Genomic and Nongenomic Actions of Thyroid Hormones
Paul M. Yen
Gregory A. Brent
Thyroid hormones (THs) (e.g., L-triiodothyronine, T3; L-tetraiodothyronine, T4) regulate a wide range of processes including growth, brain development, and metabolism (1,2). The critical role of TH in human development is demonstrated by the distinct neurological and physical deficits that occur in iodine-deficient areas when both the mother and developing fetus are hypothyroid (3,4). TH also has important effects on growth and development in the latter stages of childhood. Thyroid deficiency in early brain development is largely irreversible, but most manifestations of hypothyroidism in the adult are reversible with TH treatment (5,6). In adults, TH primarily regulates metabolism, although there are important actions on mood, cognition, the heart, muscle, and bone. The metabolic actions of TH include regulation of oxygen consumption and protein, carbohydrate, lipid, and vitamin metabolism (7,8). The many clinical manifestations of hypo- and hyperthyroidism, TH excess and deficiency, reflect the wide range of TH effects on various pathways and target organs.
TH regulates metabolic rate through actions on uncoupling of oxidative phosphorylation, stimulation of energy expenditure by the activation of Na+-K+ adenosine triphosphatase (ATPase) activity, and direct modulation of TH transporters and enzymes in the plasma membrane and mitochondria (9,10). TH also interacts with other key metabolic pathways regulating fatty acid synthesis and fatty acid oxidation (7,8,11). TH action is mediated by nuclear receptors with high affinity for TH [(Kd (dissociation constant) approximately 10-10 M for T3)] (1). The receptor-binding affinity of various THs and analogs generally correlate with their biologic potencies, suggesting that most biologic effects are mediated by the TH nuclear receptor (12). TH has some action at nongenomic sites such as the plasma membrane, cytoplasm, and mitochondria (1,13,14).
The TH exerts its nuclear actions through thyroid hormone receptors (TRs) alpha and beta, which bind to DNA regulatory elements in TH-responsive genes. There are common elements to the nuclear action of TH, as shown, although there is some variation by tissue (Fig. 8.1). Circulating free TH enters the cell, in some tissues, such as brain, via plasma membrane transporters. There are a number of thyroid transporters, including MCT8, MCT10, and Oat1C1, and their roles are being elucidated (15,16,17,18). The importance of transporters may also vary according to species, since a significant functional role of MCT8 has been found for the brain in man and a less prominent one in mouse. In addition to transport of T3 from the circulation into the cell, in some cells T3 may be converted intracellularly from circulating serum T4 by iodothyronine 5′-deiodinase Type 2 (D2) (19,20). T3 then enters the nucleus, where it binds to the nuclear TRs with high affinity and specificity. TR is found primarily in the nucleus, although there is also some TR in the cytoplasm. Cytoplasmic TR does not bind to heat shock proteins, as is seen for Type 1 nuclear receptors, such as estrogen and progesterone receptors. TR is a ligand-regulated transcription factor that is intimately associated with chromatin and heterodimerizes with another member of the nuclear receptor superfamily, retinoid X receptor (RXR) (1,21). TR/RXR, in turn, is bound to target DNA sequences known as TH-response elements (TREs), located in the promoter regions of target genes (22). In the absence of the ligand, the TR/DNA complex binds to a positive TRE (i.e., a TRE that confers stimulation of gene expression by TH), recruits corepressor, and results in gene repression. The addition of ligand disrupts corepressor binding and promotes recruitment of transcriptional co-activators, leading to activation of the target gene, with an increase in messenger RNA (mRNA) and protein expression. There are temporal patterns of recruitment of TR and cofactors to endogenous TREs of TH-regulated genes (23,24). Recent data with the glucocorticoid receptor indicates that nuclear receptor interaction with its response element may be only seconds in duration (25). Additionally, TH can negatively regulate some target genes through interaction with a negative TRE (nTRE, an element that confers repression of gene expression by TH) (26). This chapter will focus on the major aspects of TH action, as well as describe some of its nongenomic effects.
