Fig. 3.1
Structure and cellular composition of the seminiferous epithelium in the human testis. The seminiferous epithelium, as seen in this schematic drawing of a cross section of a seminiferous tubule, is composed of Sertoli and germ cells. The BTB is constituted by actin-based tight junctions, basal ectoplasmic specialization (ES), and gap junctions, as well as the intermediate filament-based desmosome. As one of the tightest blood–tissue barriers in the human body, the BTB divides the seminiferous epithelium into the adluminal (apical) and basal compartments. Spermatogonia (both type A, B and undifferentiated) and preleptotene spermatocytes reside in the basal compartment, whereas the other primary and secondary spermatocytes and post-meiotic spermatids are found in the apical compartment. The most notable junction in the mammalian testis including humans is the ES, which is typified by the presence of actin microfilament bundles sandwiched in-between cisternae of endoplasmic reticulum and the apposing Sertoli cell–cell plasma membranes. The ES at the BTB is called basal ES while the ES at the Sertoli–spermatid interface is designated apical ES. Since developing germ cells (e.g., preleptotene spermatocytes) and developing spermatids are being transported across the seminiferous epithelium, Sertoli cell–Sertoli cell and Sertoli–germ cell junctions are continuously remodeling throughout the 6 stages of the epithelial cycle of spermatogenesis in the human testis
In humans, Sertoli cells undergo mitotic proliferation in two separate phases. The first phase takes place shortly after birth during the neonatal-infantile period when the Sertoli cell population increases via mitotic proliferation [40]. During puberty, Sertoli cells again rapidly divide mitotically [40], giving rise to ~500 × 106 Sertoli cells per testis (each adult human testis weighs ~18–19 gm). The Sertoli cell number declines to ~300 × 106 by 50–85 years of age, associated with a reduction in daily sperm output [8]. Each testis has ~4.5 × 106 Sertoli cells in mice [41] and 30−40 × 106 Sertoli cells in rats [42–44] compared to 500 × 106 in men [8], and a daily sperm production of 8x [41], 70x [45], and 200×106 [46], respectively. The number of Sertoli cells is determined by FSH, thyroid hormones, growth hormone, and several paracrine growth factors [40]. Outside the tubules in the interstitial space lay the Leydig cells, fibroblasts, and some macrophages and microvessels (Fig. 3.1). Leydig cells produce testosterone (T) under the influence of luteinizing hormone (LH) since LH receptors are limited to Leydig cells in human and rodent testes. Interestingly, the T level in the interstitial fluid and seminiferous tubule fluid is at least 70- and 50-fold higher than the T level in the systemic circulation in the rat [47]. In humans, the intratesticular T (e.g., interstitial fluid) level is also 100-fold higher than the systemic circulation [48–50], illustrating a considerably higher T level is maintained in the testis to support spermatogenesis.
Sertoli Cells and the Blood–Testis Barrier (BTB)
The seminiferous epithelium of the mammalian testis is further divided into two functional compartments: (1) the basal, and (2) the adluminal (apical) compartments (Fig. 3.1), due to the presence of the Sertoli cell blood–testis barrier (BTB) located near the basement membrane. The BTB is constituted by coexisting actin-based basal ectoplasmic specialization (basal ES) and tight junction (TJ), as well as coexisting basal ES and gap junction, and intermediate filament-based desmosome [51, 52]. As such, undifferentiated spermatogonia, both type A and B spermatogonia, and preleptotene spermatocytes differentiated from type B spermatogonia reside in the basal compartment. More advanced primary spermatocytes, secondary spermatocytes, and all haploid spermatids including spermatozoa reside in the adluminal compartment (Fig. 3.1).
Briefly, the BTB provides a unique microenvironment in the adluminal compartment for meiosis I/II and post-meiotic spermatid development so that these events are segregated from the systemic circulation, behind an immunological barrier. Studies have shown, however, that the BTB contributes minor significance to the immune privilege of the testis since germ cells residing in the basal compartment are equally immunogenic, containing multiple testis-specific antigens [53, 54]. Instead, the testis immune privilege is maintained mostly by Sertoli cells through their secretory immunosuppressive biomolecules: cytokines, bioactive lipids and peptides, and androgens from Leydig cells [55]. The BTB in humans is established at puberty by ~12–13 years of age when Sertoli cells cease to divide and become fully differentiated, concomitant with the onset of meiosis I/II [40]. It is of interest to note that human Sertoli cells, similar to Sertoli cells in rodents, can be de-differentiated, becoming mitotically active when they are exposed to fetal bovine serum [40], such as cultured in media containing 5–10% fetal bovine serum (v/v) [56]. Under such conditions, Sertoli cells can be maintained through multiple passes in vitro without detectable changes in their functional and physiological properties [57].
BTB function in rodents is dependent on testosterone [58–61]. However, treatment of normal men with testosterone plus levonorgestrel (LNG), which is capable of suppressing spermatogenesis and causing azoospermia or oligospermia with suppression of intratesticular androgen levels, did not affect BTB function since the distribution of claudin-3 remained relatively unaltered [62]. However, in a more recent study in which healthy men were treated with T plus LNG together with the GnRH antagonist acyline or the 5α-reductase inhibitor dutasteride (in order to provide added suppression of spermatogenesis), there was considerable down-regulation of claudin-11, connexin-43, and vinculin at the BTB compared to men treated wth T + LNG alone [63]. Collectively, these findings suggest that human BTB is regulated in a manner similar to rodents. Some species differences exist, however, such as the extent of androgen-dependency, since it appears that a considerable reduction in the intratesticular T level is necessary to suppress the BTB integrity in humans.
