Glucagon and Glucagon-like Peptides
Joel F. Habener
Timothy J. Kieffer
Investigations during the past 15 years have uncovered a remarkable, unanticipated complexity in the repertoire of biologically active hormones produced by the expression of the proglucagon gene. Because of these new discoveries, an entirely new perspective has arisen regarding the biologic functions and importance of proglucagon-derived peptides in the pathogenesis of diabetes mellitus. The structure of the proglucagon gene, established by DNA cloning approaches, reveals that it encodes a large preproglucagon protein consisting of an N-terminal signal sequence and a prohormone encoding glucagon and several glucagon-like peptides (GLPs). The signal sequence is removed from the prohormone during its synthesis so that the initial completed protein synthesized is the prohormone referred to as proglucagon. The structure of proglucagon is representative of prohormones that encode peptide hormones inasmuch as it contains multiple biologically active hormones that are released from the prohormone by tissue-specific proteolytic cleavages. Proglucagon undergoes alternative posttranslational cleavages whereby the hormone glucagon is produced in the α-cells of the endocrine pancreas (islets of Langerhans) and glucagon-like peptides are produced in the L cells of the intestine. Glucagon and the glucagon-like peptides are all members of a superfamily of peptides structurally related to glucagon, including vasoactive intestinal peptide, secretin, gastric inhibitory polypeptide, and growth hormone-releasing hormone, among others. Each of these glucagon-related peptide hormones is involved to some extent in the regulation of nutrient homeostasis, growth, or development, acting via G-protein-coupled receptors that increase the formation of cyclic adenosine monophosphate (cAMP). Glucagon is secreted from α-cells and functions predominantly during the fasting state to maintain blood glucose levels by the mobilization of glucose from glycogen stores in peripheral tissues such as muscle and liver. Excessive production of glucagon contributes to hyperglycemia, and thus approaches that antagonize glucagon action in subjects with diabetes mellitus are being actively investigated. The glucagon-like peptides are secreted in response to feeding. Glucagon-like peptide-1 (GLP-1) and gastric inhibitory polypeptide (GIP) comprise the intestinal “incretin” hormones released from the intestine in response to feeding and augment glucose-stimulated insulin secretion from the β-cells of the islets of Langerhans. GLP-1 also enhances insulin-stimulated glucose uptake in peripheral tissues (muscle, fat, liver), suppresses glucagon secretion, induces satiety, and promotes the growth and differentiation of new β-cells in the pancreas. These antidiabetic properties of GLP-1 have prompted considerable interest in the therapeutic potential of GLP-1 for the treatment of diabetes mellitus. GLP-2 is a potent growth factor for intestinal epithelium and holds promise for the treatment of inflammatory bowel diseases. This chapter provides an overview of the historical advances in the understanding of the biologic and physiologic roles of these proglucagon-derived peptides and their potential importance in the pathogenesis of diabetes mellitus. A recent review describes the biology of glucagon and the GLPs (1). Additional review articles describe the biology of the GLPs in detail (2,3,4,5,6,7,8,9,10).
EXPRESSION OF THE PROGLUCAGON GENE
Tissue Distribution
The expression of the proglucagon gene (chromosome 2q36-q37 in humans) is restricted to a limited number of tissues. So far, proglucagon or proglucagon messenger RNA (mRNA) has been found in the α-cells of the endocrine pancreas, L cells in the distal small intestine and colon, and localized regions of the brain (hypothalamus and nucleus of the solitary tract). Current evidence indicates that the regulation of expression of the proglucagon gene takes place at two levels of expression: posttranslational processing of proglucagon and the transcription of the preproglucagon gene.
Regulation
ALTERNATIVE POSTTRANSLATIONAL PROCESSING
Proglucagon, synthesized in the α-cells of the islets, is processed by specific proteolytic cleavages in a pattern that differs markedly from the processing in the L cells in the intestine and the peptidergic neurons in the brain (Fig. 11.1). In the α-cells, specific prohormone convertases cleave and liberate glucagon from proglucagon, leaving behind the presumably biologically inert “major proglucagon fragment” and the N-terminal fragment of proglucagon (11). In contrast, in L cells and in peptidergic neurons, cleavages of proglucagon by a different set of prohormone-converting enzymes results predominantly in the formation of GLPs comprising the GLP-1 group of isopeptides and GLP-2.