Thyroid Hormone Metabolism and Nuclear Binding of Thyroid Hormones
Both T4 and T3 are synthesized by the thyroid gland, although T4 is the major secretory product. T4 can be considered a prohormone for the active hormone, T3. The major pathway for the production of circulating T3 is via 5′ deiodination of the outer ring of T4 by deiodinases (20,27). Type I deiodinase (D1) is expressed in the liver and kidney. Type 2 deiodinase (D2) has high affinity for T4 (Kd in the nanomolar range) and is found primarily in the pituitary gland, brain, brown fat, and skeletal muscle, where conversion of T4 to T3 modulates the intracellular concentration of T3. D1 expression is directly stimulated by T3 (28). D2 expression is reduced when T4 concentrations are increased and it increases in activity when serum T4 concentrations are low. This process is controlled through rapid and local deactivation and activation by a ubiquitination/deubiquitination process (28,29). In the presence of high concentrations of T4, ubiquitin is attached to the D2 protein and it is degraded, leading to low D2 activity. In the presence of low concentrations of T4, ubiquitin
is removed through specific deubiquitinases and the D2 protein is increased, leading to high D2 activity. Thus, tissues that contain D2 can modulate T3 concentration at the local level based on the circulating concentration of T4 (by intracellular conversion to T3) in contrast to those organs that can only respond to T3. Type 3 5-deiodinase (D3) is expressed in placenta, brain, and skin, and along with D1, converts T4 to reverse triiodothyronine (rT3) by removal of an outer ring iodine, leading to the inactivation of TH. These deiodinases have all been cloned and shown to be selenoproteins (20). Additionally, rT3 and T3 can be further deiodinated in the liver and are sulfo- and glucuronide-conjugated before being excreted in the bile. Enterohepatic circulation of TH occurs as intestinal flora deconjugates some of these TH metabolites and promotes their reuptake.
is removed through specific deubiquitinases and the D2 protein is increased, leading to high D2 activity. Thus, tissues that contain D2 can modulate T3 concentration at the local level based on the circulating concentration of T4 (by intracellular conversion to T3) in contrast to those organs that can only respond to T3. Type 3 5-deiodinase (D3) is expressed in placenta, brain, and skin, and along with D1, converts T4 to reverse triiodothyronine (rT3) by removal of an outer ring iodine, leading to the inactivation of TH. These deiodinases have all been cloned and shown to be selenoproteins (20). Additionally, rT3 and T3 can be further deiodinated in the liver and are sulfo- and glucuronide-conjugated before being excreted in the bile. Enterohepatic circulation of TH occurs as intestinal flora deconjugates some of these TH metabolites and promotes their reuptake.
T3 binds to its receptors with approximately 10- to 15-fold higher affinity than T4. The dissociation constants for liver nuclear receptors measured in vitro are 2 × 10-9 M for T4 and 2 × 10-10 M for T3. Nuclear receptors are approximately 75% saturated with TH in the brain and pituitary, and 50% saturated with TH in the liver and kidney (1). Of note, TR occupancy varies among different tissues, and thus provides a mechanism for fine adjustment of circulating TH levels to nuclear receptor concentration. Type 1 steroid receptors, such as estrogen, progesterone, glucocorticoid, and mineralocorticoid, bind to heat shock protein and are found predominantly in the cytoplasm, until bound by ligand, which disrupts binding to heat shock protein and promotes movement into the nucleus. TRs, and other Type 2 receptors, such as retinoic acid and vitamin D receptors (VDRs), are mostly nuclear, both in the absence and presence of ligand (1). In fact, TRs are tightly associated with chromatin, consistent with their proposed role as DNA-binding proteins that regulate gene expression (12,30,31).
