Fat Metabolism in Diabetes
Ananda Basu
Michael D. Jensen
The pathophysiology of diabetes mellitus involves impairments in insulin secretion and insulin action. Whereas type 1 diabetes results from extreme impairment or absence of insulin secretion, an important causal factor in type 2 diabetes is resistance to insulin action, with impairment in insulin secretion progressing as the disease continues. In both type 1 and type 2 diabetes, major changes in fatty acid metabolism are concomitant with alterations in carbohydrate metabolism. As will be discussed, perturbations in the release of free fatty acids (FFAs) by adipose tissue result in an increase in plasma concentrations of FFAs. Abnormally high concentrations of FFAs can lead to defects in insulin action. In addition, recent evidence suggests high plasma concentrations of FFAs can affect the secretory capacity of pancreatic β-cells.
OVERVIEW OF FATTY ACID METABOLISM
In the postabsorptive state, muscle, heart, liver, and renal cortex use fatty acids as their primary fuel (1). In humans, in contrast to rodents, there is very little de novo synthesis of fatty acids (2), either in adipose tissue or in the liver. Hence, the proximate source of fatty acids in humans is dietary, with the fatty acids generally stored in adipose tissue or oxidized (3).
In the postprandial absorptive state, dietary fatty acids are packaged in the intestinal mucosa as chylomicrons and transported by the lymphatic system to the circulation. These triglyceride-rich particles are cleared rapidly under normal circumstances, with the majority of the lipid content stored in adipose tissue (3). The cholesterol-rich chylomicron remnant particles are cleared by the liver by binding to specific receptors (low-density lipoprotein receptor-related protein) on hepatocytes.
Plasma FFAs derive almost exclusively from lipolysis of adipose tissue triglycerides by hormone-sensitive lipase (HSL), which is so named because of its responsiveness to insulin and catecholamines. The one apparent exception is following the ingestion of a mixed meal, when some plasma FFAs arise from the rapid hydrolysis of plasma chylomicron triglycerides by lipoprotein lipase (LPL), an enzyme that acts primarily to allow tissue uptake of circulating triglycerides (4). In the postabsorptive state, lipolysis by adipose tissue HSL is likely the overwhelming, if not the only, source of FFAs (5).
The hydrolysis of circulating lipoprotein triglyceride is mediated by LPL at the capillary lumen (6). LPL activity is greatest in adipose tissue, where its regulation is reciprocal to that of HSL. During the postabsorptive state, HSL is activated, whereas LPL is inhibited; in the absorptive state, HSL is inhibited and LPL is activated. The regulation of LPL activity also is tissue specific, with LPL activity in adipose tissue stimulated by insulin, whereas LPL activity in skeletal muscle is unaffected by insulin (7,8).
After their release from adipose tissue, circulating FFAs are bound to albumin, which is a very effective solubilizer. FFAs are rapidly removed from the circulation by various tissues, with a half-life of less than 4 minutes (5), and they supply 25% to 50% of energy requirements postabsorptively in adults. FFAs not utilized for oxidative needs are reesterified, probably to a large extent in the liver, and exported as very-low-density lipoprotein (VLDL) triglyceride. The VLDL triglyceride is then re-stored primarily in adipose tissue. FFAs can thus be released in excess of energy needs without disastrous consequences because the cycle of FFA to VLDL triglyceride back to adipose tissue triglyceride is relatively efficient under most circumstances.
Plasma concentrations of FFAs reflect the balance between their release and uptake, but this relationship is not linear over the physiologic range (9,10). At low flux rates, a disproportionate
decrease in plasma concentrations of FFAs occurs, whereas at very high flux rates, plasma levels of FFAs may increase to a greater degree than would be predicted if the relationship between flux and concentrations were linear. Basal FFA flux typically exceeds the fatty acid oxidation rate (measured by indirect calorimetry) by 50% to 100%; the excess FFAs are thought to be reesterified into triglycerides in the liver (11) and muscle (12,13,14,15). FFA clearance increases dramatically with exercise, such that at the onset of exercise plasma concentrations of FFAs may decrease even while rates of FFA release are increasing. Thus, although FFA concentrations are a reasonable indicator of FFA release, it is not possible to use concentration values to make quantitative estimates of release rates, and in some circumstances FFA concentrations can be misleading with respect to changes in lipolysis.
decrease in plasma concentrations of FFAs occurs, whereas at very high flux rates, plasma levels of FFAs may increase to a greater degree than would be predicted if the relationship between flux and concentrations were linear. Basal FFA flux typically exceeds the fatty acid oxidation rate (measured by indirect calorimetry) by 50% to 100%; the excess FFAs are thought to be reesterified into triglycerides in the liver (11) and muscle (12,13,14,15). FFA clearance increases dramatically with exercise, such that at the onset of exercise plasma concentrations of FFAs may decrease even while rates of FFA release are increasing. Thus, although FFA concentrations are a reasonable indicator of FFA release, it is not possible to use concentration values to make quantitative estimates of release rates, and in some circumstances FFA concentrations can be misleading with respect to changes in lipolysis.
