Fibrinogen Structure and Function
John W. Weisel
Carl-Erik H. Dempfle
Fibrinogen, originally factor I (FI) in the clotting cascade, is a fibrous adhesive glycoprotein, present in the plasma at a concentration of approximately 2.5 g/L, which is essential for platelet aggregation and for fibrin gel formation in hemostasis, wound healing, inflammation, angiogenesis, and other biologic processes. This chapter reviews basic and clinical aspects of fibrinogen’s structure and functions, focusing on fibrinogen’s role in hemostasis and thrombosis; other roles are covered in other chapters.
AMINO ACID SEQUENCE AND DISULFIDE BONDING
Fibrinogen is a large complex glycoprotein made up of three pairs of polypeptide chains, designated as Aα, Bβ, and γ, with molecular masses of 66.2, 54.5, and 48.4 kDa, respectively, for a total mass of about 340 kDa, including the posttranslational addition of asparagine-linked carbohydrate to the Bβ– and γ-chains.1,2,3 The unusual nomenclature for fibrinogen—(Aα, Bβ, γ)2—arises from the inclusion of “A” and “B,” representing the small peptides that are cleaved from fibrinogen by thrombin to yield fibrin monomer, which is designated as (α, β, γ)2.
In the most common forms of the polypeptide chains, there are 610, 461, and 411 amino acids in the Aα-, Bβ-, and γ-chains, respectively (FIGURE 16.1). The numbering for the amino acid sequence has been based on the mature product, but the numbering from the primary translation product is now used frequently. The amino acid sequences of the three chains are homologous, but there are also important differences that are discussed at the appropriate sections of this review. The evolutionary implications of these homologies are also be summarized.
Fibrinogen has 8, 11, and 10 cysteines in each Aα-, Bβ-, and γ-chain, respectively, for a total of 58, all of which participate in disulfide bonds.1,2 The N-termini of all six chains are held together by disulfide bonds, with five interchain bonds linking the two halves of the molecule, including one between the Aα-chains, two between the Aα– and Bβ-chains, and two between the γ-chains (FIGURE 16.1). There are two pairs of disulfide rings linking all three chains at either end of the coiled-coil regions. There are also several intrachain disulfides.
THREE-DIMENSIONAL STRUCTURE
Overall Molecular Organization
Rather than describing the historical steps in the elucidation of the structure of fibrinogen, the most important aspects of the structure itself will be outlined, starting with general features. On the other hand, some of the early work will be mentioned where it is still important for current studies and understanding functional aspects of this protein.
Fibrinogen is a fibrous protein about 45 nm in length and consists of a central nodule linked to two end nodules by rod-like α-helical coiled coils (FIGURE 16.2).3 The central nodule contains the disulfide bonds mentioned above that hold the two halves of the molecule together. There are at least two domains in the central nodule: N-terminal portions of both γ-chains meet at the center to form the γN-domain, and portions of two Aα– and Bβ-chains that meet to form what is called the funnel-shaped domain.4,5 In addition, the N-terminal Bβ-chain, which is longer than the others, has been called the Bβ-domain. Each end nodule consists of a β-module and a γ-module, which are each made up of the C-termini of β– and γ-chains, respectively, and are homologous to each other. The β-module and the γ-module each consist of three domains, the N-terminal A-domain, central B-domain, and C-terminal P-domain. The C-terminal Aα-chain, which is not homologous to the other two chains, is called the αC region and is made up of a compact C-terminal portion called the αC-domain and a flexible tether called the αC-connector that is variable in different species of fibrinogen. The two α-helical coiled coils are made up of all three polypeptide chains, and the distal portions are four stranded, with the addition of a part of the Aα-chain that runs antiparallel to the other three strands.
Visualization of Individual Molecules by Electron Microscopy
Aspects of the overall shape of fibrinogen have been deduced from a variety of physicochemical and structural methods. A diverse array of structures were proposed over many years, and it should be remembered that crystallographic structures do not capture the molecular flexibility and other potential structures that may be present in solution.
One of the first proposals for fibrinogen’s shape arose from transmission electron microscopy of rotary shadowed molecules.6,7,8,9,10 Fibrinogen appeared to have an elongated, 46 nm long trinodular shape (FIGURE 16.3A). A variety of artifactual images of negatively contrasted fibrinogen were then published, in retrospect suggesting that the protein reacts adversely to the low pH of most common heavy metal stains. Subsequent studies revealed that fibrinogen carefully prepared by this method shows the same basic shape.7,10 Electron microscopy continues to be useful for observations of binding interactions, aspects of fibrin polymerization, and visualization of parts of the molecule that are not seen in crystal structures.