Thyroid Hormone Receptors
TRs are members of the nuclear hormone receptor (NR) superfamily that include the steroid, vitamin D, retinoic acid, peroxisome proliferators, and “orphan” (unknown ligand and/or DNA target) receptors (21). TRs act as ligand-regulatable transcription factors that bind both ligand and DNA-enhancer elements or TREs located in the 5′-flanking region and introns of target genes. TRs have a central DNA-binding domain (DBD) that contains two “zinc-finger” motifs that intercalate with the major and minor grooves of the TRE nucleotides sequences, and a carboxy-terminal ligand-binding domain (LBD) (Fig. 8.2). The hinge region between these two domains is a stretch of multiple lysines that are important for nuclear localization of the receptor. X-ray crystallographic studies of the liganded rat TRα-1 and human TRβ LBDs demonstrate that TH is buried in a hydrophobic “pocket” lined by discontinuous stretches of amino acids with additional hydrophobic interfaces likely contributing to RXR heterodimerization (11,32). The LBD
is composed of 12 amphipathic helices, with specific helices providing the contact surfaces for protein–protein interactions with co-activators and corepressors (helices 3, 5, 6, 12 and 3, 4, 5, 6, respectively) (33). Ligand binding induces major conformational changes in the TR LBD, particularly in helix 12, which affect TR interaction with co-activators and corepressors, respectively.
is composed of 12 amphipathic helices, with specific helices providing the contact surfaces for protein–protein interactions with co-activators and corepressors (helices 3, 5, 6, 12 and 3, 4, 5, 6, respectively) (33). Ligand binding induces major conformational changes in the TR LBD, particularly in helix 12, which affect TR interaction with co-activators and corepressors, respectively.
There are two genes encoding TR isoforms (Thra and Thrb) located on human chromosomes 17 and 3, respectively (6,12). These genes generate several major TR isoforms, TRβ-l,α-l,α-2, which bind TH with similar affinity and mediate TH-regulated transcription (Fig. 8.3). The TR-α isoforms, which range from 400 to slightly over 500 amino acids in length among mammalian species, contain highly conserved DBDs and LBDs.
The Thra gene encodes two different proteins, TRα-1 and c-erbAa-2, which are generated by alternative-splicing of TRα mRNA. In the rat and human, these proteins are identical in amino acid residues 1 through 370; however, their respective sequences diverge markedly thereafter (Fig. 8.3). Consequently, c-erbAα-2 cannot bind T3, as it contains a 122-amino-acid carboxy-terminus that replaces a region in TRα-1 LBD required for TH binding. Additionally, c-erbAa-2 binds TREs weakly but cannot transactivate TH-responsive genes. On certain target genes, c-erbAα-2 may act as an inhibitor of TH action, possibly by competing for binding to TREs. A second TRα variant, c-erbAα2V, in which the first 39 amino acids of the divergent sequence are missing, has been identified; however, its function is currently unknown. Yet another surprising aspect of the TRα gene is the use of the opposite strand to generate another gene product, rev-erbA. The mRNA of rev-erbA contains a 269-nucleotide stretch, which is complementary to the c-erbAα-2 mRNA due to its transcription from the DNA strand opposite of that used to generate TRα-1 and c-erbAα-2. This protein also is a member of the NR superfamily. It is primarily expressed in fat and muscle, can bind to TREs and retinoic acid response elements and can repress transcription of target genes containing these elements. One potential role for rev-erbA may be the regulation of TRα mRNA splicing, as increased levels of rev-erbA mRNA correspond to increased TRα-1 mRNA (relative to c-erbAα-2) (34). It also promotes adipogenesis and functions as a heme receptor (35). Rev-erbA has been demonstrated to play a major role in circadian rhythms (36). This seems to be an important signal, coordinating circadian and metabolic actions with nutrient signals.
The Thrb gene encodes two major TRβ isoforms (1). This gene contains two promoter regions, and by their alternate use, one or both of the coding mRNAs are transcribed. The resultant TRβ isoforms are designated as TRβ-1 and TRβ-2. The amino acid sequences of the DNA-binding, hinge-region, and ligand-binding domains of these two TR isoforms are identical; however, the amino-terminal regions are divergent (Fig. 8.3). Both TRβ isoforms have high homology with TRα-1 DBD and LBD. They bind TREs and TH with high affinity and specificity, and mediate TH-dependent transcription. TRβ-2 is expressed selectively in tissues, and its expression may be regulated by factors such as the pituitary-specific transcription factors, Pit-1.
