Development of the Endocrine Pancreas
Ondine Cleaver
Douglas A. Melton
The vertebrate pancreas is an essential organ, responsible for both digestion and glucose homeostasis. The pancreas is also the sole source of insulin production in vertebrates, and impairment leads to a major health problem, diabetes mellitus. Current research on early development of the pancreas is aimed at elucidating the generation of pancreatic cells and the genetic mechanisms underlying the anatomy and physiology of the pancreas. More specifically, the central goals of pancreas developmental studies include mapping of the spatiotemporal origins of endocrine cells and the identification of key genes that determine endocrine character. These studies also examine regulatory factors that direct the proliferation and differentiation of the pancreas from its initial commitment
in the endoderm through morphogenesis and growth during postnatal life. The possibility of islet neogenesis in vitro and transplantation as a potential treatment for diabetes makes the study of the basis for β-cell development and insulin production particularly significant (1). In addition, the prospect of using pluripotent stem cells to generate an unlimited supply of β-cells underscores our need to understand the regulation of normal endocrine cell generation in the embryo (2).
in the endoderm through morphogenesis and growth during postnatal life. The possibility of islet neogenesis in vitro and transplantation as a potential treatment for diabetes makes the study of the basis for β-cell development and insulin production particularly significant (1). In addition, the prospect of using pluripotent stem cells to generate an unlimited supply of β-cells underscores our need to understand the regulation of normal endocrine cell generation in the embryo (2).
In this chapter, we will present studies on the development of the pancreas in humans and in model organisms, including mice, rats, chickens, and frogs (3,4,5,6). Fundamental genetic mechanisms controlling germ layer and organ development are highly conserved throughout evolution, and conclusions drawn from model organisms can often be extrapolated generally. We will first review the principal landmarks of pancreatic function and anatomy. We define fundamental cell types that comprise the pancreas and examine in detail the commitment, patterning, and morphogenesis of the pancreas. We also discuss cell-lineage relationships among pancreatic cells, providing insights into the origins of endocrine cells. In addition, we introduce current research on putative pancreatic stem cells and the potential for therapeutic applications. Last, we will briefly review some of the important transcriptional regulators and growth factors demonstrated to play critical roles during islet development.
THE PANCREAS
General Anatomy
The human pancreas is a racemose, lobulated gland that weighs 60 to 170 g, is 13 to 25 cm long, and is located just caudal to the stomach and opposite the liver along the gastrointestinal tract (7,8) (Fig. 2.1). Its head (proximal portion) lies in the crook of the duodenum, and its tail (distal portion) contacts the spleen. It is also in juxtaposition to a number of large blood vessels, including the aorta, the inferior vena cava, and the superior mesenteric vein and artery and is in direct contact with the portal and splenic veins (8). The pancreas consists primarily of exocrine, endocrine, and ductal cell types that together with a blood supply coordinate to regulate nutritional equilibrium. The exocrine function of the pancreas is carried out by acinar cells, which secrete digestive enzymes and other nonenzymatic components into the duodenum. Acinar cells are located at the tips of the smaller ducts, which connect to an extensive system of larger ducts and in turn join the primary excretory duct of the pancreas. This duct, also called the canal of Wirsung, extends transversely through the body of the pancreas and connects to the duodenum at the ampulla of Vater, where it joins the common bile duct (9). The endocrine function of the pancreas is carried out by the islets of Langerhans. These are compact, spheroid clusters of cells scattered throughout the more abundant exocrine tissue. Islets consist of four different cell types that secrete hormones into the bloodstream to regulate glucose homeostasis. Islets are therefore penetrated by a network of fenestrated microvasculature and nerve fibers that help administer this regulation (10,11,12,13). It has long been observed that both endocrine and exocrine cells originate in the pancreatic endodermal epithelium and then migrate into the surrounding mesenchyme before undergoing differentiation (14,15,16,17).