Structural Insights from Limited Proteolysis of Fibrinogen
Before the crystallographic structure of fibrinogen was determined, the enzymatic degradation products of fibrin(ogen) were extensively analyzed to decipher the structure of the molecule.11 The nomenclature originally developed has persisted, with the main parts of fibrinogen that remain significant being fragments X, Y, D, and E. These proteolytic fragments are still extensively used in research, are important to understand fibrinolysis (see Chapter 20), and are used in some clinical assays.
Fibrinogen is first converted by plasmin to a clottable derivative, called fragment X, which is missing the N-terminal end of the Bβ-chains 1 to 42 and the C-terminal ends of the Aα-chains. Fragment × is then cleaved asymmetrically into fragment D and fragment Y, the latter in turn cleaved into another fragment D and fragment E. Fragment D is made up of Bβ-modules and a γ-module and approximately the distal half portion of the coiled-coil region. D-dimer (or double D) consists of two D fragments covalently cross-linked by FXIIIa. Fragment E is made up of the central domain and the proximal portion of both coiled coils. It is important to note that each of these fragments is made up of multiple domains, so the older practice of calling them domains is inappropriate, and they should be called D and E regions.
Carbohydrate
Each fibrinogen molecule contains two pairs of biantennary oligosaccharides attached via N-glycosyl bonds at Bβ364 and γ52. The amino acid motifs at these two sites are NXT and NXS, respectively, typical of N-glycosylation. The Aα-chains contain two NXS sequences but no attached carbohydrate, although extended AαE-chains do. Circulating fibrinogen is variably desialated, leading to heterogeneity, with about equal amounts of mono- and disialyated chains, but generally no asialo chains.12,13
The presence of carbohydrate on fibrinogen has important functional consequences. The most striking example comes from patients with cirrhosis and other liver disease who have hypersialated fibrinogen that forms clots with thin fibers and many branch points.14 Conversely, if all sialic acid on fibrinogen is removed with neuraminidase, fibrinogen makes clots consisting of thicker fibers with fewer branch points.15 Further, complete
removal of all carbohydrate has more extreme effects, leading to clots with extremely thick fibers and even fewer branch points.16 These results indicate that the carbohydrate on fibrinogens modulate clot structure through effects of both charge and mass on polymerization, probably particularly lateral aggregation.
removal of all carbohydrate has more extreme effects, leading to clots with extremely thick fibers and even fewer branch points.16 These results indicate that the carbohydrate on fibrinogens modulate clot structure through effects of both charge and mass on polymerization, probably particularly lateral aggregation.
Circulating fibrinogen also contains variable amounts of nonenzymatic glycation. Patients with uncontrolled diabetes have increased levels of fibrinogen glycation due to the high concentrations of sugar in their blood, but even nondiabetics have several sugar residues attached. The effects of nonenzymatic glycation have not been fully elucidated, but higher levels of glycation appear to result in clots made up of thinner fibers and more branch points.17,18,19,20,21,22,23,24
Structure of the End Regions of Fibrinogen
The homologous β– and γ-modules are each made up of three domains, the N-terminal A-domain, central B-domain, and C-terminal P-domain,25 but the two modules are oriented quite differently (FIGURE 16.4).26 The β-module is folded back about 130 degrees with respect to the γ-module, as predicted from analysis of the molecular packing in fibrinogen crystals.9
The P-domain is named because it contains a major polymerization pocket. Before structure determinations of this part of fibrinogen, short peptides containing the Gly-Pro-Arg sequence, the N-terminal end of the α-chain of fibrin, were found to bind to fibrinogen and to be effective polymerization inhibitors.27 Such peptides were incorporated into crystals of the γC-module and fragment D and were identified in the binding pocket of the P-domain.28 There are only subtle changes in the conformation of the P-domain upon peptide binding.29 The binding sites for the peptide are present on three extended loops that form a cavity with many negatively charged side chains complementary to the peptide amino acids. Residues in contact with the peptide are Gln329, Asp330, His340, and Asp364.
Peptides with the sequence Gly-His-Arg, corresponding to the N-terminus of the β-chain, also inhibit polymerization, but to a lesser extent.27 In contrast to Gly-Pro-Arg binding to the γ-module, binding of Gly-His-Arg to the β-module results in a major conformational change.29,30 Specifically, two carboxylates from BβAsp398 and BβGlu397 rotate inward to complete formation of the β-module P-domain-binding pocket.
