images is obtained, the patient position is determined, and adjustments are made.
CyberKnife was initially based on tracking the skeletal anatomy of the skull and upper spine, limiting treatment to tumors of the brain, head and neck, and upper spine. Subsequently, the ability to track implanted fiducial markers was introduced, allowing treatment of lower spinal tumors with submillimeter precision.216 More recently, software has been developed that obviates the need for implanted fiducials in spine patients and enables respiratory tracking.
Several preclinical studies have been published reporting high levels of accuracy of CyberKnife. Murphy and Cox217 noted a mechanical accuracy of the beams of 0.7 mm with a calibration accuracy of plus or minus 0.5 mm along each axis. Yu et al.218 reported submillimeter accuracy in a phantom study using fiducial markers. Many clinical studies have described favorable outcomes for patients treated with CyberKnife, including adenoma,219,220 schwannoma,221 glioma,222,223 brain metastases,224 meningioma,225 trigeminal neuralgia,226,227 AVM,228 and tumors abutting the optic nerves or chiasm.229,230
A provocative use of the CyberKnife is for pediatric brain tumors. CyberKnife avoids the need for a rigid head frame and, in select children, general anesthesia. Moreover, frameless treatment can be fractionated. Investigators at Baylor University reported promising results with the CyberKnife in infants231 and the general pediatric population.232 Giller et al.232 treated 21 children (median age, 6 years) with CNS tumors, with a median dose of 18.8 Gy primarily delivered in a single fraction. At a median follow-up of 18 months, 10 children had evidence of decreased tumor size or stable disease on follow-up imaging.
Many studies have focused on CyberKnife for spinal lesions (Table 11.1).233,234–237,238,239,240,241–244,245,246,247,248 Dodd et al.233 treated 51 patients with 55 benign intradural extramedullary spinal tumors, with a median dose of 19.6 Gy delivered primarily in 1 or 2 fractions. All patients with more than 2 years of follow-up had either stable or smaller tumors on repeat imaging. Most patients had stable or improved symptoms. One developed a spinal cord injury 8 months following treatment. Investigators at the University of Pittsburgh reported on 125 primarily malignant spinal lesions (115 patients) treated to a median dose of 14 Gy in 3 to 5 fractions (Fig. 11.9).238 At a median follow-up of 18 months, no patient developed new symptoms or had evidence of RT sequelae, despite the fact that 68% had received prior RT. Of 79 patients presenting with pain, 74 (94%) noted improvement. Others have reported similarly promising results in patients with benign and malignant spinal tumors.235–249
Recently, CyberKnife has been used to treat extracraniospinal sites.250–258 However, outcome data remain limited. King et al.252 treated 41 low-risk prostate cancer patients, prescribing 36.25 Gy in 5 fractions of 7.25 Gy. At a median follow-up of 33 months, two patients developed grade 3 GU toxicity and no patients developed grade 3 or higher GI toxicity. Less rectal toxicity was observed with an every-other-day approach versus 5 consecutive days (0% vs. 38%; P = .0035). At last follow-up, all patients remained biochemically controlled. Of 32 patients with 1-year minimum follow-up, 25 (78%) achieved a PSA nadir of 0.4 ng/mL or less.
Several investigators have explored the use of CyberKnife in patients with lung cancer.251,254 Nuyttens et al.251 treated 20 patients with lung tumors in whom fiducial markers had been implanted for tumor tracking. A system of light-emitting diodes placed on the patient’s abdomen was used to monitor the location of fiducials with respect to respiratory motion and provide feedback to the robotic arm of the CyberKnife for tracking. Four-dimensional CT simulation scans were acquired, and patients were treated with hypofractionated radiation (36 to 60 Gy in 3 fractions). With a median follow-up of 4 months, no local failures were observed.
FIGURE 11.8. CyberKnife radiosurgery system. Two amorphous silicon x-ray detectors are positioned orthogonally to the treatment couch. (From Gerszten PC, Ozhasoglu C, Burton SA, et al. Cyberknife frameless stereotactic radiosurgery for spinal lesions: clinical experience in 125 cases. Neurosurgery 2004;55:89–99, with permission.)