The Exocrine Pancreas
The exocrine pancreas constitutes the bulk of pancreatic tissue and comprises primarily acinar cells. Acinar cells are organized into acini, which are epithelial pouches located at the tips of a branched network of ducts (Fig. 2.2A,D). These secretory cells are linked to each other by large gap junctions and are flanked by centroacinar cells at the neck of the acini. Acinar cells are pyramidal in shape and contain an extensive secretory apparatus at the apical end, including numerous zymogen granules (18). These granules contain digestive enzymes, including amylases, proteases, nucleases, and lipases, which are secreted into the duodenum. Initially, these are produced and secreted as inactive proenzymes, which are then activated by limited proteolysis once they enter the digestive tract. The ducts connect the acini and constitute a tubular epithelial network that is continuous with the gut tube. It is through the ducts that the exocrine secretions are transported into the duodenum. The duct cells produce mucins and a bicarbonate-rich fluid, which is used to neutralize the acidic product of the stomach (19). The ducts contain scattered endocrine cells, and it has been hypothesized that the ducts include a population of precursor cells that can give rise to endocrine and exocrine cells (6,17,20).
The Endocrine Pancreas
The endocrine function of the pancreas is performed by a number of cell types in the islets of Langerhans. These structures were identified by the German physician Paul Langerhans in 1869. Islets are tight aggregations or clusters of cells embedded in the surrounding exocrine tissue. There are four cell types found in pancreatic islets: α-cells, β-cells, δ-cells, and PP-cells (pancreatic peptide; also called γ-cells) (Fig. 2.2A,B,C). The β-cells are the majority of the endocrine cell population of the pancreas and secrete insulin, the insulin antagonist amylin, and other peptides (21). Insulin release is stimulated by high glucose levels, as well as by glucagon, gastric inhibitory peptide, epinephrine,
and increased levels of amino acids (see Chapter 6). β-Cells are polyhedral and packed with secretory granules (22). The α-, δ-, and PP-cells secrete glucagon, somatostatin, and pancreatic polypeptide, respectively. In most mammals, the β-cells lie in the middle of the islet and are surrounded by a thin layer of α-, δ-, and PP-cells (one to three cells thick) (23). These peripheral cells are smaller than β-cells and are also well granulated. In humans and other primates, the concentric segregation of cells within the islet is less defined, with islets sometimes taking on oval and cloverleaf patterns (24,25). The endocrine cells that produce some islet hormones are found in other regions of the gut; however, within the endoderm, insulin-expressing cells are found only in the pancreas (26).
and increased levels of amino acids (see Chapter 6). β-Cells are polyhedral and packed with secretory granules (22). The α-, δ-, and PP-cells secrete glucagon, somatostatin, and pancreatic polypeptide, respectively. In most mammals, the β-cells lie in the middle of the islet and are surrounded by a thin layer of α-, δ-, and PP-cells (one to three cells thick) (23). These peripheral cells are smaller than β-cells and are also well granulated. In humans and other primates, the concentric segregation of cells within the islet is less defined, with islets sometimes taking on oval and cloverleaf patterns (24,25). The endocrine cells that produce some islet hormones are found in other regions of the gut; however, within the endoderm, insulin-expressing cells are found only in the pancreas (26).
In the islets of the mature human pancreas, β-cells constitute approximately 70% to 80% of the islet mass; α-cells, approximately 15% to 20%; δ-cells, approximately 5%; and PP-cells, up to 1%. The pancreas of an adult human of average weight (70 kg) contains between 300,000 and 1.5 million islets (27), adult mice have approximately 100 to 200 islets (JM Wells and DA Melton, unpublished observations), and some fish have a single islet (28). Hormones produced by the islets appear sequentially during development, and the order of appearance varies slightly among different organisms. In the mouse embryonic pancreas, differentiated glucagon cells first appear at E9.5 (9.5 days postconception) followed by insulin-expressing β-cells at E10.5, and finally by somatostatin- and PP-expressing cells at E15.5 (29,30). Endocrine gene transcription, however, can be detected earlier. Somatostatin transcripts are first detectable throughout the foregut epithelium at E8; insulin and glucagon transcripts are found a day later at E9; and last, pancreatic polypeptide is detectable at E10 to 10.5 (31). Transcription of exocrine genes begins slightly later than that of all endocrine genes. Despite the synthesis of endocrine hormones during embryogenesis, it is unclear whether islets are actually functional at this time. In mouse embryos, endocrine cells respond to glucose only after fetal day 18 (32).