There is one strand of β-sheet near the C-terminal end of the γ-chain that is inserted into the central B-domain. This strand can be pulled out of the γ-module without destroying its compact structure, which could be significant for FXIIIa-induced cross-linking and for the mechanical properties of fibrin.31,32
Structure of the Central Region of Fibrinogen
The central region contains portions of all six chains tightly folded into a compact structure held together by disulfide bonds.4 In addition, there is an extensive “handshake” interface with many noncovalent interactions (FIGURE 16.5). Neither the A nor B fibrinopeptides (Fps) are visible in the crystal structure nor is the larger N-terminal end of the Bβ-chain. On one face of the central region, the Aα– and Bβ-chains from the two half molecules form a funnel-shaped domain with a hydrophobic core, while on the opposite face, the N-terminal γ-chains form a separate domain. Although the two halves of the molecule are identical in amino acid sequence, a pair of adjacent disulfide bonds constrain the two γ-chains to adopt different conformations. The resulting asymmetry of the molecule may be responsible for the twisting of oligomers and protofibrils.33,34
Coiled Coils
The central and end regions of fibrinogen are joined by α-helical coiled coils that are principally responsible for fibrinogen’s elongated shape. Although the coiled coils represent only 30% of its molecular mass, fibrinogen was one of first proteins in which
this structural element was identified from wide-angle x-ray diffraction patterns.35 There are 111 or 112 residues in each of the three chains that form the coiled coils that are bounded at each end by disulfide rings. The amino acid sequences are largely consistent with those of typical coiled coils with nonpolar residues pointing inward forming a hydrophobic core shielded from solvent and charged residues exposed to solvent on the outside; there are specific interactions between the chains.36,37 Near the middle of the coiled coil, there are residues less consistent with the canonical sequence, giving rise to a kink in the molecule.38 This region is susceptible to proteolytic attack and is a major site of cleavage during fibrinolysis. The distal portion of the coiled coil has a fourth strand of α-helix formed from the C-terminal part of the Aα-chain.39
this structural element was identified from wide-angle x-ray diffraction patterns.35 There are 111 or 112 residues in each of the three chains that form the coiled coils that are bounded at each end by disulfide rings. The amino acid sequences are largely consistent with those of typical coiled coils with nonpolar residues pointing inward forming a hydrophobic core shielded from solvent and charged residues exposed to solvent on the outside; there are specific interactions between the chains.36,37 Near the middle of the coiled coil, there are residues less consistent with the canonical sequence, giving rise to a kink in the molecule.38 This region is susceptible to proteolytic attack and is a major site of cleavage during fibrinolysis. The distal portion of the coiled coil has a fourth strand of α-helix formed from the C-terminal part of the Aα-chain.39
The coiled coils provide the length needed for fibrin polymerization and for the platelet cross-linking responsible for platelet aggregation. The coiled coils are stable and relatively rigid structures whose mechanical properties are likely critical for the overall viscoelastic behavior of fibrin clots. Although force spectroscopy experiments have suggested that the coiled coils may unfold,40,41 the situation now appears to be much more complex. The γ-modules appear to unfold first, with the coiled coils acting as molecular capacitors, reversibly stretching to buffer the unfolding transitions of the γ-module.32 The coiled coils also provide a surface containing some specific motifs for binding of other proteins.
Structure of the αC Region
Each αC region is made up of a relatively compact or globular region, the C-terminal portion of which is called the αC-domain and the extended N-terminal region, called the αC-connector. Together, the two αC regions comprise about 25% of the total mass of fibrinogen. The αC-domain has a β-hairpin turn and another loose β-hairpin turn, yielding a mixed parallel/antiparallel β-sheet structure.42,43,44 The αC-connector is highly variable in different species of fibrinogen and is partially made up of 10 tandem repeats of 13 amino acids, each containing a characteristic Trp residue and rich in Gly, Ser, Thr, and Pro residues. Such sequences are found in extended poly-L-proline type II helices, which are left-handed with three residues per turn.45 It is possible that the αC-connector also has such a structure, since these helices are highly hydrated, are stabilized by hydrogen bonds with water molecules, and tend to form interdomain links or “sticky arms.”
In fibrinogen, the two αC regions interact with each other intramolecularly.46,47,48 Following cleavage of the Fps, particularly FpB, the αC regions dissociate and interact intermolecularly. The αC regions interact specifically to form long, branching polymers, closely related to structures formed in fibrin clots.46,49 In addition, these conformational changes on conversion of fibrinogen to fibrin expose binding sites for various ligands that are otherwise cryptic49,50 (Tsurupa, 2009 #2483).
Structure of the AαE-Domain
A less common variant of the Aα-chain, called AαE, has an additional 236 amino acids at its C-terminal end.51,52 Unlike the more common C-terminus of the Aα-chain, the AαE-domain does show significant homology to the β-module and the γ-module. In contrast to these structures, however, the binding cleft of the AαE-domain is essentially neutral and is not likely to bind Gly-Pro-Arg or Gly-His-Arg, suggesting that it does not participate directly in polymerization.53 It has been proposed that this domain may act as a lectin-like carbohydrate-binding receptor.53
BIOSYNTHESIS AND METABOLISM
Gene Regulation
The three fibrinogen genes, FGA, FGB, and FGG, are expressed coordinately, such that the relative proportion of mRNA for the three chains is nearly equal.54 They share common regulatory mechanisms, with cis-acting regulatory elements upstream of sites of transcription initiation.55 However, a TATA-like sequence, a CAAT-like sequence, and a USF-binding site control liver-specific expression of FGG. In contrast, liver-specific expression of FGA and FGB is controlled by other transcription factors, such as hepatic-specific nuclear receptor.