Due to the limited access to normal human testes for functional analysis, it is difficult to compare the regulation of the human BTB integrity with that of rodents. With advances in cell and tissue culture techniques, however, human Sertoli cells, using media containing fetal bovine serum, have been cultured and maintained successfully for several weeks in vitro [56, 57, 64–66], with a functional tight junction-permeability barrier [56, 64]. Using this approach, the organization of F-actin that supports BTB integrity has been investigated at the human Sertoli cell BTB. It is now known that human Sertoli cell F-actin organization is maintained, similar to that of rodents, by actin binding proteins, including Arp2/3 (actin related protein 2/3) complex and Eps8 (epidermal growth factor receptor pathway substrate 8) that confers branched actin network and bundled actin microfilaments, respectively [57]. Furthermore, exposure of human Sertoli cells to environmental toxicants, such as CdCl2, or bisphenol A (BPA), rapidly perturbs F-actin organization in these cells. Changes in the distribution of cell adhesion proteins at the BTB, including the TJ-protein ZO-1, and the basal ES proteins N-cadherin and ß-catenin, from the cell surface to the cell cytosol [57], likely reflect degradation via an endosome-dependent degradation pathway. These changes disrupt integrity and function of the BTB. It is expected that much information will be obtained using this in vitro system to gain insight into the biology of the human BTB.
Cell–Cell Interactions in the Testis
Due to the presence of the BTB, germ cell development, in particular post-meiotic spermatid development, relies almost exclusively on the structural, nutritional, and paracrine support of Sertoli cells. This is mediated via cell junctions at the Sertoli–germ cell interface, involving multiple genes and their proteins [37, 38, 67, 68] (Fig. 3.2). Even though undifferentiated spermatogonia [e.g., spermatogonial stem cells (SSCs)], type A and type B spermatogonia, and preleptotene spermatocytes reside outside the BTB, they also rely on the Sertoli cell for functional and structural support through the expression of unique genes (Fig. 3.2). This is particularly true for SSCs which are located at the stem cell niches—usually at the base of the seminiferous epithelium wherein three seminiferous tubules meet, adjacent to the microvessels in the interstitium. In short, SSCs rely on Sertoli cells for structural and functional support, besides biomolecules from the microvessels [69, 70].
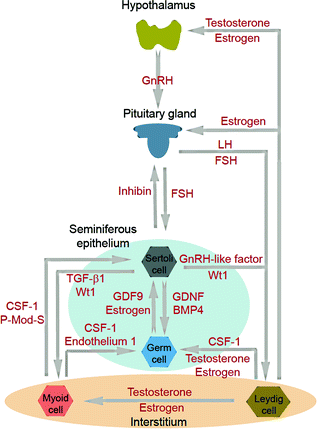
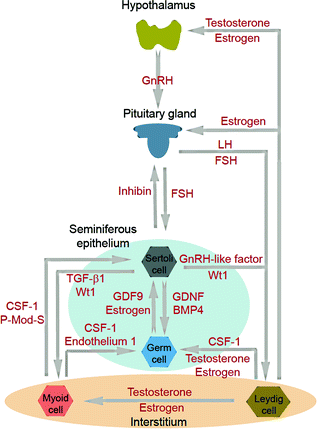
Fig. 3.2
Cell–cell interaction within the testis and its functional relationship to the hypothalamic–pituitary–testicular axis. The classic hormonal regulatory axis that exerts its regulatory effects on the testis through the release of gonadotropin-releasing hormone (GnRH) from the hypothalamus, which in turn regulates the secretion of luteinizing hormone (LH) and follicle-stimulating hormone (FSH) from the pituitary gland. Testosterone produced by interstitial Leydig cells provides the feedback loop to modulate the production of GnRH from the hypothalamus and thereby LH and FSH from the pituitary gland. In humans and primates in contrast to rodents, testosterone has no direct feedback effect on the pituitary [240]. Inhibin produced by Sertoli cells provides selective feedback to the production of FSH. There is cross talk among germ cells (including spermatogonial stem cells) and Sertoli cells in the seminiferous epithelium, as well as Leydig cells and peritubular myoid cells in the interstitium. Studies have shown an array of growth factors, genes and hormones are involved. Abbreviations: CSF1, colony stimulating factor 1; BMP4, bone morphogenetic protein 4; GDF9, growth differentiation factor-9; GnRH, gonadotropin-releasing hormone; P-Mod-S, peritubular myoid cell modulates Sertoli cell factor; TGF-β1, transforming growth factor-1; Wt1, Wilms’ tumor 1
Sertoli cells also modulate spermatogenesis through cross talk with Leydig cells. Sertoli cells stimulate Leydig cell differentiation but inhibit Leydig cell steroidogenesis [71–73]. One of the paracrine factors produced by Sertoli cells that modulates Leydig cell function has been partially characterized [74]. Studies using genetic models have shown that Sertoli cells maintain Leydig cell number (both fetal and adult Leydig cells) and support Leydig cell development [75, 76], as well as determine Leydig cell differentiation status and cell fate through the Wt1 gene [77]. On the other hand, Sertoli cells also interact with germ cells to support spermatogenesis through multiple paracrine factors [37, 78, 79] (Fig. 3.2).
There is also functional cross talk between Sertoli cells and the tunica propria, in particular peritubular myoid cells (Fig. 3.2). For instance, Sertoli cells work in concert with peritubular myoid cells in the production and deposition of extracellular matrix (ECM) components [80] to produce and maintain the basement membrane, which is a modified form of ECM [35, 36]. Studies have also suggested that peritubular myoid cells modulate Sertoli cell function wherein myoid cells produce a paracrine factor known as P-Mod-S that regulates Sertoli cell function [81]. However, the identity of this factor remains to be clarified. Another report has shown that while peritubular myoid cells do not secrete clusterin or α2-macroglobulin (a wide-spectrum protease inhibitor [82]), co-cultures of myoid cells with Sertoli cell promote Sertoli cell α2-macroglubulin and clusterin secretion [83]. It must be noted, however, that unlike Sertoli cells, which can be maintained in culture in serum-free medium (e.g., F12/DMEM with several growth factors [84]) for up to ~2-wk, peritubular myoid cell cultures require the presence of serum proteins, such as ~5–10% fetal calf serum for their survival [83].
