where k is a form factor accounting for the impact of the cell on the extracellular field distribution, r is the radius of the cell (assumed to be spherical), θ is the angle between the cell membrane and the field direction, τ is a membrane charging time constant, and g is a function of the three involved conductivities [16].
The permeabilization occurs when and where the potential difference crosses a critical poration value (ΔΨp), which has been estimated as being not less than 200 mV [17]. Clearly, E controls the poration, and a critical threshold has been defined also for this variable (E p), so that when E > E p, the permeabilization becomes possible [17]. According to the above equation, E p is cell size-dependent, and indeed it roughly ranges from 0.1 kV cm−1 for large cells (e.g., muscle cells) to 1−2 kV cm−1 for small cells (e.g., bacteria) [17]. While the expansion is progressing, the membrane goes through a transition characterized by abnormally high conductance and permeability. During this phase, the electric field intensity controls the geometry of the part of the cell affected, while the pulse duration controls the density of the perturbation of the cell membrane. Interrupting the administration of the electric field induces the stabilization process; when the electric field becomes subcritical (E < E p), there is a decrease of the conductance in the permeabilized part of the cell membrane, and the plasmalemma shifts from highly permeable to variously leaky [16].
During the resealing, the membrane leaks are annihilated. As stated before, sealing kinetics require seconds and are therefore generally much slower than the electric field relaxation, because pushing water out of the formed hydrophilic pore and the rearrangement of the lipid bilayer into the normal state require the overcoming of relatively high free energy barriers [18]. The picture emerging from this research is that of a pore that is initiated close to a head group defect (fluctuation) in which a cluster of polar heads points inwardly, with respect to the zero field average conformation. At the location of these defects, induced on the outer side of the membrane by the external electric field, water molecules start to penetrate the lipid bilayer, eventually forming hydrogen-bonded chains of molecules. The phenomenon is dynamically driven by the electric field, which tends to orient the dipoles of both water and head groups. The time scale on which the pore is formed is around 200 ps. At later times the pore becomes stabilized and presents an inner hydrophilic interface. If the field is removed, the pore gradually collapses and resealing is eventually observed (Fig. 28.1).
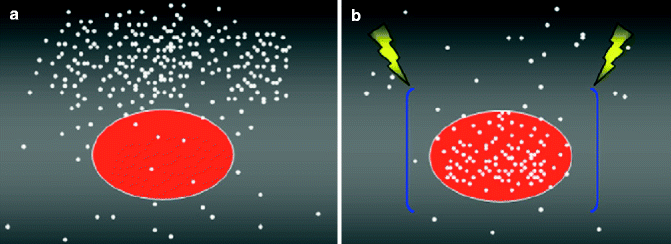
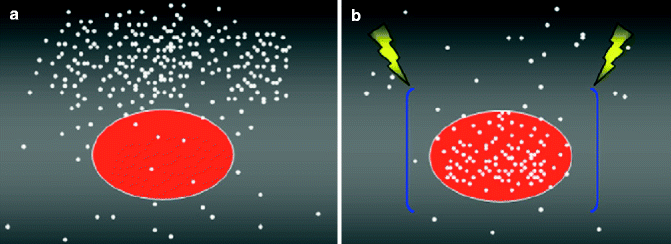
Fig. 28.1
Schematic representation of the enhanced uptake of lipophobic drug (white dots) by a tumor cell (approximated to a sphere) following the creation of an electric field (blue bars) and the administration of permeabilizing pulses (yellow sparks). (a) Drug uptake by the tumor cell before the administration of permeabilizing pulses. (b) Drug uptake by the tumor cell after administration of permeabilizing pulses (Modified from Spugnini et al. [19])
Electrochemotherapy
Electroporation is defined as permeability change in the cell membrane following exposure to short pulse of high electric field. Due to the low conductivity of the lipid bilayer, application of high external electric field generates a potential difference across the membrane. As the cross-membrane potential difference reaches a threshold value of about 200 mV, a sudden increase in membrane permeability is observed. Processes leading to this electroporation phenomenon are not fully understood on the molecular level. However, there is a general agreement that cross-membrane potential difference contributes to stabilization of transient membrane defects and to their expansion to large metastable hydrophilic pores. Both transient and stable pores can be the sites of extrinsic material entry into the cell [16, 17]. Electropermeabilization typically uses high-voltage pulses of μs to ms duration to generate the necessary electric field for opening pores in the cell membrane [20]. Optimum electroporation parameters vary depending upon the cell type and purpose. Electric field strengths of 1,200 V/cm and 100 μs pulses are applied for drug delivery. Experimental studies supported that (i) the percentage of porated cells is related to the increase of the pulse amplitude and duration and (ii) poration occurs when the pulse amplitude exceeds a threshold value. Electropermeabilization is very often performed using bursts of rectangular pulses. Their typical durations are in the range from hundreds of μs to tens of ms, while the intervals between the pulses vary from several ms to several s. The most common types of waves have been, since the pioneering years, the square, which has a defined intensity and duration [2–11] and maintains the voltage throughout the life of the pulse [2–11], and the exponentially decaying one [1]. More recently, other waves have been proposed for electroporation, in the attempt to achieve higher efficiency and greater adaptability to the in vivo setting in particular the bipolar oscillating pulses [21], such as the bipolar square pulse, which gives higher membrane permeabilization levels than unipolar pulses [21] administered in bursts (i.e., pulse trains with short interpulse intervals [21–23] for reducing the electroporation morbidity).