Fibrinogen is an acute-phase protein whose levels increase in response to injury and the accompanying release of cytokines.54 Upregulation of fibrinogen expression is controlled by interleukin 6 (IL6) through type II IL6-response elements in the 5′-flanking regions of all three fibrinogen genes. Fibrin(ogen) degradation products stimulate monocytes/macrophages to produce IL6 and hence upregulate fibrinogen synthesis. A glucocorticoid response element also upregulates fibrinogen expression.
There are also negative regulators of transcription, including the cytokines IL4, IL10, IL13, and transforming growth factor-β (TGF-β). In addition, TGF-β antagonizes IL6 induction of fibrinogen mRNA at late, but not early times, after IL6 treatment. The combined early and late effects of these cytokines may be responsible for the coordinate regulation of fibrinogen synthesis during inflammation.
Synthesis and Secretion
The polypeptide chains of fibrinogen are assembled in an ordered fashion in the rough endoplasmic reticulum of the liver.56 Newly synthesized human Bβ-chains are secreted and used more rapidly than the other two chains that accumulate in intracellular pools. The interchain disulfide bonds at the ends of the coiled coil, the coiled coils themselves, and some disulfides between the two halves of the molecule are important for proper assembly of the molecule.
Tissue-Specific Synthesis
Most fibrinogen is synthesized in the liver, with a steady-state rate of production of 1.7 to 5.0 g per day and a large reserve capacity of up to 20-fold.57 The half-life of circulating fibrinogen is 3 to 5 days, with the mechanism of turnover largely unknown. Coagulation and lysis accounts for only 2% to 3% of the total catabolism, and the classic catabolism pathways for plasma proteins do not apply.58 Fibrinogen, but not the γ-chain variant, is present in platelet α-granules and appears to be taken up by the granules via receptor-mediated endocytosis. Fibrinogen synthesis, albeit in lower amounts, has been detected in tissues other than the liver, suggesting that it may function in structural or adhesive capacities in the extracellular matrix.59
FIBRIN POLYMERIZATION
Fibrinopeptide Release
Fibrin polymerization is initiated by the enzymatic cleavage of the Fps from fibrinogen by thrombin, yielding fibrin monomer. Specific Arg-Gly bonds are hydrolyzed in the Aα– and Bβ-chains, in part arising from a set of hydrophobic residues in FpA that bind to a complementary apolar region on thrombin, but other distinct regions of FpA are also involved in binding and catalysis.60 FpB is released at a considerably slower rate than FpA. The ordered release of the Fps is dictated by the specificity of thrombin for its substrate61 since replacement of FpB with a FpA-like sequence results in nearly equal rates of Fps release. As polymerization proceeds, the rate of FpB release increases greatly, suggesting that either it is preferentially released from polymers62 or that conformational changes resulting from polymerization facilitate its release.63,64
Oligomer Formation
Although the Fps are small, their release has profound effects on the solubility of the molecule as a result of exposure of previously buried binding sites. The Fps mask knobs “A” that are complementary to holes “a” that are always exposed (FIGURE 16.6),1,65 although the Fps and knobs are not visible in x-ray crystallographic structures of fragment E.4 On the other hand, crystal structures of fragment D with peptides representing the N-terminal sequences of fibrin’s α– and β-chains have demonstrated peptide-binding pockets in the γC– and βC-modules,26,29,66,67 providing strong evidence that these are holes “a” and “b” respectively. It is not yet known if each peptide represents an entire knob, but it is likely that there are more extensive regions involved. Thus, two fibrin monomers thus interact with each other in a half-staggered manner, so that knob “A” fits into hole “a.”68,69 Because of the dimeric nature of the molecule, there are two “A”-“a” knob-hole interactions holding the two monomers together (FIGURE 16.6). However, it should be noted that fibrinogen can bind to fibrin monomer because fibrinogen has holes that can accept the exposed knobs on fibrin monomer. These fibrinogen-fibrin complexes are present most of the time and represent an important buffer mechanism to prevent the polymerization of small amounts of fibrin that is generated in the blood.70,71 Additional monomers add longitudinally to the fibrin dimer forming larger oligomers (FIGURE 16.3B), which lengthen into protofibrils, defined as oligomers that are long enough to aggregate (˜0.5 µm).62,72 The “A”-“a” knob-hole interactions are sufficient to make fibers and a branched clot network.73,74