Much of the new interest and excitement in the field of discovery research on the hormone glucagon produced by the α-cells of the islets of Langerhans arises from the findings that the precursor prohormone, proglucagon, also encodes the GLPs and that these peptides have unanticipated novel biologic activities in the regulation of nutrient metabolism, the growth and differentiation of insulin-producing β-cells of the pancreas, the control of appetite, and the promotion of the growth of intestinal epithelium (5,6,12). These recent findings on the actions of GLPs encoded by the preproglucagon gene have prompted great interest in the pursuance of the biochemistry and mechanism of actions of the GLPs.
The proteolytic cleavages—so-called posttranslational processing—of proglucagon that result in the formation of GLPs are somewhat complex. At least six GLP-1 isopeptides are formed: the largest peptides of 37 and 36 amino acids, C-terminal glycine-extended GLP-1(1–37) and C-terminal-amidated GLP-1(1–36)amide; and the N-terminal-truncated GLP-1s, GLP-1(7–37), GLP-1(7–36)amide, GLP-1(9–37), and GLP-1 (9–36)amide. So far, it appears that the biologic activities of the GLP-1s reside in the peptides GLP-1(7–37) and GLP-1 (7–36)amide; the two peptides have indistinguishable activities. The 1–37/36 and 7–37/36 peptides are cleaved from proglucagon by the actions of the prohormone convertases PC1/3 and PC2, whereas the 9–37/36 peptides are derived from the former peptides by cleavage of two N-terminal amino acids by the enzyme dipeptidyl peptidase IV (DPP-IV), also known as CD26 (13,14). DPP-IV is a ubiquitous enzyme expressed in many tissues and carried in the circulation. Cleavages of the GLP-1s by DPP IV occur at the time of their secretion from the intestinal L cells and within the circulation (15). Notably, the 9–37/36 peptides bind to the GLP-1 receptor but are biologically inactive and are weak antagonists. Most of the GLP-1 in the circulation consists of the apparently inert 1–37/36 and 9–37/36 peptides with no known biologic activities heretofore.
TRANSCRIPTION
A second level of the regulation of the expression of the GLPs is that of gene transcription. The preproglucagon gene consists of a transcribed sequence (Fig. 11.2) and a 5′-flanking promoter sequence (Fig. 11.3). The transcribed sequence in turn consists of six exons separated by five introns (16). Notably, each of the glucagon peptides—glucagon, GLP-1, and GLP-2—are encoded in separate exons (exons 3, 4, and 5), suggesting the occurrence of exonic duplication during evolutionary history (7). Although the peptides are encoded in separate exons, unlike many multiexonic
genes, the expression of these genes in mammals is not regulated by alternative exon splicing of the RNA; preproglucagons in both pancreas and intestine appear to be translated from identical mRNAs. However, in lower vertebrates (chickens, fish, and frogs), alternative splicing of exons does occur. The promoter of the preproglucagon gene is bipartite with respect to regions responsible for expression in pancreatic α-cells and intestinal L cells. Promoter sequences within 700 base pairs 5′ flanking the initiation of transcription are sufficient to direct the faithful expression of the preproglucagon gene in α-cells, whereas sequences required for expression in L cells reside more 5′ between nucleotides -3000 to -1000 (5,16,17). The sequences of these regulatory regions of the promoter contain multiple cis -acting control elements that bind proteins termed “transcription factors” that are responsible for activating the transcription of the gene via interactions with the basal promoter consisting of RNA polymerase in association with basal transcription factors. The cis -acting control elements and DNA-binding transcription factors responsible for the regulation of expression of the preproglucagon gene in α-cells are much better understood than are those in L cells. The α-cell-specific expression region of the promoter contains at least six clearly identified regions residing within the proximal 300 base pair of the promoter (Fig. 11.3) (16). These regions or elements are designated G1, G2, G3, G4, C/EBP, and CRE (cAMP response element). Each of these elements binds multiple protein complexes consisting of both relatively α-cell-specific proteins and tissue-ubiquitous proteins. These proteins consist of members of the paired homeodomain (Pax), basic helix-loop-helix (bHLH), POU homeodomain, basic leucine zipper (bZip), and hepatocyte nuclear factor (HNF) families of transcription factors. The G1 region of the promoter has been shown to lend
α-cell specificity to the expression of the gene (18). The way nutrient signals and hormones regulate the transcription of the proglucagon gene is not completely understood. However, there is convincing evidence that cAMP and insulin signaling are mediated via the CRE and G3 elements of the proglucagon gene promoter, respectively (19,20).