The most conclusive study thus far to illustrate the importance of peritubular myoid cells in spermatogenesis is the generation of a peritubular myoid cell-specific androgen receptor (AR) knockout (PTM-ARKO) mouse model [85]. PTM-ARKO male mice have reduced seminiferous tubule fluid production and are azoospermic and infertile, with reduced expression of certain androgen-dependent Sertoli cell genes [85]. These findings illustrate that ARs in Sertoli cells fail to assume the function of ARs in myoid cells to sustain spermatogenesis. Furthermore, these findings demonstrate that Sertoli cell androgen-dependent gene expression is modulated by peritubular myoid cells, unequivocally demonstrating that Sertoli and peritubular myoid cells are functionally connected. Interestingly, studies using Amh-Cre to induce expression of the Diphtheria toxin receptor in Sertoli cells to cause controlled, cell-specific, and acute ablation of the Sertoli cell population in adult mice have shown that Sertoli cells also control peritubular myoid cell fate, including its differentiation status and cell population [76]. Using this approach, Sertoli cells were shown to modulate testicular vascular network development, including modulating circulating testosterone levels in adult mice [86]. It should be noted that much of the information stated above and depicted in Fig. 3.2 is derived from studies in rodents. Clearly, further studies are needed to understand the cell–cell interactions between Sertoli cells, Leydig cells, and peritubular myoid cells in the human testis.
Spermatogonia and Self-renewal
Spermatogonia are the progenitor cells of all germ cells and are designated type A and type B [87, 88]. In humans, type A spermatogonia are further categorized into progenitor dark type A spermatogonia (Ad) and pale type A spermatogonia (Ap) which are capable of undergoing mitotic proliferation for self-renewal [89] (Fig. 3.3). Ap are the predominant spermatogonia that survive radio- and chemotherapy while Ad are largely eliminated during such treatment, illustrating that Ap are the potential spermatogonial stem cells [90]. At present, it is generally accepted that Ad constitute a pool of reserve spermatogonia which remain quiescent but can give rise to Ap when needed and are considered to be the ‘true’ spermatogonial stem cells in humans [4, 91]. The molecular basis for the regulation of human spermatogonia remains largely unknown. cKIT, a transmembrane protein tyrosine kinase receptor (also known as stem cell factor receptor or CD117), and its ligand cKIT ligand (also known as stem cell factor (SCF)) are known to be involved in the differentiation of spermatogonia in humans, rodents, and primates [91]. cKIT is expressed in spermatogonia, round spermatids, and spermatozoa as well as in Leydig cells whereas its ligand or SCF is primarily expressed in Sertoli cells in humans [92]. However, cKIT is not expressed in spermatocytes or elongating spermatids [93]. Expression of cKIT in type A spermatogonia varies across the seminiferous tubules, being highest in proliferating Ap spermatogonia and type B spermatogonia [93–95], illustrating the involvement of cKIT/SCF in spermatogonial self-renewal and differentiation. The reduced staining of cKIT in type A spermatogonia has been shown in men with subfertility, and its reduced expression is associated with an increase in apoptosis in type A spermatogonia [96].
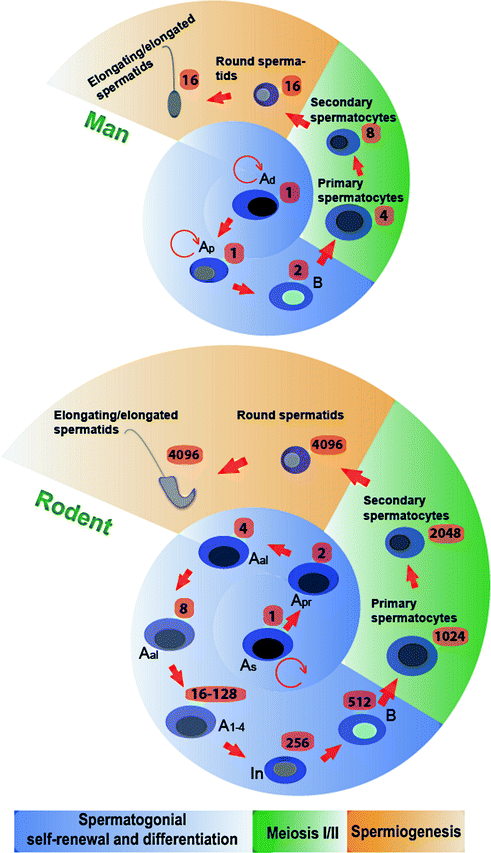
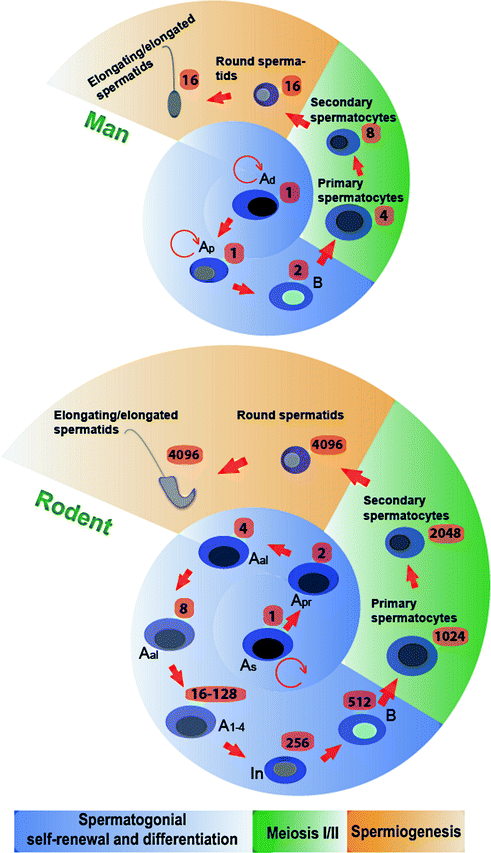
Fig. 3.3
Comparison of germ cell development in the human versus the rat testis. While the development of germ cells through spermatogenesis is similar between the two species, there are some notable differences such as during spermatogonial self-renewal and differentiation. In the rat, Asingle spermatogonia (As) divide and form Apaired spermatogonia (Apr), which further divide to form Aaligned spermatogonia (Aal). The differentiating Aal undergo 5 cell divisions (A1-4 and In) to form type B spermatogonia. In humans, both Adark spermatogonia (Ad) and Apale spermatogonia (Ap) are capable of undergoing self-renewal through mitosis, and Ap also give rise to type B spermatogonia, the latter of which are then differentiate to form preleptotene spermatocytes. In the human testis, preleptotene spermatocytes formed in stage III (concomitant with spermiation that takes place at late stage II) of the epithelial cycle are transported across the BTB to enter the adluminal compartment to prepare for meiosis I/II which takes place at stage VI of the epithelial cycle, and Sa spermatids, the equivalent of round spermatids in the rat, are found in stage I. In the rat, a single diploid As can give rise to 4096 haploid sperm; whereas in the human, only 16 sperm are derived from one type A spermatogonium. The number of different types of germ cells that can be derived from a single committed type A spermatogonium is indicated. This figure was prepared based on the information of earlier reports [1, 51, 127] and in the model of rat spermatogenesis it is noted that Apr and Aal spermatogonia have been shown to be able to revert to As [241]
GFRA1, α6-INTEGRIN, PLZF, GPR125, SALL4, and THY1 are undifferentiated spermatogonia markers in mouse, monkey, and man, whereas UTF1 (undifferentiated embryonic cell transcription factor 1) and FGFR3 (fibroblast growth factor receptor 3, also known as CD333) are molecular markers specific for human type A spermatogonia [91]. The molecular basis for the transition of undifferentiated spermatogonia to type A spermatogonia has been established in rodents and involves retinoic acid (RA), the active metabolite of vitamin A, which is also involved in the initiation of meiosis [97]. Indeed, treatment of mice with the pan-retinoic acid receptor antagonist, BMS-189453 that blocks the action of RA in the mouse testis, leads to reversible infertility due to meiotic arrest [98, 99]. The notion that RA is involved in spermatogenesis in humans is based on findings that bisdichloroacetyldiamine WIN 18,446, an inhibitor of retinoic acid synthesis, inhibits ALDH1A2 and induces reversible infertility in mice, rats, monkeys, and men [100–103]. More study is needed to define the role of retinoic acid in human spermatogonial differentiation.