FpB cleavage exposes the knobs “B,” but the functional consequences of this event are not clear. FpA cleavage alone produces clots made up of thinner fibers than those initiated by cleavage of both Fps. This led to the hypothesis that knob “A”-hole “a” interactions are responsible for the interactions within a protofibril, while knob “B”-hole “b” interactions are responsible for lateral aggregation.75 However, lateral aggregation of protofibrils and fiber formation can occur without FpB cleavage, which is not consistent with this hypothesis.73,74 Moreover, knob “B”-hole “b” interactions are potentially problematic because holes “b” are not at the ends of the molecule but 8 to 9 nm away. Although conformational changes accompanying conversion to fibrin could potentially shorten that distance, this effect is not likely to be substantial because the band pattern of fibrin indicates that the β-nodule has nearly the same spatial relationship to the γ-nodule in fibrin as in fibrinogen (FIGURE 16.3C).9 On the other hand, the N-termini of the β-chain could be highly extended and act as “crab claws” to reach the holes.76,77 Evidence that knob “B”-hole “b” interactions can actually occur in fibrin come from the dysfibrinogenemias Metz and Frankfurt XIII in which all fibrinogen molecules are homodimers from which FpB, but not FpA, can be cleaved yielding clots with thin fibers at low temperature.74,78 In addition, recombinant mutants of γ364 that have no functional holes “a” form clots slowly with thrombin but not with reptilase.79 Thus, it appears that B:b bonds can occur at least when A:a interactions are compromised, but their role under physiologic circumstances remains unclear. There is no evidence for A:b or B:a interactions in fibrin polymerization.80,81
Addition of a third molecule to a half-staggered fibrin dimer produces an end-to-end junction where the ends of two molecules abut each other. This D-D interface is an important feature of most of the known crystal and microcrystal forms.26,38,82 At this interface, γArg275 makes contact with γTyr280 and γSer300. These interactions produce a broad interface that is likely to be similar to that at the end-to-end junction between monomers in protofibrils.
Protofibril Growth by Lateral Aggregation
The existence of a structure, called the protofibril, was based on the observation that oligomers must reach a certain length before they can aggregate laterally, so protofibrils are essentially longer oligomers.68 Structurally they are two-stranded structures made up of half-staggered fibrin monomers. Protofibrils are twisted structures, with a long pitch.34,69
Protofibrils associate with each other laterally to make fibers (FIGURE 16.7). Because protofibrils do not associate until they reach a length of at least 0.5 µm,62,72 it is likely that the interactions responsible for lateral aggregation are weak and additive over the length of the protofibril. However, little is known about the nature of these interactions or their localization. Potential clues come from the analysis of interactions between D fragments in crystals (FIGURE 16.8).76 Although there is as yet no evidence that the crystallographic contacts are present in fibrin, structural proteins that self-assemble do tend to use similar
binding interactions in vitro.82,83 In this scheme, the D regions of two laterally aligned protofibrils interact, such that residues γ350-360 and γ370-380 are shielded from water. In addition, the αC regions of fibrin interact with each other intermolecularly and enhance lateral aggregation (FIGURE 16.7).47,48,49,84 Although they are not required for lateral aggregation, clots made from fibrinogen missing the αC regions are made up of thinner fibers.
binding interactions in vitro.82,83 In this scheme, the D regions of two laterally aligned protofibrils interact, such that residues γ350-360 and γ370-380 are shielded from water. In addition, the αC regions of fibrin interact with each other intermolecularly and enhance lateral aggregation (FIGURE 16.7).47,48,49,84 Although they are not required for lateral aggregation, clots made from fibrinogen missing the αC regions are made up of thinner fibers.
Although such binding interactions may be present in fibrin, packing in fibrin is not crystalline, but paracrystalline.85,86,87 Consequently, fibrin molecules are in good register in the longitudinal direction but are less well ordered across the fibers.88 In addition, the twist present in protofibrils leads them to twist around each other to make twisted fibers (FIGURE 16.3B).34 Because the periodicity of 22.5 nm is maintained as new protofibrils are added (FIGURE 16.3C), they must be stretched as their path length increases as fibers grow thicker. It has been proposed that fibers stop growing laterally when the energy necessary to stretch an added protofibril exceeds the energy of bonding.
Fibrin Branching
Fiber branching is necessary to produce a three-dimensional network. Although little is known about mechanisms of branching, one clue comes from the fact that fiber ends are seldom observed in an undamaged clot. Thus, it is likely that protofibrils do not add to a growing fiber with their ends in register but with random longitudinal spacing and that protofibrils of different lengths and smaller oligomers add to the growing fiber.