genes, the expression of these genes in mammals is not regulated by alternative exon splicing of the RNA; preproglucagons in both pancreas and intestine appear to be translated from identical mRNAs. However, in lower vertebrates (chickens, fish, and frogs), alternative splicing of exons does occur. The promoter of the preproglucagon gene is bipartite with respect to regions responsible for expression in pancreatic α-cells and intestinal L cells. Promoter sequences within 700 base pairs 5′ flanking the initiation of transcription are sufficient to direct the faithful expression of the preproglucagon gene in α-cells, whereas sequences required for expression in L cells reside more 5′ between nucleotides -3000 to -1000 (5,16,17). The sequences of these regulatory regions of the promoter contain multiple cis -acting control elements that bind proteins termed “transcription factors” that are responsible for activating the transcription of the gene via interactions with the basal promoter consisting of RNA polymerase in association with basal transcription factors. The cis -acting control elements and DNA-binding transcription factors responsible for the regulation of expression of the preproglucagon gene in α-cells are much better understood than are those in L cells. The α-cell-specific expression region of the promoter contains at least six clearly identified regions residing within the proximal 300 base pair of the promoter (Fig. 11.3) (16). These regions or elements are designated G1, G2, G3, G4, C/EBP, and CRE (cAMP response element). Each of these elements binds multiple protein complexes consisting of both relatively α-cell-specific proteins and tissue-ubiquitous proteins. These proteins consist of members of the paired homeodomain (Pax), basic helix-loop-helix (bHLH), POU homeodomain, basic leucine zipper (bZip), and hepatocyte nuclear factor (HNF) families of transcription factors. The G1 region of the promoter has been shown to lend
α-cell specificity to the expression of the gene (18). The way nutrient signals and hormones regulate the transcription of the proglucagon gene is not completely understood. However, there is convincing evidence that cAMP and insulin signaling are mediated via the CRE and G3 elements of the proglucagon gene promoter, respectively (19,20).
![]() Figure 11.2. Diagram of proglucagon gene and encoded messenger RNA (mRNA). The gene consists of six exons, E1 to E6, and five introns, IA to IE. The exons encode functional domains of the preproglucagon. The pairs of basic residues that serve as posttranslational sites of processing of the preproglucagon encoded by the mRNA are shown to key in with Figure 11.1. S, signal peptide; N, amino-terminal sequence of proglucagon; Gluc, glucagon; GLP, glucagon-like peptides; IP, intervening peptides; M, methionine encoded by AUG codon that initiates translation; Q, glutamine; H, histidine; and UN-TX, untranslated regions of mRNA. (From Mojsov S, Heinrich G, Wilson IB, et al. Preproglucagon gene expression in pancreas and intestine diversifies at the level of post-translational processing. J Biol Chem 1986;261:11880–11889, with permission. Copyright © 1986 by The American Society for Biochemistry and Molecular Biology.) |
Superfamily of Glucagon-Related Peptide Hormones
The glucagon superfamily of peptide hormones are so designated because of their close similarities in amino acid sequences (Fig. 11.4). They have a diverse number of functions consisting in part of neurotransmitters, growth factors, and regulators of nutrient metabolism. Of these hormones, GLP-1 has the highest degree of similarity to glucagon (48%). The amino acid sequences of the glucagons and GLP-1s have been highly conserved throughout evolution; the human and anglerfish glucagons and GLP-1s are 75% and 79% identical, respectively (5,7). This strong conservation of sequences over 300 million years of evolutionary time attests to the importance of these hormones in physiologic processes.