Spermatogonial differentiation is a gonadotropin-dependent event in primates [104] since FSH treatment increases the number of Ap and type B spermatogonia in monkeys [105, 106]. Emerging evidence suggests that FSH plays a role in spermatogonial differentiation in men [107]. However, in normal adult men, gonadotropins (FSH and LH) serve as spermatogonial survival factors by regulating the intrinsic apoptotic pathway to promote germ cell survival but have no effect on germ cell proliferation [108].
Spermatocytes and Meiosis
Spermatocytes, namely leptotene, zygotene, pachytene, and diplotene spermatocytes are found in the human seminiferous epithelium [109] following the initiation of meiosis in humans at puberty [110] (Figs. 3.3 and 3.4). Diplotene spermatocytes undergo meiosis I to form secondary spermatocytes (with haploid number of chromosomes but 2n content of DNA), which rapidly progress to meiosis II so that a single secondary spermatocyte produces two haploid round Sa spermatids. In this context, it is of interest to note that, unlike other somatic cells, neither spermatocytes nor spermatids metabolize glucose; instead, they rely on lactate supplied by Sertoli cells as their energy source [111, 112]. In brief, glucose is taken up by Sertoli cells via the specific glucose transporter GLUT1 and is processed into lactate glycolytically [113] by testis-specific lactate dehydrogenase C4 (LDHC4). Lactate is transported out of Sertoli cells by a monocarboxylate transporter MCT1 and taken up by meiotic and post-meiotic germ cells via their specific monocarboxylate transporter, MCT2 [111]. Studies have shown that energy metabolism of Sertoli and germ cells is regulated by FSH, steroids, insulin and paracrine factors, and their disruption leads to male infertility [112, 114]. It is also noted that defects in meiosis, such as meiotic maturation arrest (or early maturation arrest) is found in ~10% of men with non-obstructive azoospermia (NOA), with the histopathological features of reduced tubule diameter, reduce germ cell number, and degenerating spermatocytes [115].
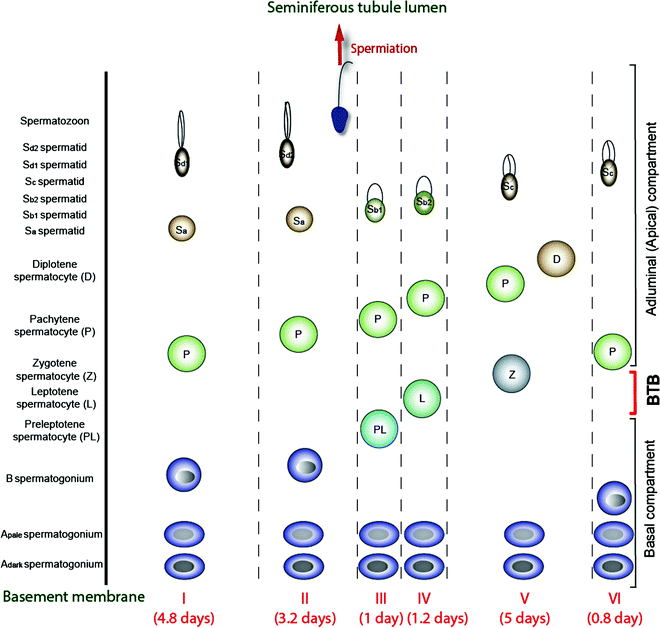
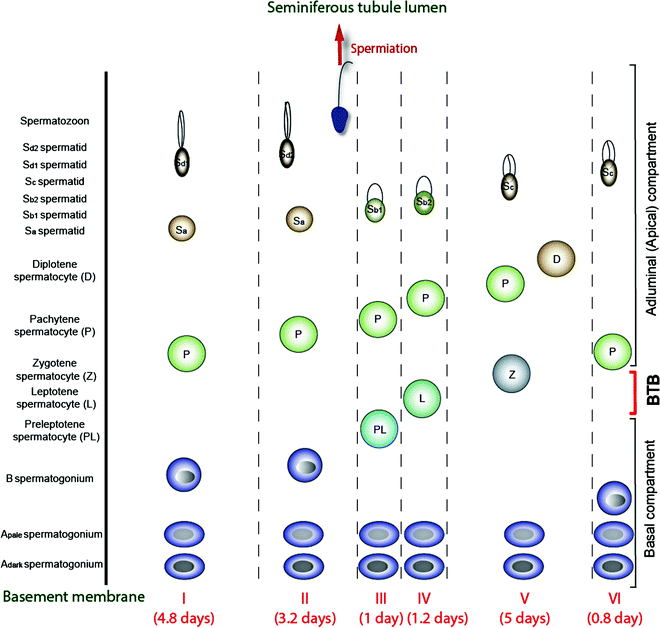
Fig. 3.4
A schematic drawing illustrating the six stages of the epithelial cycle (I-VI) in the human testis, and the associated germ cell types in the seminiferous epithelium in each of these stages. It is noted that spermiation takes place in late stage II, and preleptotene spermatocytes arise in stage III, which are being transported across the BTB in stage III. Meiosis takes place at stage VI of the cycle, and the entire epithelial cycle takes 16 days to complete. The duration of each stage, from I to VI, is also annotated. This drawing was prepared and modified from earlier reports [4, 34, 242], and recent advances in the staging of human spermatogenesis have recently been discussed [243]
Spermatids and Spermiogenesis
The process during which spermatids undergo extensive morphological transformation through round Sa spermatids, Sb1, Sb2, Sc, Sd1, and Sd2, and eventually to spermatozoa is known as spermiogenesis [116] (Figs. 3.3 and 3.4). During this process, no further cellular divisions occur, newly formed round spermatids from secondary spermatocytes are characterized by a small spherical nucleus with the usual array of cytoplasmic organelles, including the Golgi apparatus, mitochondria, and centrioles [116]. Each spermatid undergoes considerable morphological changes that begin with a large granule called the acrosomic granule which is generated by several small proacrosomic granules in the area of the Golgi apparatus that grows over the nucleus. As spermiogenesis continues, the acrosome transforms further, and nuclear chromatin condensation begins [117]. At the final steps, the spermatid nucleus completes its chromatin condensation, and the majority of the cytoplasm eventually detaches from the spermatid to form the residual body, which is engulfed by the Sertoli cell and processed into a phagosome. The phagosome is then transported to the base of the Sertoli cell for lysosomal degradation [118]. In