Nearly all branch points in fibrin clots are trifunctional, in other words, a junction of three fibers.89 Observations of branching by either scanning electron microscopy or transmission electron microscopy of negatively contrasted specimens show that the diameters of all three fibers at a branch point are often, but not invariably, similar (FIGURE 16.9). There
is a general correspondence between numbers of branch points and fiber diameter; as the number of branch points increase, the fiber diameters decrease.89 Together, these observations suggest that branching and lateral aggregation are opposite sides of the same coin, that is, conditions that favor lateral aggregation tend to produce clots with thick fibers and few branch points, while conditions that inhibit lateral aggregation tend to yield clots made up of thin fibers with many branch points.
is a general correspondence between numbers of branch points and fiber diameter; as the number of branch points increase, the fiber diameters decrease.89 Together, these observations suggest that branching and lateral aggregation are opposite sides of the same coin, that is, conditions that favor lateral aggregation tend to produce clots with thick fibers and few branch points, while conditions that inhibit lateral aggregation tend to yield clots made up of thin fibers with many branch points.
At the molecular level, branches could result from two protofibrils that associate over part of their lengths and then diverge from each other or from the addition of two fibrin monomers that diverge from each other at the end of an individual protofibril.90
Fibrin Structure and the Gel Point or Clotting Time
Once the branching fibers form a space-filling, three-dimensional network, a clot or gel exists (FIGURES 16.10 and 16.11). The gel point or clotting time has been used as an indication of altered or pathologic coagulation. The gel point occurs relatively early in polymerization, perhaps when only 10% of the fibrinogen has been incorporated into the gel. Under standard conditions, however, it is a sensitive measure of the overall kinetics of the clotting cascade, and hence an indicator of abnormalities. Although there are correlations between gel time and final clot structure,91 the complete and final network is generally not established at the gel point, with new fibers and branch points still being established after the gel point has been reached.92
The structure of a clot can be characterized by fiber diameter, density, number and nature of the branch points, distances between branch points, and the size of the pores (FIGURE 16.10). Although diffusion of proteins is rarely affected by the fiber network, perfusion of fluid through the gel is a sensitive measure of pore size and hence clot structure.93
Role of Calcium in Fibrinogen Structure and Function
Calcium binding to fibrinogen is important for its structural stability and function. X-ray crystallography has revealed one calcium ion in the γ-module and two in the β-module.25,26 Specific residues in the calcium-binding loop, γ311-336 (as
well as corresponding residues in the βC-module), together with two strongly bound water molecules, form a high-affinity calcium-binding site that differs from a classic EF hand. Since the dissociation constant for calcium ion binding to these sites is much lower than the free calcium ion concentration in plasma, the sites should normally be fully occupied in fibrinogen. Physiologic concentrations of calcium are important for maintaining the stability of fibrin(ogen), protecting against plasmin digestion and denaturation by heat and high temperature, as well as the extent of reduction of disulfide bonds.94,95,96
well as corresponding residues in the βC-module), together with two strongly bound water molecules, form a high-affinity calcium-binding site that differs from a classic EF hand. Since the dissociation constant for calcium ion binding to these sites is much lower than the free calcium ion concentration in plasma, the sites should normally be fully occupied in fibrinogen. Physiologic concentrations of calcium are important for maintaining the stability of fibrin(ogen), protecting against plasmin digestion and denaturation by heat and high temperature, as well as the extent of reduction of disulfide bonds.94,95,96
There are also lower affinity calcium-binding sites that may have regulatory roles, some of which may bind to sialic acid.15 Several lower affinity calcium-binding sites are associated with interactions of the βC-module with the coiled coil.29,67,97 A movable flap made up of β397Glu and β398Asp is pinned back to the coiled coil with a calcium bridge until the GHR peptide occupies the βC-module-binding pocket.
There is no effect of calcium on thrombin-catalyzed Fp release, but it does have effects on subsequent polymerization step(s). Key calcium-binding residues in the γC-module appear to be necessary for protofibril formation.98 Calcium increases the rate and extent of lateral aggregation, such that thicker fibers are formed at higher calcium ion concentrations. There is a calcium-dependent conformational change in fibrin that is correlated with the release of FpB,”99 and simultaneously, two calcium-binding sites shift from low to high affinity,100 which may be related to the structural changes observed by x-ray crystallography.29,67
The peptide representing the knobs “B,” GHRP, binds 10 times more strongly to hole “b” in the presence of 2 mM calcium than in its absence. Correspondingly, the βC-modules have lowaffinity binding sites,66 and mutation of one of them resulted in increased lateral aggregation, consistent with a role for knob “B”-hole “b” interaction involvement in lateral aggregation.97
Factor XIIIa-Catalyzed Cross-Linking of Fibrin
FXIIIa facilitates clot stability by catalyzing the formation of covalent by Nε-(γ glutamyl) lysine bonds between fibrin molecules. FXIIIa also cross-links other proteins, including α2-antiplasmin, plasminogen activator inhibitor-2 (PAI-2), and fibronectin, to fibrin. FXIII is discussed in detail in Chapter 17, but several aspects of FXIII relevant to fibrin properties are discussed here.