GLUCAGON
History
In their early studies (1921), Banting and Best (21) suspected the existence of a hyperglycemia-producing hormone in the pancreas. They observed that dogs administered insulin-enriched pancreatic extracts had a biphasic blood glucose response: There was an initial rise in blood glucose followed by a lowering of blood glucose attributable to the expected actions of insulin. They suspected that the initial increase in glucose level was due to a “contaminant” in the crude pancreas extracts, which was later shown to be glucagon. In 1957, Bromer et al. (22) determined the amino acid composition and partial sequence of glucagon and determined that it was a peptide of 29 amino acids. The availability of pure crystalline glucagon led to the establishment of its hyperglycemic properties via the stimulation of glycogenolysis (23) and gluconeogenesis (24) in liver and skeletal muscle and of lipolysis in fat (25). The seminal studies of Sutherland and DeDuve (26) demonstrated that the glycogenolytic and lipolytic actions of glucagon in liver and fat, respectively, were mediated by the stimulation of the formation of the cellular “second messenger” cAMP. The development by Unger et al. (27) of a radioimmunoassay specific for the detection of glucagon in blood plasma paved the way for numerous cellular and physiologic studies showing that glucagon is a hormone critical for the maintenance of blood glucose levels during fasting (28,29). Furthermore, it was soon established that individuals with diabetes have inappropriately elevated levels of glucagon in the circulation, thereby potentially worsening the physiologic derangements of diabetes by driving the overproduction of glucose by the liver (i.e., increasing hepatic glucose output, and raising blood glucose levels) (28,29). The access to a glucagon-specific antiserum and resultant radioimmunoassay also led to the definitive identification of the α-cells of the pancreatic islets as the source of the production of glucagon; the identification of large, immunoreactive, forms of glucagon in the circulation, known then as glucagon-like immunoactivities (GLIs); and the expression of proglucagons in the L cells of the intestine (1).
Chemistry and Structure of Glucagon
Glucagon was crystallized in 1953 (30), and its amino acid sequence was determined in 1957 (22). X-ray analyses of crystallized glucagon provide a tentative structure of the hormone, in which the N-terminal and C-terminal groups consist of α-helices and are in close proximity by virtue of a “hairpin” bend in the molecule (31). However, it is uncertain whether glucagon in solution or bound to its receptor assumes the same secondary structure as it displays in a crystal. Solution nuclear magnetic resonance (NMR) studies are consistent with a largely unstructured and flexible chain (32). Both in crystals and in solution at high concentrations, glucagon self-aggregates in the form of trimers (32). Structure-function studies of N-terminal- and C-terminal-truncated glucagon peptides provide relatively consistent findings. The N-terminal domain of the hormone is critical for the activation of the cAMP-mediated signal transduction pathway, whereas the C-terminal domain is required for high-affinity binding to the glucagon receptor (33).
The N-terminal histidine residue, His1 and Asp9 and Ser16, appears to be particularly important for both receptor binding and the activation of cAMP formation (34,35). Removal of the N-terminal histidine and modification of Asp9 result in the formation of a partial antagonist of glucagon actions.
The N-terminal histidine residue, His1 and Asp9 and Ser16, appears to be particularly important for both receptor binding and the activation of cAMP formation (34,35). Removal of the N-terminal histidine and modification of Asp9 result in the formation of a partial antagonist of glucagon actions.