humans, spermiogenesis can be disrupted, leading to: (i) late maturation arrest, manifested by an arrest in development in early spermatids with dark round nuclei, and (ii) hypospermatogenesis, with an arrest in further development of condensed oval spermatids [115]. Mature spermatozoa, once formed, are released into the tubule lumen via the final cellular process of spermatogenesis at spermiation [5, 119]. While the molecular mechanism underlying spermiation remains relatively unexplored, studies in rodents have shown that spermiation is tightly regulated by the spatiotemporal expression of signaling molecules such as p-FAK-Tyr407, as well as actin binding/regulatory proteins (e.g., the Arp2/3 complex, Eps8), involving degeneration of apical ES and generation of laminin fragments [5, 119]. However, this process is poorly understood in humans except that it is likely dependent on FSH and testosterone. So far, human male infertility has not been attributed to defects of spermiation alone [3].
Cycle of the Seminiferous Epithelium
In human as well as rodent testes, the distinctive cellular associations in the seminiferous epithelium along the tubule can be defined into different stages which appear cyclically throughout spermatogenesis, known as the epithelial cycle of spermatogenesis [88, 120, 121]. In the testis of rats, mice, and humans, an epithelial cycle is composed of I-XIV, I-XII, and I-VI stages, respectively [4, 122, 123]. It is of interest to note that spermiation and meiosis I/II take place at stage VIII and XIV, VIII and XII and II and VI, in the rat, mouse, and human testis, respectively. It takes about 16 days to complete an epithelial cycle in the human [124] versus 8.6 days in the mouse [125] and 12.8 days in the rat [126]. This is the time it takes for a given spot in a seminiferous tubule at a specific stage of the epithelial cycle, such as at stage II, when observed under the stereomicroscope, to undergo cyclic staging and become stage II again. However, the duration of spermatogenesis, i.e., from type A spermatogonia to spermatozoa, is ~68 days (4.2 cycles) in humans versus ~35 days (4 cycles) and ~58 days (4.5 cycles) in mice and rats as noted in Fig. 3.4 for humans (for reviews, see [1, 127, 128]). This is the time it takes for a single human diploid Ap spermatogonium (progenitor germ cell, wherein Ad spermatogonium is the regenerative reserve stem cell) to develop into multiple haploid spermatozoa which requires ~4.2 cycles. In this context, it is noted that the staging of the epithelial cycle is largely defined according to changes in the Golgi region of developing spermatids, namely the acrosome, when it is visualized by the periodic acid Schiff’s reaction (PAS). In mouse, rat, and human, spermatids can be divided into 16, 19, and 6 steps, respectively, throughout spermiogenesis before they develop and transform into spermatozoa. The classification of human spermatids into 6 steps of Sa, Sb1, Sb2, Sc, Sd1, and Sd2 was based on the use of osmium-dichromate as fixative for the human testis, which was then stained with osmium and examined by transmission electron microscopy [117].
Factors that Regulate Spermatogenesis
Numerous factors are known to affect human spermatogenesis. Below, we highlight regulators that are well established or are rapidly developing in the field.
Hormonal Regulation
Hormonal regulation of spermatogenesis in humans is a complex biological event involving both testosterone and estradiol-17ß [10, 13, 14, 129, 130] and is tightly regulated by the hypothalamic–pituitary–testicular axis by follicle-stimulating hormone (FSH) and luteinizing hormone (LH) that exert their effects on Sertoli and Leydig cells, respectively. Besides these hormones, inhibins, activins, follistatin, and other paracrine factors are also involved. Since the hormonal regulation of spermatogenesis in humans has been eminently reviewed recently [11, 13, 131–136], interested readers are encouraged to seek further information from these earlier reviews. Below is a summary of recent findings pertinent to the hormonal regulation of human spermatogenesis.