γ-Chain Cross-Linking
Fibrinogen γ-chains are cross-linked more rapidly than α-chains and γ-chains are cross-linked more rapidly in fibrin than in fibrinogen. The latter results from the physical proximity of the acceptor and donor sites in fibrin.101 Residues γGln398/399 and γLys406, located near the C-terminal end of the γ-chain, are cross-linked.102 The three-dimensional structure of the crosslinks is yet unknown, since the crystal structure of cross-linked human D-dimer does not show the region where the cross-links are present, likely because of the flexibility of the end of the γ-chains. However, half of one cross-link is visible in the crystal structure of lamprey D-dimer.103 There is still controversy about whether the γ-chains of fibrin are cross-linked longitudinally or transversely; evidence for both viewpoints is summarized elsewhere.104,105,106,107 The slow formation of γ-chain multimers confers greater resistance to fibrinolysis.
α-Chain Cross-Linking
Cross-linking via α-chains occurs more slowly, with multiple bonds per molecule. The α-chains contain potential glutamine acceptor sites at residues 221, 237, 328, and 366 and lysine donor sites at residues 508, 539, 556, 580, and 601.102,108 The noncovalent association of αC regions brings acceptor and donor sites in proximity, facilitating the formation of the isopeptide bonds catalyzed by FXIIIa. The result is a network of αC regions, called αC polymers.
MECHANICAL PROPERTIES OF FIBRIN
The unique mechanical properties of fibrin are essential for its functions in hemostasis and wound healing since fibrin must be rigid enough to stop bleeding and yet open for perfusion of liquid and penetration of cells. The mechanical properties of fibrin in a thrombus will determine how it responds to flowing blood.109 Reversible elastic deformation, irreversible plastic deformation, and embolization are all responses that can have serious clinical outcomes. Clots of low stiffness may lead to bleeding, while stiff clots are associated with premature coronary thrombosis.110 Moreover, the effects of nearly all therapeutic regimes involving clots or thrombi, including thrombolysis, percutaneous coronary interventions, and surgery, are dependent on these mechanical properties.
Fibrin forms a branched three-dimensional network that is a viscoelastic polymer.109 Finite stiffness is first established when a continuous network is established at the gel point and the stiffness increases over time as polymerization proceeds. Stiffness is also increased by the formation of covalent bonds introduced by FXIIIa, making the gel more stable mechanically and more resistant to proteolytic digestion. The viscoelastic properties determine how the clot responds to the forces to which it is subjected, such as flowing blood or contraction of muscle. A stiff clot will deform less than a softer one with the same applied stress. A clot with a larger inelastic or viscous component will incur more irreversible deformation than one with a smaller inelastic component.
Clots are highly extensible, meaning that they can be greatly stretched before breaking.111 At least part of the reason for this behavior is the great extensibility of individual fibers making up the clot,112,113 but the structural rearrangements that occur with stretching also play a role.
For small strains over short times, many clots are highly elastic with little inelastic component,114 meaning that stiffness remains the same under a variety of conditions during the application of force, which can be quite different depending on whether the clot is arterial or venous. The origins of the inelastic component of fibrin are unknown, but it has been suggested that protofibrils may slide past each other, or knob-hole bonds may be broken and reformed, or certain domains of the fibrin may unfold.109 In contrast to most nonbiologic polymers, fibrin exhibits strain stiffening or hardening, which means that the stiffness increases with increasing strain.115,116 This nonlinear elasticity is probably important to prevent damage to clot structures at high strains.