Ontogeny of Pancreatic α-Cells
Although α-cells were identified as being histologically distinct from β-cells in the islets of Langerhans as early as 1907 (36), not until 1962 were α-cells shown to be the source of glucagon (37). The α-cells are one of four recognized hormone-producing cell types in the islets, which also include insulin-producing β-cells, somatostatin-producing δ-cells, and the pancreatic-polypeptide-producing PP cells. In the islets of rodents, the α- and δ-cells are located on the surface, or mantle, of the spherical shaped islets, whereas the β-cells comprise the core of the islets. Glucagon is believed to be the earliest endocrine pancreatic hormone expressed during embryonic development of the pancreas—preceding the expression of insulin (38). The pancreas originates by a dual (dorsal and ventral) outbudding of a specialized endodermal epithelium located in the anterior region of the developing gut tube of the embryonic (E) mouse at day 9 to 9.5 in the mouse (39). Proglucagon mRNA and glucagon immunoreactive positive cells are detected in the early pancreatic buds. From days E10 to E12, the buds undergo branching morphogenesis, forming a ductal tree containing clusters of endocrine cells that express glucagon, insulin, neuropeptide Y, and somatostatin. Between days E13 and E14, a major transition occurs in which the exocrine acinar tissue and aggregating endocrine cells separate into distinct compartments. By days E17 to E19, the morphologic development of the pancreas is essentially completed as the islets condense into spherical structures embedded in the exocrine acinar tissue. For 3 to 6 weeks after birth, the endocrine pancreas undergoes considerable remodeling, as the rate of formation of new endocrine cells by proliferation and differentiation from islet progenitor cells located in the ducts remains high and is associated with comparable rates of apoptosis of existing older endocrine cells.
Regulation of Glucagon Secretion
In the absence of ketosis, glucose is the obligate fuel used by the brain (40). Because the brain cannot synthesize glucose and only has enough glycogen stores to last a few minutes, the glucose required by the brain to maintain viability must be provided by the circulation. The importance of the maintenance of adequate blood glucose levels has resulted in the evolution of insulin counterregulatory hormones, among which glucagon is critical. Impaired formation and action of glucagon results in hypoglycemia. Insulin and glucagon are physiologic antagonists; insulin removes glucose from the circulation by stimulating uptake of glucose into liver, muscle, and fat during meals, whereas glucagon stimulates the formation and release of glucose into the circulation, particularly by the liver. Thus, in direct contrast to insulin secretion, glucagon secretion is increased during periods of fasting and is suppressed by elevated plasma levels of glucose. The balanced counteractions of insulin and glucagon maintain blood glucose levels in a relatively narrow range (∼120 mg/dL during feeding and 60 mg/dL during fasting).
The most effective regulator of glucagon secretion is glucose, which suppresses glucagon secretion. However, certain amino acids produced by the digestion of proteins, such as arginine, stimulate glucagon secretion. Notably, carnivores, such as canines, have α-cells in their stomachs, suggesting an adaptive mechanism to provide glucagon early during the digestion of a high-protein meal to defend against insulin-induced hypoglycemia (41). Whether orally ingested fat affects glucagon secretion is unclear, although elevated plasma levels of free fatty acids reduce plasma concentrations of glucagon and inhibit the release of glucagon from the perfused rat pancreas (42). Several hormones regulate α-cell functions and coordinate the secretion of glucagon during changes in nutrient availability. These include the gastrointestinal hormones GLP-1 (5) and GIP (43) and the pancreatic-islet hormones insulin and somatostatin (44). In conditions of metabolic stress, such as hypoglycemia, additional hormones are released (e.g., growth hormone, catecholamines, glucocorticoids, and endorphins) all of which augment the secretion and actions of glucagon on peripheral tissues to ensure the maintenance of adequate blood glucose levels. Glucopenic stress also activates parasympathetic autonomic neural input to the islets that enhances glucagon secretion (45).
The intracellular signaling mechanisms within α-cells that culminate in the secretion of glucagon are complex. In situ hybridization studies of rat α-cells have shown the presence of the mRNAs encoding the KATP channel subunits Kir6.2 and SUR1 (46). Electrophysiologic studies of mouse α-cells in intact islets have determined that, although the expression of ion channels on α-cells is somewhat similar to that of β-cells and δ-cells, responses to glucose differ. α-Cells are distinguished from β-cells and δ-cells by the presence of a large tetrodotoxin-sensitive Na+ current, a triethylamine-resistant K+ current, and two kinetically separable Ca2+ currents: low- (T-type) and high-threshold (L-type) Ca2+ channels. In contrast to β-cells, α-cells are electrically silent in the presence of insulin-releasing glucose concentrations. The action potentials generated in the absence of glucose are inhibited by tetrodotoxin, nifedipine, and tolbutamide (47). These findings suggest that the electrical activity in and secretion of glucagon from α-cells is dependent on the generation of Na+-dependent action potentials. Furthermore, the KATP channel opener diazoxide inhibits the electrical activity and increases the whole-cell K+ conductance leading to glucagon secretion (46). Thus, glucagon secretion depends on a low activity of KATP to maintain sufficient negativity of membrane potential to prevent voltage-dependent inactivation of voltage-gated membrane currents. It was postulated that glucose inhibits glucagon release by depolarizing the α-cell, with resultant inactivation of the ion channels that participate in the generation of action potentials (47).