FSH
FSH is an important regulator of spermatogenesis through effects on Sertoli cells where FSH receptors are expressed. FSH activates at least five signaling pathways in Sertoli cells: cAMP-PKA, MAPK, PI3 K-AKT, intracellular Ca, and phospholipase A2 [137–139]. Gene expression profiles indicate that FSH regulates a panoply of Sertoli cell genes. FSH promotes Sertoli cell proliferation before puberty, while after puberty, FSH activates Sertoli cell to support germ cell development. Despite the important role of FSH in spermatogenesis, this hormone, unlike testosterone (see below), is not considered essential to, but rather fine-tunes spermatogenesis. For instance, deletion of the FSH-ß subunit in mice led to infertility in FSH-deficient females due to a block in folliculogenesis, but FSH-deficient males were fertile even though the testes of these mice were reduced in size [140, 141]. Furthermore, men with inactivating mutations of the FSH-β gene or the FSH receptor have impaired spermatogenesis but generally remain fertile—an observation explained in part by the finding that the FSH receptor possesses low-level constitutive activity in the absence of FSH [129, 142–144] (see Chap. 6). On the other hand, it is of interest to note that men with congenital complete hypogonadotropic hypogonadism (CHH) are usually treated with hCG (human chorionic gonadotrophin) together with FSH to induce testis development, spermatogenesis, and fertility (see Chap. 20). Also, men with idiopathic oligoasthenoteratozoospermia treated with recombinant FSH for 3 months were found to have considerable improvement in seminal parameters vs. untreated controls [145, 146].
LH
The primary function of LH is to stimulate the production of testosterone by Leydig cells [147]. LHCG-receptor (luteinizing hormone/choriogonadotropin receptor, also called LHCG-R or LHR) expression begins in fetal Leydig cells [148]. Human males with inactivating mutations of the LHCG-R develop ambiguous genitalia and are testosterone-deficient indicating that hCG/LH signaling is essential for testosterone production in the fetus and adult [149–151]. LH stimulates testosterone production by stimulating the expression of key steroidogenic enzyme genes as well as transcription factors that are required for testosterone biosynthesis to support spermatogenesis and other male reproductive function [152]. Ligand binding to the LHCG-R stimulates adenylate cyclase, increases cAMP production and phosphorylates target proteins through protein kinases A and C, activates ERK1 and 2, and increases calcium signaling [148]. Interestingly, accumulating evidence supports the notion that steroidogenesis in human fetal testes is highly sensitive to environmental toxicants or elected lifestyle (e.g., cigarette smoking) which are disruptive to LH-mediated testosterone production by Leydig cells [17, 153].
Intratesticular Testosterone (ITT) Microenvironment
Testicular aspirations of normal men have shown that T is the predominant intratesticular sex steroid [48, 49, 154]. ITT concentration in men averages 609 ± 50 ng/ml, which is much higher than the average serum T level of 3.7 ± 0.3 ng/ml [48–50]. This gradient between the testis and serum is similar to findings in rodents, wherein ITT in rat testes is ~100-fold higher than the serum T level [47, 155]. Collectively, these findings support the notion that a high ITT level is necessary to maintain spermatogenesis. For instance, the high level of T in the testis is known to support spermatid adhesion, spermiogenesis, and BTB function in rodent testes [5, 51, 156]. In this context, it is of interest to note that the ITT concentration at ~2000 nM vesurs ~12 nM of T in serum in normal men is considerably higher than that of SHBG/ABP (sex hormone binding globulin/androgen binding protein, ~50 nM; note: SHBG/ABP reduces androgen bioavailability), suggesting that most ITT is bioavailable [154]. Studies by Jarow et al. found that ~70% of the total ITT is bioactive based on a novel androgen bioactivity assay [154]. Interestingly, neither ITT concentrations nor intratesticular bioactive androgen levels were strongly correlated with serum T concentration (correlation coefficients, r = 0.38) nor serum bioactive androgen level (r = 0.46). Yet SHBG/ABP levels in the testis and serum in humans were strongly correlated [48, 154]. These data thus fail to explain the disparity between intratesticular and serum bioactive androgen levels [48, 154, 157]. In contrast, Roth et al. found that T levels in testicular aspirations (i.e., to obtain ITT) and serum of fertile men in samples obtained simultaneously were strongly and positively correlated (r = 0.67) [158]. The different results in the two studies may be due to different assay approaches. In this context, it is of interest to note that a recent report has shown that high ITT is not necessary to support spermatogenesis in humans [159], and proposed that the ITT level may be high only because T is synthesized locally by Leydig cells in the interstitial space of the testis. Thus, the physiological significance of the ITT level to support spermatogenesis requires additional further studies.
Importantly, the earlier report of Roth et al. also demonstrated considerable variations in ITT levels in fertile men which correlated very strongly with serum LH levels (r = 0.87). It was hypothesized that LH secretory pulsatility might cause pulsatility in ITT concentrations [158]. In fact, ITT pulsatility was suggested by an earlier study that quantified T and estradiol concentrations over 4 h in cannulated gonadal veins of men with varicocele [32]. While the importance of ITT pulsatility to spermatogenesis remains to be established, it may influence the cyclic nature of spermatogenesis during the epithelial cycle. For instance, the requirements of the seminiferous epithelium to support androgen-dependent cellular events at late stage II during spermiation are quite different from stages VI when meiosis takes place. As such, it is likely that the ITT level required at stage II is different from stage VI and other stages to support spermatogenesis. Nonetheless, the ITT concentration necessary to support spermatogenesis in humans has not been established by quantifying T levels in intratesticular fluid obtained during micro-TESE (microdissection testicular sperm extraction) [160]. Furthermore, studies in rodents have shown that T, besides playing a crucial role in maintaining germ cell adhesion in particular developing spermatids, modulates endocytic vesicle-mediated protein trafficking [161]. Due to the high level of cellular activities in the epithelium to maintain the daily sperm production rate, many cellular proteins, in particular those found at the Sertoli–Sertoli or Sertoli–germ cell interface, are being re-cycled [162]. Studies have shown that the relative ratio of ITT/cytokines within the micro-environment of the seminiferous epithelium may be crucial to govern these protein recycling events during the epithelial cycle [163]. These studies, while recently performed in rodents, can now be extended to humans by using the human Sertoli cell in vitro system [57].