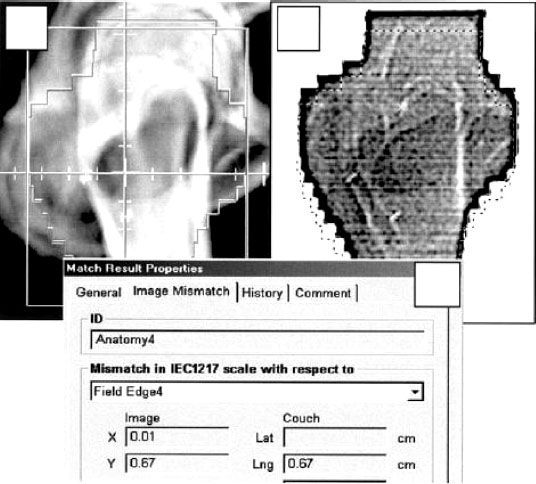
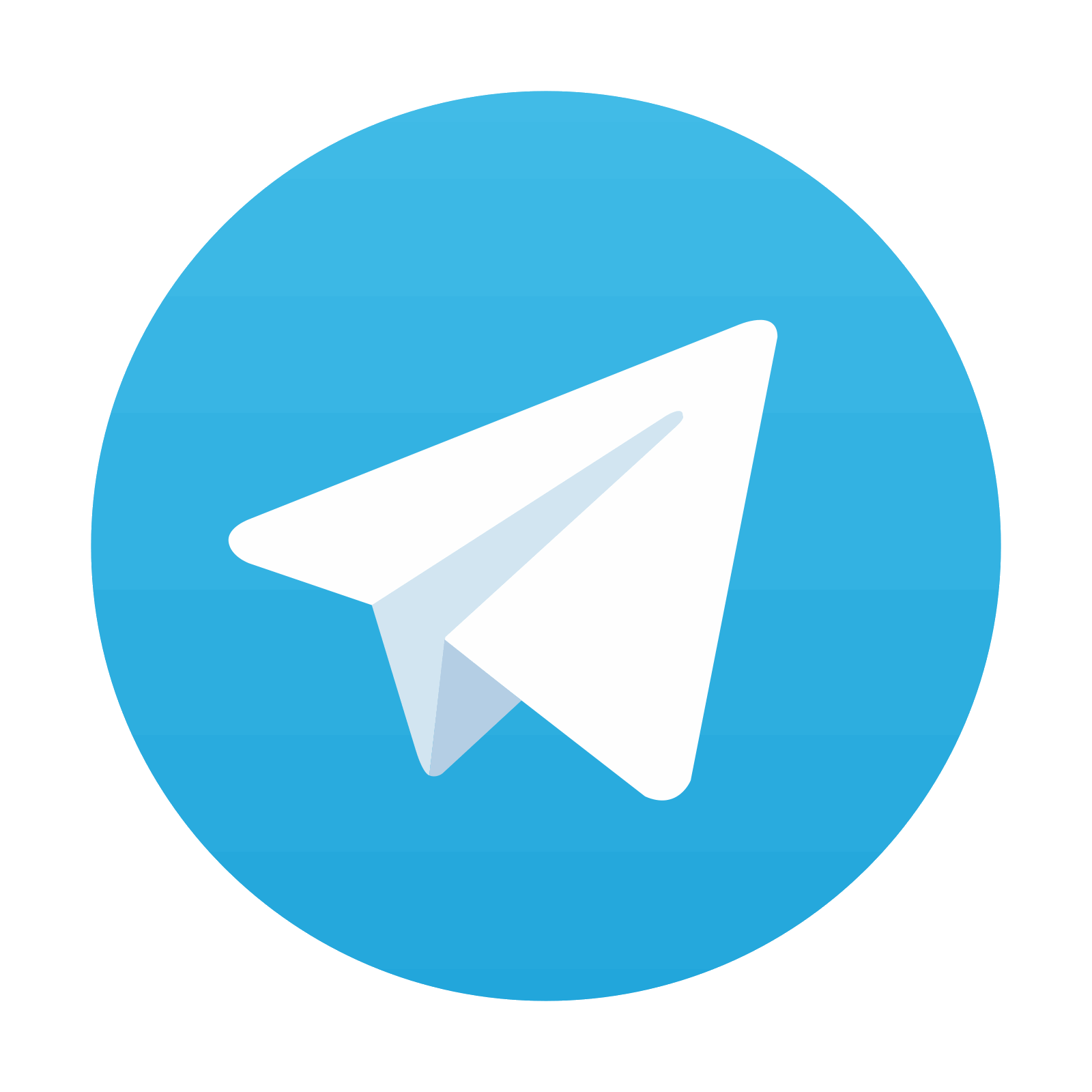