Many hormones regulate lipolysis in adipose tissue, thereby influencing the rate of FFA release. Given the profound disturbance of fat metabolism in diabetes mellitus and the fact that insulin administration to a large extent reverses the abnormalities, it is important to understand the role of insulin in the regulation of adipose tissue metabolism. Insulin is the major regulator of adipose tissue lipolysis through its inhibition of the activity of HSL, thereby reducing the release of FFAs and glycerol. Lipolysis is normally exquisitely sensitive to suppression by insulin, with a half-maximal effect occurring at concentrations of approximately 12 pmol/L. To put this in perspective, fasting concentrations of insulin typically may vary from approximately 6 to 60 pmol/L (16). Adipose tissue is much more sensitive than many other tissues to insulin in this regard. Lipolysis is more sensitive than glucose uptake and metabolism to changes in insulin concentrations (11,16). In addition to insulin, other important endogenous inhibitors of lipolysis include ketone bodies (which also stimulate insulin secretion), adenosine, and FFAs themselves (17,18,19,20).
Several hormones accelerate the release of FFAs from adipose tissue and therefore raise plasma concentrations of FFAs. Catecholamines (epinephrine and norepinephrine) are the most important stimulators of lipolysis, although growth hormone (GH) and, to a lesser degree, cortisol, also increase the release of FFAs. Catecholamines stimulate the activity of adenyl cyclase through their β-adrenergic effect, hence increasing the tissue availability of cyclic adenosine monophosphate (cAMP), which in turn stimulates the activity of HSL (2). Catecholamines reach adipose tissue through the circulation or via adrenergic nerve terminals, and the relative importance of their local neural versus systemic origin has not been fully established.
In contrast, glucocorticoids promote lipolysis via synthesis of new HSL protein through a cAMP-independent pathway (2). Cortisol may be an important promoter of lipolysis only in conditions of relative insulin deficiency and marked cortisol elevations, as in uncontrolled diabetes or stress states (21,22,23,24). This conclusion is based on experimental observations that cortisol does not stimulate lipolysis during cortisol infusion unless compensatory hyperinsulinemia is prevented by a pancreatic somatostatin clamp (21). There is evidence that the effect of GH on lipolysis depends on the synthesis of proteins involved in the formation of cAMP (2,22,25,26). However, the creation of acute GH deficiency results in a lower availability of FFAs within several hours (27), and pulsatile delivery of GH results in enhanced lipolysis within 60 to 90 minutes. These observations suggest that GH may have some direct stimulatory effects on lipolysis in vivo. Other weaker stimulators of lipolysis include thyroxine, tumor necrosis factor-α (TNF-α), and prostaglandin I2(28,29,30). Insulin antagonizes the effects of these lipolytic hormones. The antilipolytic effects of insulin and drugs such as nicotinic acid are accounted for by inhibition of adenyl cyclase activity (2).
ASSESSMENT OF FATTY ACID METABOLISM
Both in vitro and in vivo approaches have been used to study fatty acid metabolism. The in vitro approach permits the investigator to control a maximum number of variables during studies of lipolytic regulation. However, in vivo studies allow a more physiologic assessment and an understanding of the metabolic processes in the context of the whole organism.
in vivo methods for studying systemic lipolysis (10,31,32) include isotope-dilution techniques, selective catheterization of adipose tissue venous drainage, and adipose tissue microdialysis. Regional lipolytic rates can be measured with the arteriovenous catheterization (33,34,35) or microdialysis techniques (34). The combination of arteriovenous catheterization and isotope-dilution techniques allows investigators to collect information regarding regional fatty acid kinetics (uptake and release). This approach is perhaps one of the most useful techniques for gaining an understanding of regional FFA metabolism in vivo.
Systemic appearance and disappearance of FFAs can be accurately measured with isotope-dilution techniques under both steady-state and non-steady-state conditions. An intravenous tracer infusion coupled with arterialized venous or arterial blood sampling provides data that mirror the physiology of the release by adipocytes of FFAs into the venous circulation for delivery to tissues via the arterial circulation. Under conditions of stable FFA concentrations and flux (steady state), the rate of FFA appearance equals its rate of disappearance. FFA flux is calculated using the ratio of tracer infusion rate and the specific activity or enrichment of the tracer in plasma FFAs (32).