Both TRα-1 and TRβ-1 mRNAs and proteins are expressed in almost all tissues. TRα-1 is highly expressed in brain, heart, skeletal muscle, and brown fat, whereas TRβ-1 mRNA has highest expression in liver and kidney (2,37). The c-erbAα-2 mRNA is most prevalent in testis and brain. In contrast, TRβ-2 mRNA and protein are expressed tissue-selectively in the anterior pituitary gland and hypothalamus, as well as in the developing brain and inner ear (38). In the chick and mouse, TRβ-2 mRNA also is expressed in the developing retina (39), shown to be stimulated by the neuronal transcription factor, Neuro D1 (40). Additionally, several short forms of TRα and TRβ generated by alternative-splicing of mRNA, or by use of internal transcriptional start sites, have been found in embryonic stem cells and fetal bone cells. These short forms may have biological significance in certain tissues, especially those that interfere with the transcriptional activity of the wild-type TR (dominant negative activity).
Regulation of the TR isoform mRNAs varies and is cell-type dependent (2). In the intact rat pituitary, T3 decreases TRβ-2 mRNA, modestly decreases TRα-1 mRNA, and slightly increases rat TRβ-1 mRNA. These net changes reduce total T3 binding by 30% in the T3-treated rat pituitary. With the exception of TRβ-1 in the brain, where c-erbAα-2 levels are unaffected, T3 slightly decreases TRα-1 and c-erbAα-2 mRNA in almost all tissues. T3 has minimal effect on TRβ-1 mRNA expression in nonpituitary tissues. Additionally, TRH from the hypothalamus decreases TRβ-2 mRNA, slightly decreases TRα-1 mRNA, and minimally affects TRβ-1 mRNA in
pituitary GH3 cells. Retinoic acid also influences TR expression in hepatocytes (41).
pituitary GH3 cells. Retinoic acid also influences TR expression in hepatocytes (41).
The amino acid sequences of TR isoforms are highly conserved among mammalian species, which suggests that TR isoforms may have specialized functions. Isoform-specific function at individual target genes has been difficult to demonstrate in in vitro systems, despite distinct phenotypes observed when TR isoforms are knocked out in mice (42). TRβ-1 may exhibit isoform-specific regulation of the TRH and myelin basic protein genes, and TRβ-2 may play an important role in the regulation of the growth hormone and TSHβ gene expression in the pituitary (5,6). Complementary DNA (cDNA) microarray studies, in TR isoform knockout (KO) mice and other models indicate that TRα and TRβ have a similar profile of transcriptional regulation of target genes (43,44). However, the potency of T3-induction by TRα and TRβ varies by gene and is also influenced by the relative isoform distribution in a given tissue (44). TR isoform-selective agonists have demonstrated specific roles for TRα and TRβ, especially in metabolic regulation (11). Future studies with TR KO mice, TR siRNAs (small interfering mRNAs targeted to silence expression of a specific mRNA, such as TR), isoform-specific ligands and antagonists may help resolve the issue of TR isoform-specificity and potentially identify isoform-specific pathways (1,45). Novel therapies for diseases with TRβ-selective agonists, such as hypercholesterolemia, obesity, and diabetes have been effective by themselves or in combination with other drug therapies (11). These agents have selective metabolic actions, and minimal side effects, such as atrial arrhythmias or osteoporosis, since the heart and bone contain mostly TRα (46).
Thyroid Hormone Response Elements
TRs bind to TREs, which are typically located in the upstream promoter regions of target genes. In positively regulated target genes, TREs generally contain two or more hexamer half-site sequence of AGGT(C/A)A arranged in tandem (47,48). TRs bind to TREs, which have considerable degeneracy in primary nucleotide sequences of half-sites, as well as the number, spacing, and orientation of their half-sites (Fig. 8.4). In particular, they can bind to TREs in which half-sites are arranged as direct repeats, inverted palindromes, and palindromes that contain optimal spacing of four, six, or zero nucleotides between half-sites, respectively. Of the approximately 20 to 30 natural positive TREs that have been characterized thus far, direct repeats occur most frequently, followed by inverted palindrome and infrequently palindromes (Table 8.1). TR heterodimerization with RXR facilitates binding to a wide repertoire of nucleotide sequences and motifs, as RXR proteins enhance TR binding to DNA and reduce the rate of receptor dissociation from DNA. The specificity and affinity for the TR/RXR heterodimer is determined by sequences within the half-site, the length of the spacer region, and the sequence context within the spacer region (22).