Androgen Receptor (AR)
T exerts its effects through AR signaling [156, 164–166]. In humans, AR expression is restricted to Sertoli cells [167, 168]. Studies using murine models have shown that specific deletion of AR in Sertoli cells (SC-specific AR KO) leads to meiotic arrest and early spermatogenic maturation arrest [169, 170], and also terminal differentiation of haploid spermatids [171]. Collectively, these findings illustrate the significance of AR-mediated action in spermatogenesis, in particular murine meiosis and spermiogenesis. In men, the AR expression level visualized by immunohistochemistry in Sertoli cells was higher in those with NOA (non-obstructive azoospermia) vs. OA (obstructive azoospermia), and a significant positive correlation was observed between FSH levels and Sertoli cell AR expression in OA patients [167]. However, efforts to relate AR function or distribution to infertility in humans have been unsuccessful. For instance, no correlation was found between AR expression in Sertoli cells with serum T levels, or serum LH, and FSH levels in men with NOA [167]. Also, exogenous FSH was shown to trigger an increase in AR expression in the human testis [167]. However, hCG therapy had no apparent effect, thereby underscoring the significance of FSH-dependent Sertoli cell AR expression [167].
Exon 1 of the AR contains a CAG trinucleotide repeat that encodes a polyglutamine tract in the N-terminus of the AR. This region is required for AR interaction with transcriptional co-regulators, and variation in CAG repeat length, even within normal alleles, influences AR function in that shorter repeats are associated with a more transcriptionally active receptor. Furthermore, an expanded polyglutamine tract confers toxic properties responsible for neuronal and non-neuronal degeneration in the neurological disorder spinal and bulbar muscular atrophy in which affected men have small testes and gynecomastia (SBMA) [172]. Attempts to correlate the severity of spermatogenic dysfunction with CAG-encoded polyglutamine length polymorphism in the AR gene have, however, yielded inconsistent and conflicting results [173–176]. Interestingly, an insertion mutation near the beginning of the CAG repeat in exon 1 of the AR gene was found in an azoospermic man [177]. Treatment of an infertile man who had a point mutation of p.Val686Ala in the AR ligand-binding domain with prolonged high-dose testosterone therapy was found to produce marked improvement in sperm count and semen quality [178]. Combined with intracytoplasmic microinjection, testosterone treatment resulted in fertility. A point mutation in the transactivation domain (TAD) of the AR gene was found in an infertile man with gynecomastia with a high FSH level, small testes, and Sertoli cell-only syndrome [179]. However, a fertile man with gynecomastia was found to have a p.Pro69Ser mutation within the AR ligand-binding domain but to display no considerable defects in semen quality [180]. Taking these data collectively, it is anticipated that more AR mutation-associated male infertility cases will be identified. As more data are available, a systemic analysis is warranted to relate mutation(s) of the AR gene with idiopathic male infertility. At this point, based on the available data, it is likely that FSH-dependent AR expression is crucial for spermatogenesis. However, more accurate functional assays are necessary to pin-point the importance of AR in male infertility.
Estrogen
Testes are known to produce a considerable amount of estradiol-17ß via aromatase, which is essential to maintain spermatogenesis [10, 12, 181]. In humans, the role of estrogen in spermatogenesis remains a subject of debate. Emerging evidence, however, supports the notion that estrogen is involved in testis development, fluid resorption in the rete testis, maintenance of spermatogenesis, and in the maturation of spermatozoa [10, 181, 182]. Most importantly, aromatization of T to estradiol-17ß in the hypothalamus provides an important negative feedback signal to gonadotropin secretion [10]. Aromatase is expressed by human Leydig cells, Sertoli cells, spermatocytes, spermatids, and spermatozoa (its presence in spermatogonia remains unknown) [181] while estrogen is produced mostly by Leydig cells in the adult human testis [10].
Estrogen exerts its effects via estrogen receptors ERα (ESR1) and ERß (ESR2) [10, 12]. Studies in rodents have shown that ERα is expressed by Leydig cells and peritubular myoid cells; whereas ERß is found in some Leydig cells, but mostly in Sertoli cells and germ cells [183, 184]. In short, ERα is predominant in the interstitium and ERß in the seminiferous epithelium. Subsequent studies, however, have demonstrated that ERα is also expressed in Sertoli and germ cells in the rat testis [185]. In human testes, the binding of estradiol-17ß to human sperm was first reported in 1981 [186]. In 1998, human sperm were shown to express both the ERα mRNA and protein [187]. Subsequent studies have confirmed the presence of ERß as well as ERα in human sperm [12]. It is now generally accepted that ERα is expressed in spermatogonia, pachytene spermatocytes, and early round spermatids; whereas ERß is expressed in pachytene spermatocytes, early round spermatids, Sertoli cells, and Leydig cells in human testes [188, 189]. A genetic analysis study in 300 infertile Indian men and 255 fertile normal subjects identified single nucleotide polymorphisms (SNPs) (found in 4 subjects) and mutations (found in 8 subjects) in the ERß gene, suggesting ERß gene mutations are a cause of spermatogenesis failure in men. These infertile men displayed normal reproductive tract and serum hormone levels [190]. The few men identified with aromatase deficiency due to autosomal recessive inheritance of mutations in the CYP19A1 gene tend to have small testes and an abnormal semen analysis. Additionally, hormone levels in men with mutation in ERα and deficient in aromatase have considerable changes in serum T, estradiol, LH, and/or FSH compared to normal subjects (see Chap. 1). While the number of patients is small, these findings illustrate the significance of estrogen in human spermatogenesis. Furthermore, genetic and pharmacologically induced estrogen deficiency leads to reduced libido in men [191, 192]. In summary, estrogen is known to be involved in the development of the testis, maintenance of male reproductive tract, fluid resorption in the rete testis, and in particular sperm maturation in humans [12, 181, 193, 194].