The choice of isotopic tracers is important in the assessment of FFA kinetics. Although there are slight regional differences in the metabolism of different fatty acids (36), the kinetics of the long-chain fatty acids are sufficiently similar to consider the rate of appearance of a single fatty acid such as palmitate or oleate representative of FFA turnover in humans. Differences in the kinetics of linoleate and stearate (the other major long-chain fatty acids) compared with the kinetics of FFAs as a whole are such that tracers of these FFAs are not used to measure FFA flux (36,37). Both stable isotopic tracers (13C or 2H) and radioactive tracers (14C or 3H) are used. The main disadvantage of 14C or 3H tracers relates to radiation exposure. They are unacceptable for use in pregnant women and in children, and some institutions and governments place severe restrictions on the administration of radioisotopes to humans for research purposes. The use of stable isotopic tracers of FFAs provides the advantage of avoiding radiation exposure; however, relatively large amounts of tracer (requiring substantial amounts of albumin) are needed for standard gas chromatography and mass spectroscopy analysis. Recent advances in stable isotope approaches appear to have overcome some of these limitations (38).
Isotopic tracers of glycerol have been used to measure systemic lipolysis. The rate of appearance of glycerol is considered to reflect whole-body adipose tissue lipolysis, because adipose tissue does not contain glycerol kinase, the enzyme needed for reuse of glycerol for esterification of fatty acids (39,40,41,42,43,44). Recent studies of glycerol metabolism have suggested that this assumption is unlikely to be rigorously correct (45); thus, the rate of glycerol appearance is not a totally reliable measure of total adipose-tissue lipolysis in humans.
Regional FFA kinetics can be estimated with arteriovenous catheterization techniques combined with isotopic-tracer approaches and measurement of plasma flow (46), as well as by microdialysis techniques (47,48,49,50,51). Regional rates of FFA release can be related to regional fat content to assess the lipolytic rates of different adipose tissue beds. Regional fat mass usually is assessed by imaging studies [computed tomography (CT) or
magnetic resonance imaging (MRI)] (52) and/or by dual-energy x-ray absorptiometry (53). For the most part, these approaches allow accurate measurement of systemic and regional (leg, splanchnic, and nonsplanchnic upper body) FFA kinetics. It should be noted that FFAs released into the portal circulation by omental and mesenteric adipose tissues are partially taken up by the liver before reaching the systemic circulation (5,15). Therefore, this portion of “hidden” or “unseen” visceral lipolysis cannot be accurately determined despite the use of tracer techniques and arteriovenous catheterization approaches. Nevertheless, it is possible to use the combination of regional catheterization and isotope-dilution techniques to determine the relative contribution of the splanchnic bed, leg, and nonsplanchnic upper-body adipose tissue to systemic lipolysis (Fig. 16.1).
magnetic resonance imaging (MRI)] (52) and/or by dual-energy x-ray absorptiometry (53). For the most part, these approaches allow accurate measurement of systemic and regional (leg, splanchnic, and nonsplanchnic upper body) FFA kinetics. It should be noted that FFAs released into the portal circulation by omental and mesenteric adipose tissues are partially taken up by the liver before reaching the systemic circulation (5,15). Therefore, this portion of “hidden” or “unseen” visceral lipolysis cannot be accurately determined despite the use of tracer techniques and arteriovenous catheterization approaches. Nevertheless, it is possible to use the combination of regional catheterization and isotope-dilution techniques to determine the relative contribution of the splanchnic bed, leg, and nonsplanchnic upper-body adipose tissue to systemic lipolysis (Fig. 16.1).
Several agents have been used to manipulate FFA availability to determine the effects of FFA on tissue function. The agents most commonly used to lower plasma FFA concentrations are nicotinic acid (54) and acipimox (55,56,57). Etomoxir (57,58) (inhibits carnitine acyltransferase) has been used to inhibit FFA oxidation without lowering FFA concentrations. FFA plasma levels have been raised by intravenous infusion of a lipid emulsion to raise serum levels of triglycerides together with heparin to release LPL into the circulation (6,59), thereby generating FFA by the intravascular hydrolysis of triglycerides.