Table 8.1 Naturally Occurring Thyroid Hormone Response Elements | ||||||||||||||||||||||||||||||||||||||||||||||||||||||||||||||||||||||||||||||
---|---|---|---|---|---|---|---|---|---|---|---|---|---|---|---|---|---|---|---|---|---|---|---|---|---|---|---|---|---|---|---|---|---|---|---|---|---|---|---|---|---|---|---|---|---|---|---|---|---|---|---|---|---|---|---|---|---|---|---|---|---|---|---|---|---|---|---|---|---|---|---|---|---|---|---|---|---|---|
|
TRs can interact with a wide variety of other nuclear receptors and transcriptional adaptor proteins (see next section); however, the RXR proteins (α, β, γ) are its most important heterodimeric partners. RXR also heterodimerizes with the retinoic acid and vitamin D receptors, and promotes binding to their respective hormone response elements. TR/RXR binds to direct repeat TREs with half-site spacing of four nucleotides, whereas vitamin D and retinoic acid receptors bind to response elements with half-site spacing of three or five nucleotides, respectively. The proposed 3, 4, 5 rule for nuclear receptor specificity on hormone response elements underscores the role of spacing between half-sites as a critical determinant of nuclear receptor binding, although other influences of response element context are likely to be important (22). RXR binds to the 5′ sequence, and TR binds to the 3′ sequence in direct repeat TREs. The DBDs interact with the major grooves of the half-sites on the same face of DNA. The carboxy-terminal end of the TR DBD forms an α-helical structure that interacts with the spacer region in the DNA minor groove between the TRE half-sites. Although protein–protein contacts between the RXR and TR DBDs contribute to dimerization, their LBDs probably contain the most critical interaction sites. The dimerization surface of TR appears to involve residues located in helices 10 and 11.
In in vitro studies, unliganded TRs can bind as homodimers and heterodimers to TREs, whereas liganded TRs bind primarily as heterodimers (12). Thus, it is likely that TR/RXR heterodimers play a major role in T3-mediated transcription. The RXRs bind a stereoisomer of all trans-retinoic acid, 9-cis retinoic acid (41). Lastly, genome-wide studies examining
androgen and estrogen receptor binding to DNA have shown that nuclear hormone receptors also bind to non-canonical sequences that have some deviation from the established nucleotide sequence for a hormone response element, and are co-regulated by other transcription factors such as FoxA1, GATA2, and Oct1 (49,50). Although this type of analysis (Chip on ChIP; ChIP seq) has not been successfully performed yet for TRs, it is likely that there will be TR binding to non-canonical DNA sites via protein–protein interactions with other transcription factors as well as complex formation with other transcription factors that may bind to DNA segments near TR binding sites.
androgen and estrogen receptor binding to DNA have shown that nuclear hormone receptors also bind to non-canonical sequences that have some deviation from the established nucleotide sequence for a hormone response element, and are co-regulated by other transcription factors such as FoxA1, GATA2, and Oct1 (49,50). Although this type of analysis (Chip on ChIP; ChIP seq) has not been successfully performed yet for TRs, it is likely that there will be TR binding to non-canonical DNA sites via protein–protein interactions with other transcription factors as well as complex formation with other transcription factors that may bind to DNA segments near TR binding sites.