Metabolism and Degradation of Glucagon
Glucagon is cleared relatively rapidly from the circulation, with a half-life of about 5 minutes [metabolic clearance rate (MCR) ∼10 mL · kg-1 · min-1] (48). Both the kidney and the liver remove glucagon from the circulation, accounting for 30% and 20% of disposal, respectively. Notably, the remaining 50% of glucagon is destroyed in the circulation by enzymes, including serine and cysteine proteases, cathepsin B, and primarily DPP-IV, which cleave glucagon into proteolytic fragments. Not all cleavages of glucagon are in the pathway for its disposal. Cleavage after arginines 17 and 18 by an endopeptidase isolated from rat liver membranes results in the formation of glucagon 19–29, so-called miniglucagon (49). Remarkably, miniglucagon was found to be a highly potent activator of Ca2+ channels in heart and liver cells (49). In heart cells it stimulates the accumulation of Ca2+ into sarcoplasmic reticular stores, which are targets for Ca2+-induced Ca2+ release by glucagon. Unlike glucagon, miniglucagon does not activate adenylyl cyclase. The cAMP-independent actions of miniglucagon in heart cells are mediated by the release of arachidonic acid, which acts synergistically with glucagon-induced
formation of cAMP to trigger inotropic responses (50). There is also evidence that miniglucagon can inhibit glucose-induced insulin secretion from β-cells at picomolar levels (51). The physiologic roles of other potentially biologically relevant truncated glucagon peptides remain to be elucidated.
formation of cAMP to trigger inotropic responses (50). There is also evidence that miniglucagon can inhibit glucose-induced insulin secretion from β-cells at picomolar levels (51). The physiologic roles of other potentially biologically relevant truncated glucagon peptides remain to be elucidated.
Physiologic Actions of Glucagon
The most important physiologic action of glucagon occurs during the postabsorptive and fasting states. Through its actions on key enzymes, glucagon induces glycogenolysis, gluconeogenesis, and ketogenesis by the liver, and, to some extent, lipolysis in adipose tissue and glycogenolysis in muscle to mobilize stored energy. In the fasting state, the liver is the essential organ that provides glucose fuel to the brain. In humans the brain requires (utilizes) ∼6 g of glucose per hour, whereas all other tissues utilize ∼4 g of glucose per hour in the resting state. Collectively, the liver must provide ∼10 g of glucose per hour to maintain euglycemia. The actions of glucagon on the liver account for ∼75% of the glucose production in the fasting state. Additional fuel is derived from the mobilization of fatty acids from adipose tissue metabolized in the liver to ketone bodies. This process is particularly important during periods of stress or starvation, as these ketones can be used by tissues, particularly the brain, to generate ATP. Other important mediators of this response include catecholamines, growth hormone, and glucocorticoids. The central nervous system senses glucopenia and, in response, triggers neural-sympathoadrenal hormones to counteract hypoglycemia. The ventromedial hypothalamus is proposed to be an important sensor of hypoglycemia and to initiate neural afferent signals to stimulate counterregulatory responses by way of the secretion of catecholamines, growth hormone, and glucocorticoids (52,53).