GLUCOSE-FATTY ACID INTERACTIONS
In 1963, Philip Randle and coworkers first described an interaction between glucose and fatty acid metabolism (60), which subsequently has been termed the glucose-fatty acid cycle or Randle cycle. Studying rat heart muscle and diaphragm, they observed that increasing fatty acid oxidation by these tissues impaired their oxidation of glucose. The mechanism they suggested involved increased mitochondrial ratios of [acetyl-CoA]/[CoA] and [NADH+]/[NAD] as a result of increased fatty acid oxidation. They proposed that these changes inhibit pyruvate dehydrogenase and thus limit the entry of pyruvate derived from glycolysis into the mitochondria for participation in the citric acid cycle. In addition, increased acetyl coenzyme (acetyl-CoA) derived from β-oxidation leads to accumulation of citrate that in turn inhibits phosphofructokinase, one of the key regulators of glycolysis. This results in the accumulation of intracellular glucose-6-phosphate, which further inhibits hexokinase II, the glucose-phosphorylating enzyme present in muscle (57,60). Reduced activity of hexokinase II serves to reduce glucose transport into the muscle cells. Subsequently, this metabolic cycle was extended to involve inhibition of nonoxidative glucose metabolism by inhibition of glycogen synthase by elevated FFA levels (61,62,63).
Recognition of the glucose-fatty acid cycle as observed in studies of laboratory rats brought appropriate attention to the interactions between FFAs and glucose. Much subsequent work has shown that equally important glucose-FFA interactions occur in humans but that these result from quite different regulatory mechanisms (64,65). Human studies failed to show either reduced activity of glycogen synthase and pyruvate dehydrogenase enzymes in skeletal muscle biopsies as a consequence of FFA elevation (induced by lipid emulsion- heparin infusion) (66) or an elevation in citrate or carnitine palmitoyltransferase activity reflecting increased fatty acid oxidation (62). Intramuscular concentrations of glucose-6-phosphate were not increased in response to FFA concentrations as high as 0.5 mM during hyperinsulinemia (61), suggesting reduced glucose transport/phosphorylation as an alternative primary cause of reduced glucose uptake. Concordant findings have been reported using nuclear MRI to study human skeletal muscle during a euglycemic hyperinsulinemic clamp in the presence of elevated FFA levels (64,65). Reductions in insulin-receptor substrate-1-associated phosphatidyl-inositol 3-kinase activity were found, which could lead to inhibition of glucose transport into the myocytes. In addition, it was shown that non-insulin-mediated glucose uptake was not reduced as a result of elevated FFA concentrations in nondiabetic humans, suggesting that the effect of fatty acids on glucose transport is mediated through the insulin-responsive glucose transport system (67).
In recent years, a reverse “glucose-fatty acid” cycle has been proposed (68,69), through which hyperglycemia in the presence of elevated FFA concentrations reduces fat oxidation. This could
result from a reduction in the entry of fatty acids into the mitochondria as a consequence of inhibition of carnitine acyltransferase activity (68), although increased reesterification of fatty acids in sensitive tissues represents another potential mechanism that has not been studied. In summary, although the glucose-fatty acid cycle involves a clear reciprocal relationship between glucose and fatty acid oxidation in muscle, the fundamental mechanism by which this occurs is likely different from that originally proposed on the basis of rat studies. Nevertheless, whatever the cellular mechanism of this vital metabolic cycle, elevated levels of FFAs impair the stimulation of glucose uptake by insulin in human skeletal muscle, and this represents a major site of altered glucose metabolism in individuals with type 2 diabetes.
result from a reduction in the entry of fatty acids into the mitochondria as a consequence of inhibition of carnitine acyltransferase activity (68), although increased reesterification of fatty acids in sensitive tissues represents another potential mechanism that has not been studied. In summary, although the glucose-fatty acid cycle involves a clear reciprocal relationship between glucose and fatty acid oxidation in muscle, the fundamental mechanism by which this occurs is likely different from that originally proposed on the basis of rat studies. Nevertheless, whatever the cellular mechanism of this vital metabolic cycle, elevated levels of FFAs impair the stimulation of glucose uptake by insulin in human skeletal muscle, and this represents a major site of altered glucose metabolism in individuals with type 2 diabetes.