Many cancers are resistant to multimodal treatments, and there is a need for therapeutic innovations and discovery. The ideal treatment of cancer should effectively control local and systemically recurrent disease, be applicable to a diversity of tumor types and anatomical locations, facilitate multimodal and systemic therapies, be minimally intrusive, and improve patient well-being and life expectancy by tumor control and cure. Electrochemotherapy (ECT) has been defined as the enhanced uptake of chemotherapy agents by solid tumors, following the application of permeabilizing pulses [2]. The first and most actively studied ECT agent has been bleomycin. This drug can penetrate the cell membrane only through protein receptors due to its lipophobic nature, thus resulting in slow and quantitatively limited uptake under normal conditions [24]. The complex formed by bleomycin and its carrier is transported in the cytosol by means of endocytotic vesicles, but the mechanism of its release is still unknown. Following internalization by the cell, this drug induces single and double deoxyribonucleic acid (DNA) breaks [25] which are seen as chromosomal gaps, deletions, and DNA fragmentation that can ultimately lead to cell death [26]. Bleomycin requires oxygen and metals as cofactors to cause DNA damage [27]. It forms a complex with Cu2+, and the complex is internalized into the cell [26]. There is some evidence that the bleomycin-Cu2+ complex is a prodrug, which once inside the cell is converted to the biologically potent Fe2+-bleomycin [26]. The Fe2+-bleomycin complex binds to O2 and then to DNA, and this quaternary complex (Fe2+-bleomycin- O2-DNA) results in DNA cleavage. The binding of Fe2+-bleomycin complex to O2 happens very rapidly and is stabilized by the presence of DNA [26]. The interaction with the DNA takes place at the minor groove with clear nucleotide selectivity, e.g., preferentially at the level of the GC base pairs [26–28].
Bleomycin cuts the chromatin at the level of the linker DNA between nucleosomes [28, 29] inducing single- and double-strand DNA breaks with a ratio of six to ten single-strand to one double-strand break [26, 28, 29]. The attack to the opposite strand is not as sequence specific as the first cleavage but is cut within one nucleotide of the first attack site [28]. It has been calculated that one molecule of bleomycin can make eight to ten DNA breaks [28, 29] and that 3 × 106 bleomycin molecules can create about 5 × 106 double-strand breaks in one cell [18, 29]. Bleomycin-induced DNA fragmentation is a very rapid phenomenon: it happens within 30 s. of drug entry within the cell [28, 29]. Bleomycin may induce cell damage through other mechanisms such as release of free nucleic bases without strand cleavage, oxidative degradation of RNA, an attack on small organic molecules, and lipid peroxidation [26, 27, 29]. The high toxicity of bleomycin when it reaches the intracellular environment is impaired by its inability to freely diffuse through the cytoplasmic membrane [28, 29]. In vitro studies evidenced that less than 0.1 % of bleomycin added to culture medium becomes associated to the cell [24]. It is on this background that the cytotoxicity of bleomycin can be enhanced by 300–700-folds by electroporation [30].
In particular, one study [29] using electroporation as a mechanism to enhance the cellular internalization of bleomycin suggested two possible mechanisms of cell killing that were dependent on the number of internalized molecules of bleomycin:
1.
When only a few thousands molecules were internalized, the cells arrested in the G2–M phase and became enlarged and polynucleated before dying, thus mimicking the behavior of lethally radiated cells.
2.
When several million molecules where internalized following electroporation, the cells died showing swelling and nucleus dissolution, changes suggestive of apoptotic death.