Glucagon is a major contributor to the immediate responses of the “fight-or-flight” circumstance. During times of sudden and intense physical effort, an instant increase in fuel for skeletal muscle is required without a decrease in fuel delivery to the brain. The skeletal muscles contain a limited supply of glycogen and lipids that can provide energy for the muscles for a short time. Sustained muscular activity requires an increase in circulating glucose and free fatty acids. Catecholamines play a critical role in these fight-or-flight circumstances during which increased fuel in the form of glucose and free fatty acids must be delivered to the circulation. Catecholamines stimulate glucagon secretion and reduce insulin levels during periods of stress and exercise. Notably, the reduction in insulin levels does not limit glucose uptake by exercising skeletal muscle but rather curtails glucose uptake by liver and fat, thereby protecting skeletal muscle from potential deleterious effects of a lowering of blood glucose levels. The increase in glucagon levels, stimulated by increased catecholamines, drives increased glycogenolysis and gluconeogenesis in liver and lipolysis in fat to further augment circulating levels of glucose and free fatty acids.
Mechanisms of Glucagon Action
The glucagon receptor belongs to group IV of the “B” family of seven membrane-spanning G-protein-coupled receptors (54). This family of receptors includes those for the hormones glucagon, GLP-1, GLP-2, GIP, vasoactive intestinal peptide (VIP), pituitary adenylyl activating peptide (PACAP), secretin, calcitonin, parathyroid hormone (PTH), and growth hormone releasing-hormone (GRH). A common feature of the glucagon receptor and these structurally related receptors is their coupling to the G-protein, Gs, that activates adenylyl cyclase and the resultant production of cellular cAMP. A major component of the cAMP-dependent signaling pathway is the activation of protein kinase A (PKA), an enzyme that phosphorylates and activates the functions of many different proteins in cells (55). PKA can activate proteins in the mitogen-activated protein kinase pathways by phosphorylation of Rap-1 that activates B-Raf and the downstream targets MEKKs (MAPK/ERK kinases), including ERK P-42, and ERK P-44. In addition, cAMP can bind directly to and activate a group of cellular mediators known as GEFs (guanine exchange factors). These additional pathways activate phosphoinositol 3-kinase and release of intracellular stores of Ca2+. Thus, the intracellular signaling pathways mediated by the actions of glucagon on its target tissues are highly complex.
The expression of the glucagon receptor has been demonstrated in a large number of different tissues, including not only the generally recognized target tissues of liver, fat, and muscle but also the kidney, heart, lung, brain, intestine, adrenal gland, spleen, ovary, thyroid (56), and the pancreatic islet α-, β-, and δ-cells (57). On the basis of these findings, it seems certain that glucagon has wide-ranging metabolic actions on the functions of many organs in the body. Within the pancreatic islets, the glucagon receptor is expressed in most β-cells and on a substantial subpopulation of the α- and δ-cells (57). It has been suggested that the glucagon receptors on β-cells may stimulate small amounts of insulin during periods of fasting to maintain the β-cells in a primed state awaiting a nutrient challenge and also may provide some insulin to peripheral tissues to facilitate glucose uptake during fasting (57).
Notably, the actions of glucagon may be regulated at the level of the expression of the glucagon receptor. In rats, expression of glucagon receptor in brown adipose tissue is downregulated following exposure to cold under the control of the sympathetic nervous system (58). The hepatic expression of glucagon receptor increases progressively from the first day of life to the adult stage in rodents. However, the expression can be increased under conditions in which intrahepatic glucose metabolism is activated, such as during fasting or in diabetes (59). Glucagon, acting through increased cellular levels of cAMP, is capable of downregulating the expression of hepatocyte glucagon receptors (60). Therefore, regulation at the level of the expression of receptors appears to be another means by which glucagon actions are modulated.