The viscoelastic properties of clots can only be understood by integration of their material properties over all levels of structure, including macroscopic, network, fiber, and molecular. The imposition of stress on a clot causes distinctive changes at each level of structure. Initially, fibers align
along the direction of strain, bundling fibers into higher order structures. Then, fibers become aligned as a result of the strain, stretching, which causes unfolding of some domains, exposing buried hydrophobic residues, which aggregate and expel water.111
along the direction of strain, bundling fibers into higher order structures. Then, fibers become aligned as a result of the strain, stretching, which causes unfolding of some domains, exposing buried hydrophobic residues, which aggregate and expel water.111
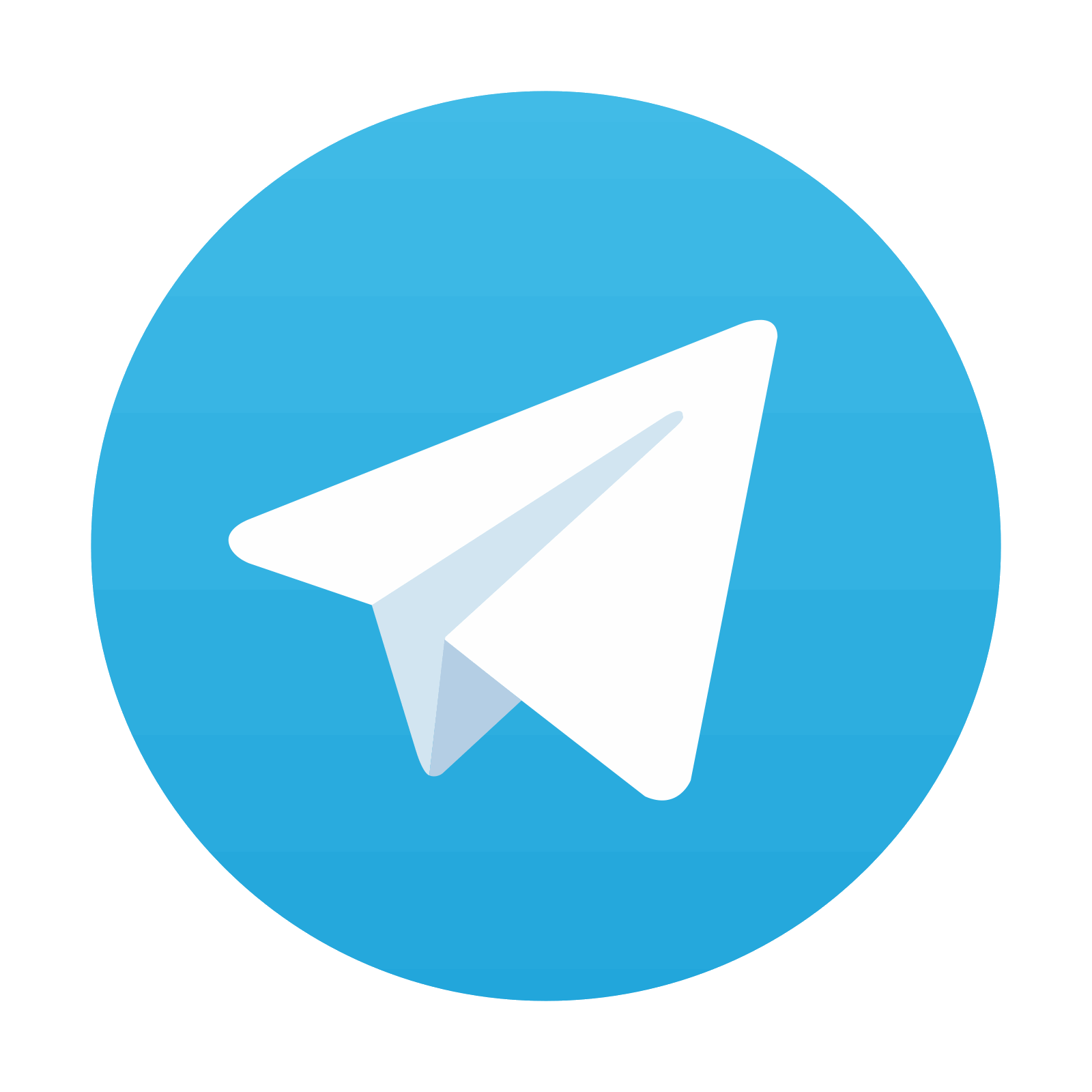
Stay updated, free articles. Join our Telegram channel
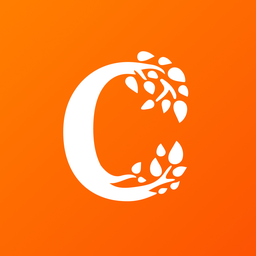
Full access? Get Clinical Tree
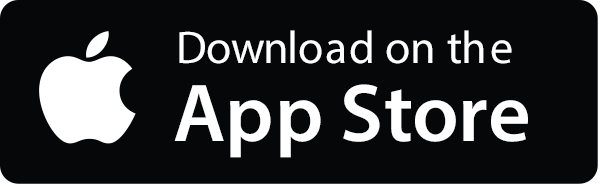
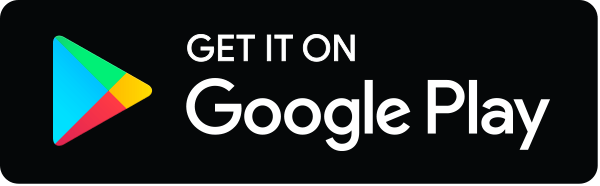