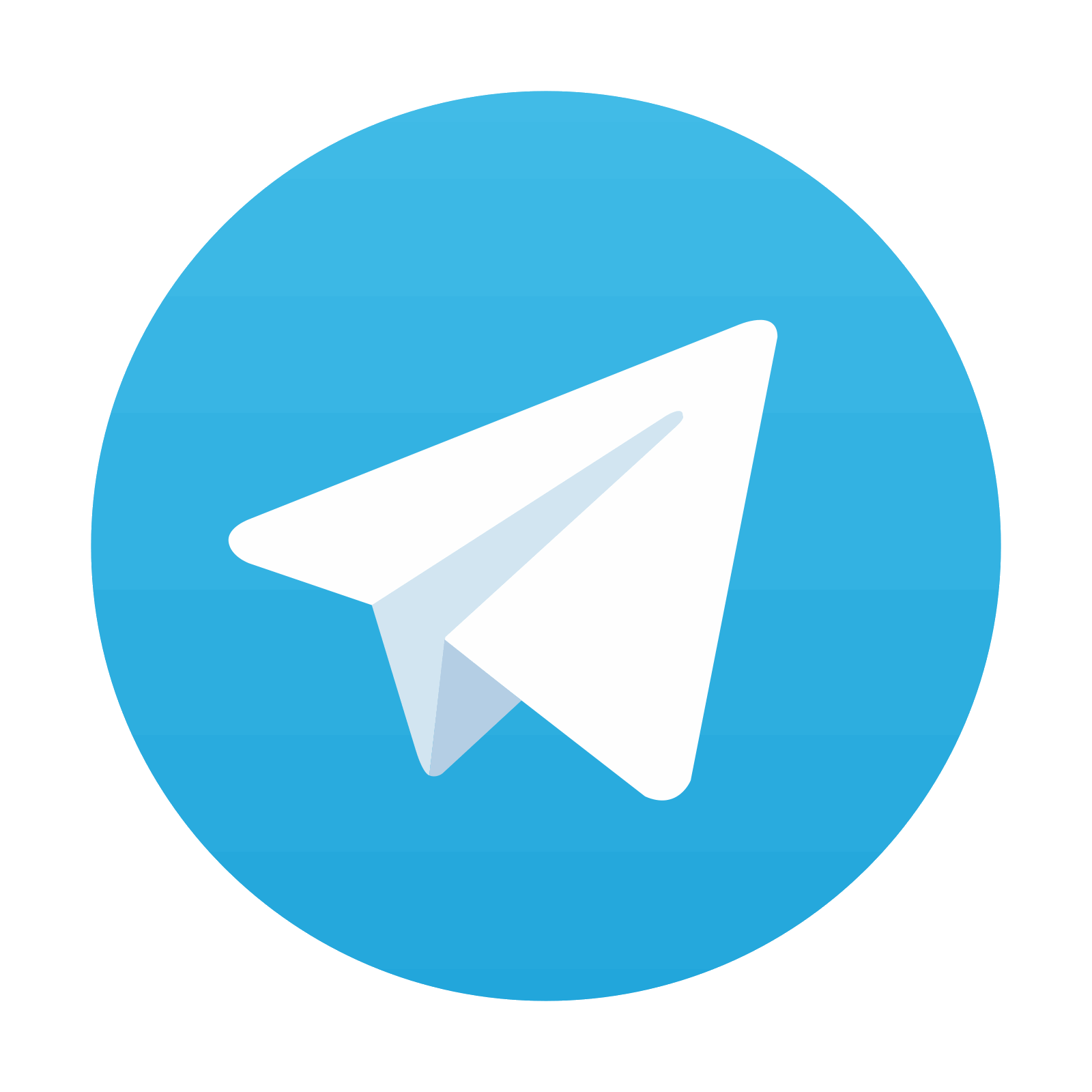
Stay updated, free articles. Join our Telegram channel
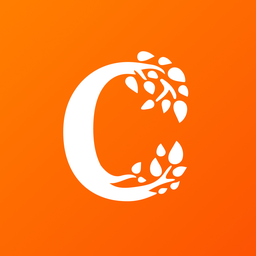
Full access? Get Clinical Tree
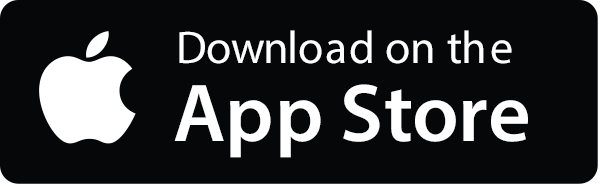
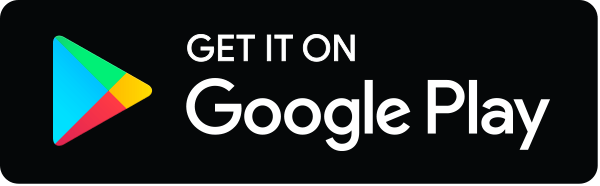