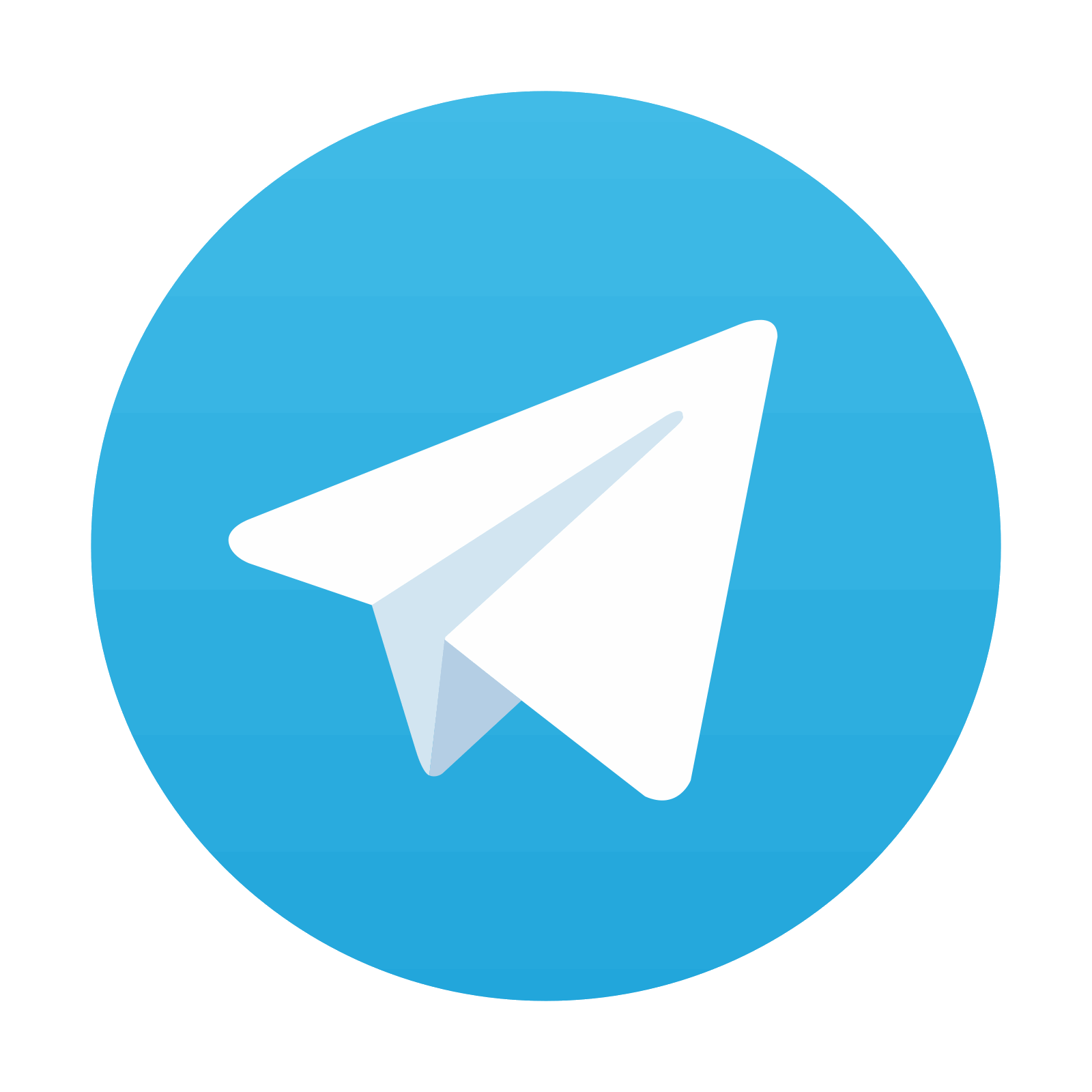
Stay updated, free articles. Join our Telegram channel
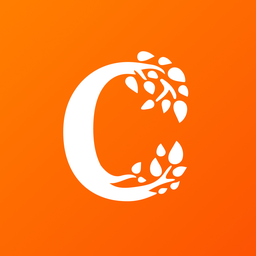
Full access? Get Clinical Tree
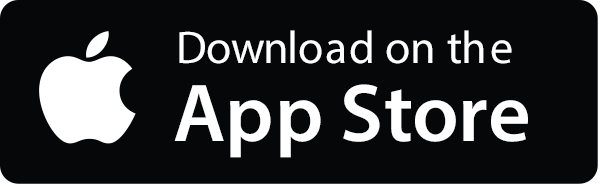
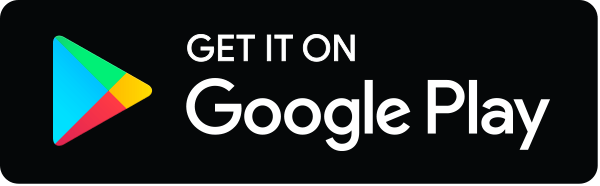