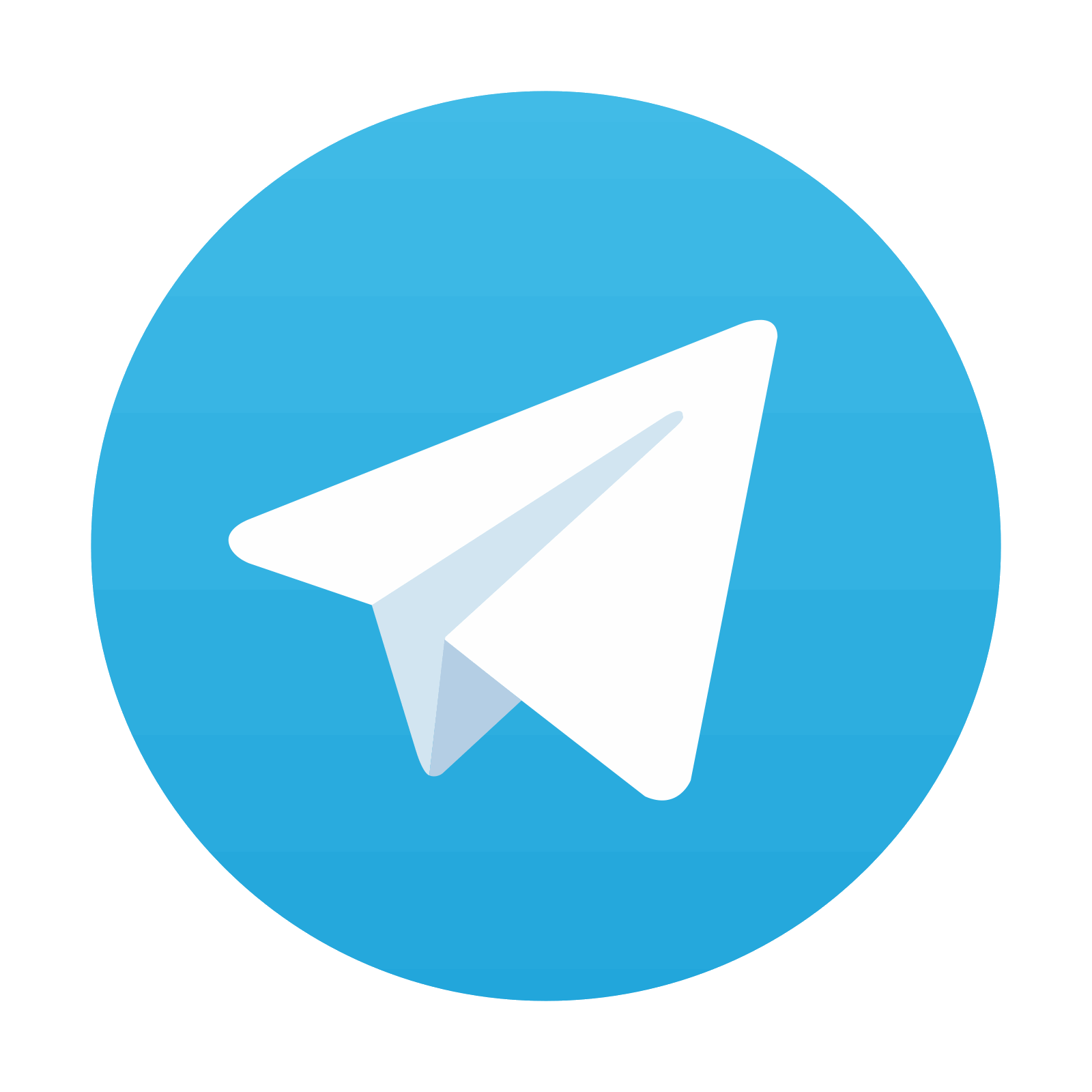
Stay updated, free articles. Join our Telegram channel
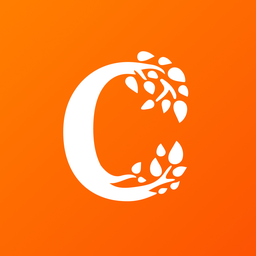
Full access? Get Clinical Tree
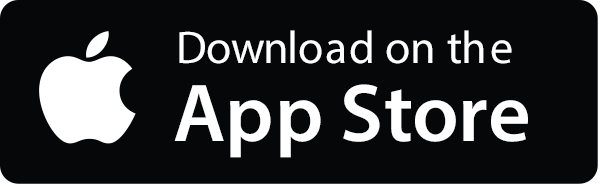
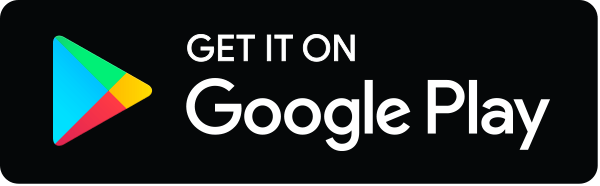