The first use of electrochemotherapy with bleomycin in humans was published in 1991 on head and neck tumor nodules [1]. Since that time, the therapy has undergone significant advances in terms of the systems used and the cancers demonstrated to be suitable for treatment [2–5]. A number of companies, including IGEA (IGEA, Carpi, Italy) and Inovio (Inovio Biomedical Corporation, CA, USA), have developed pulse generators with approval for use in humans. In the past decade since their development, electrochemotherapy has become established as a safe and effective therapy in the treatment of a wide variety of cutaneous and subcutaneous lesions. Electrochemotherapy may be given with conventional anticancer treatments, and experiences to date suggest it may also be a significant adjunct in combination with surgery. While several cancer types appeared to be responsive to electrochemotherapy, its clinical application to date has been confined to cutaneous malignancies, often recurrent, multiple, and metastatic after multimodal therapies [6–11, 21, 22].
The treatment is associated with minimal side effects for patients, is easy to perform in a day-hospital setting, and is inexpensive. Most of the treated neoplasms have been Kaposi sarcoma, squamous and basal cell carcinoma, fibrosarcoma, and mammary carcinoma [6–11, 21, 22, 31]. Moreover cutaneous metastases of hypernephroma, ovarian cancer, mammary carcinoma, and Merkel’s tumor have been reported [32–35]. Pooling together partial and complete responses observed in the first studies, the overall response rate of the first trials with bleomycin or cisplatin was around 86 % (with a range from 62 to 100 %) [36, 37]. Figure 28.2 shows two electrochemotherapy equipments currently used in clinical oncology, and Figs. 28.3 and 28.4 show different electrodes.
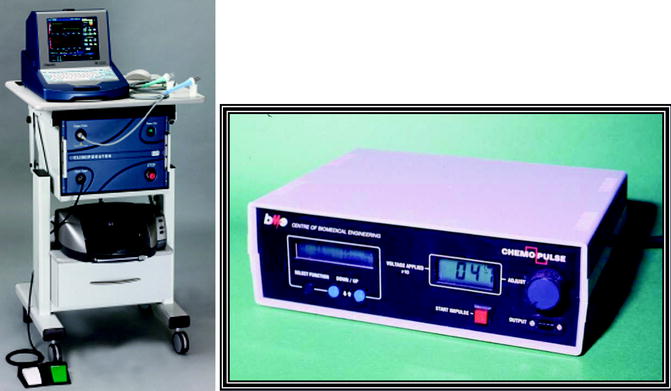
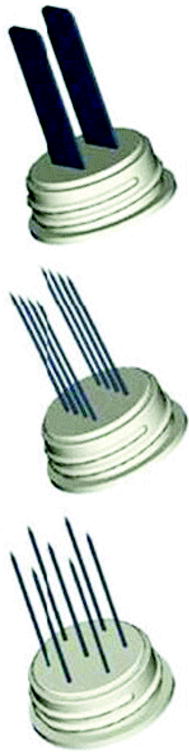
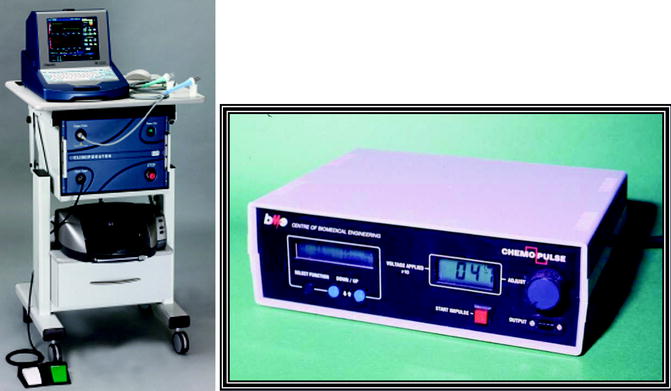
Fig. 28.2
(a) Cliniporator IGEA. (b) Chemopulse
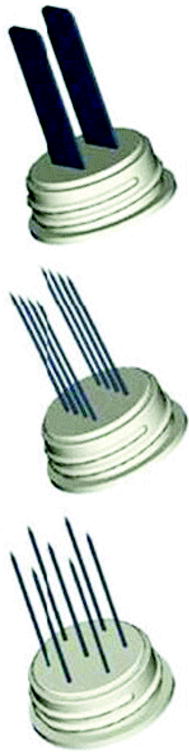
Fig. 28.3
Electrodes for Cliniporator