Small Non-coding RNAs (sncRNAs)
As noted above, spermatogenesis is a highly complicated process that requires the intriguing participation of multiple genes at the transcriptional and post-transcriptional levels to produce spermatozoa. Studies have shown that germ cells, including spermatogonia, spermatocytes, and post-meiotic spermatids all contain abundant levels of non-coding RNAs, such as siRNA (small interfering RNA, 20–25 nucleotides), miRNA (microRNA, 21–24 nucleotides), and piRNAs (Piwi-interacting RNA, 26–31 nucleotides) [195–197]. The most important sncRNAs that are involved in spermatogenesis are miRNAs and piRNAs and the associated pathways based on studies of genetic models in rodents [198–201]. These small RNAs are short single-stranded non-coding nucleotides that are known to directly disrupt target mRNAs through degradation, causing translation repression to block protein synthesis [20, 202]. Since miRNA can bind to more than one mRNA, a specific miRNA can regulate the function of more than a single gene. Small RNAs including miRNAs and piRNA are mostly stored in the chromatoid body which is a dense structure in the germ cell cytosol composed of mainly RNAs and RNA-binding proteins and are involved in the regulation of germ cell apoptosis, proliferation, and differentiation, as well as spermatogonial stem cell self-renewal [200, 203, 204]. Studies have shown an alteration in the expression patterns of small RNAs in infertile men [205–207]. For instance, miR-141, miR-429, and miR-7-1-3p were up-regulated in men with idiopathic non-obstructive azoospermia (NOA) [206] while miR-34c-5p, miR-122, miR-146b-5p, miR-181a, miR-374b, miR-509-5p, and miR-513a-5p were markedly down-regulated in men with NOA. These miRNAs are possibly involved in regulating germ cell apoptosis [207].
In this context, it is of interest to note that miRNAs and siRNAs are processed by an RNase III endonuclease Dicer, the deletion of which in mice leads to infertility. Dicer is essential for haploid spermatid differentiation [208] and for the assembly and maintenance of cell junctions in the seminiferous epithelium during spermatogenesis [209]. Specific deletion of Dicer in Sertoli cells also leads to infertility with complete absence of spermatozoa in seminiferous tubules and progressive testicular degeneration [210]. While the role of Dicer in human fertility remains to be elucidated, it is obvious that small regulatory RNAs represent a tempting target for non-hormonal male contraception. Also, much work is needed to better understand the role of small RNAs in human spermatogenesis, and their use as diagnostic markers to monitor infertility, for example, men who are at risk because of industrial exposure to toxicant-induced infertility.
Obesity
According to NIH guidelines (see http://www.nhlbi.nih.gov/health/health-topics/topics/obe/diagnosis), adults with a body mass index (BMI) >30 (kg/m2) are obese. Based on the latest statistics in 2014, the obese adult population in the U.S. is at 27.7% (http://www.gallup.com/poll/181271/obesity-rate-inches-2014.aspx), which is double the world’s average of 13% (http://www.who.int/mediacentre/factsheets/fs311/en/), illustrating an alarming trend given the health risks associated with obesity. A lower sex hormone binding globulin (SHBG) level is found in men with obesity and/or diabetes, which in turn lowers the total testosterone level (see Chap. 16 and [211]). Emerging evidence suggests a negative correlation between rising BMI and sperm count, sperm concentration, and motile sperm, which impedes male fertility [212–214]. Interestingly, while other studies suggest that obesity has no major impact on fertility, semen quality, gonadotropin levels, or other sperm parameters despite reduced testosterone levels [215, 216]. The reason(s) behind the different conclusions is not immediately known. Nonetheless, studies have demonstrated possible mechanism(s) by which obesity might lead to reduced spermatogenesis capacity and daily sperm output, as well as reduced sperm count and sperm quality. One proposed mechanism is hyperestrogenism in which serum estrogens are considerably higher in obese men due to an increase in peripheral conversion of testosterone to estrogens by aromatase in adipose tissue [217–219], coupled with a reduced testosterone production [214]. The excessive estrogens in the systemic circulation thus inhibit the release of LH and FSH from the pituitary gland via the negative feedback on the hypothalamus, thereby reducing T production, intratesticular T levels, and the testosterone/estrogen ratio. The reduced T and FSH levels result in suppressed spermatogenesis. However, the correlation between high levels of estradiol and obesity is controversial and is further affected by low SHBG [220], suggesting the need for additional studies. Nonetheless, this mechanism is supported by findings that obese men have considerably lower levels of inhibin B than healthy men [221–223]. Elevated levels of cytokines are another potential cause of hypospermatogenesis in obese men [224]. Other mechanisms that might cause defects in human spermatogenesis in obesity include reduced levels of SHBG, insulin and leptin resistance, sleep apnea, and adiponectin deficiency [225–229]. Additionally, factors involved in the pathogenesis of obesity, such as high calorie diet, genetic, and epigenetic disorders, might also play a role in perturbing sperm production [230, 231].
Besides the disruptive effects of obesity on spermatogenesis, there is emerging evidence that obesity also affects the molecular structure of testicular germ cells and mature spermatozoa, such as an impairment of acrosome reaction [232], leading to altered growth in offspring, increased susceptibility to disease in adults [233], and erectile dysfunction [234]. Studies also suggest that high-fat diets can affect the epigenetic content of sperm or the endocrine content of seminal fluid, which in turn affects early fetal development [213].
Bariatric surgery [235], such as Roux-en-Y gastric bypass surgery [236], has been reported to increase T levels and improve sexual function in obese men. Much of the increase in T, however, results from an increase in the level of SHBG [236]. Interestingly, there are case reports in which bariatric surgery was followed by impaired semen parameters, possibly by perturbing absorption of vitamins and trace elements [237, 238]. However, one study of six men showed no disruptive changes of bariatric surgery on semen parameters, coupled with an increase in urinary total T levels [239].
Summary
Spermatogenesis is a series of cellular events that take place in the seminiferous tubules of the testis. In this review, we provide a brief overview of human spermatogenesis, from spermatogonial self-renewal via mitosis, meiosis, post-meiotic spermatid development via spermiogenesis, to the release of sperm at spermiation. We discuss the role of Sertoli cell and especially the role of the BTB in spermatogenesis. We also highlight some specific areas of research that deserve future attention. Due to the lack of human testis samples for analysis, in particular those from normal subjects, the study of human spermatogenesis lags far behind studies in rodents. However, human Sertoli cells obtained at biopsy can now be cultured in vitro and maintained up to weeks and months where they remain mitotically active. These cells can be subcultured for creative experiments. We also briefly discuss some emerging fields of research that focus on factors affecting human spermatogenesis. These factors may deserve more attention by investigators in future years.