Overview of Pancreatic Development
In the early rodent embryo, the pancreas derives from distinct dorsal and ventral endodermal evaginations caudal to the developing stomach (33) (Fig. 2.3A,B). The dorsal bud becomes evident slightly before the ventral bud. The buds then grow and proliferate rapidly into highly branched structures. As the epithelium grows, it depends on its intimate association with a growing cap of mesodermal mesenchyme surrounding it (34,35). The dorsal and ventral anlagen then fuse during gut rotation, when the ventral bud rotates around the duodenum and joins the dorsal bud on the dorsal portion of the gut tube (Fig. 2.3C,D). The dorsal bud will form the largest portion of the pancreas, including part of the head and all of the body and tail of the pancreas, while the ventral bud will form the posterior portion of the head. Exocrine, endocrine, and ductal cell types arise from the endodermal epithelium, whereas the mesenchyme forms smooth muscle and supportive tissue (36).
Although the key landmarks of pancreatic development have been investigated quite thoroughly, many questions remain. For instance: What are the early events that result in regionalization of the endoderm along the developing gut? What determines the position of committed pancreatic endoderm between the stomach and duodenum? Are there common signals that direct the formation of pancreatic buds on both the dorsal and ventral portions of the gut tube? What is the nature of the signals from the pancreatic mesenchyme to the endoderm that are necessary for later morphogenesis and cell differentiation? Most important for diabetes research, What are the specific combinations of factors that give rise to β-cells?
ENDODERMAL ORIGINS OF ENDOCRINE CELLS
Germ Layers
During gastrulation, three fundamental germ layers are established in the embryo: the ectoderm, the mesoderm, and the endoderm (Fig. 2.4). The ectoderm gives rise to the epidermis and nervous system. The mesoderm develops into the notochord, the muscle, the heart, the kidney, the vasculature, the gut mesenchyme, and the blood. The endoderm gives rise to a variety of organs along the anterior-posterior axis, more specifically that of the gastrointestinal and respiratory tracts (Fig. 2.5). Pharyngeal endoderm gives rise to the pharynx, as well as to portions of the thymus and thyroid gland. Foregut endoderm
forms the esophagus, lungs, stomach, liver, gallbladder, and both the endocrine and exocrine cells of the pancreas. The midgut endoderm gives rise to the jejunum, the ileum of the small intestine, and the anterior colon; and the hindgut endoderm forms the caudal large intestine and rectum. In all cases, the endoderm generates primarily the epithelial lining of the organs. Splanchnic mesodermal mesenchyme surrounds the endoderm and is active in inducing and supporting its proliferation and morphogenesis.
forms the esophagus, lungs, stomach, liver, gallbladder, and both the endocrine and exocrine cells of the pancreas. The midgut endoderm gives rise to the jejunum, the ileum of the small intestine, and the anterior colon; and the hindgut endoderm forms the caudal large intestine and rectum. In all cases, the endoderm generates primarily the epithelial lining of the organs. Splanchnic mesodermal mesenchyme surrounds the endoderm and is active in inducing and supporting its proliferation and morphogenesis.
Endoderm Formation in Amphibians
The genetic basis for endoderm cell specification is presently not well understood. The signals that determine endodermal cell fate remain largely unknown, although a growing number of candidate molecules are being identified, including members of the transforming growth factor-β (TGF-β), fibroblast growth factor (FGF), and Wnt (small secreted glycoproteins) growth factor families (37,38). It is also unclear at what point embryonic cells become determined to form endoderm. This process appears to be complete by the end of gastrulation because all three germ layers are identifiable at this time.
Studies in amphibians have identified a number of genes that can direct endodermal cell fate. In the frog Xenopus laevis, the endoderm arises from the vegetal blastomeres, which invaginate through the blastopore and become internalized. The fate of these blastomeres becomes increasingly restricted to endoderm after the mid-blastula transition, as shown by transplantation experiments (39,40). These experiments have suggested that both cell-autonomous and non-cell-autonomous mechanisms are involved in the initiation of early endodermal gene expression. Several nodal-related molecules (41,42,43) and two vegetally localized maternal determinants, Vg1 and VegT, have been implicated in the regulation of both mesoderm and endoderm (44,45). In addition, several endoderm-specific factors have been identified that can direct the fate of endodermal cells in a cell-autonomous fashion. For example, the transcription factors Mixer, Mix.1, and Sox 17(α and β) and the ribonucleic acid-binding molecule XBic-C are expressed exclusively in the early endoderm and can direct ectopic endoderm formation when expressed in ectoderm cells, such as animal caps (46,47,48,49). Furthermore, when dominant-negative forms of some these molecules are expressed in the endoderm, its formation is disrupted (50). Mammalian and avian orthologues of these endoderm genes have not yet been identified.
Endoderm Formation in Amniotes
In amniotes, the primitive streak is directly involved in endoderm cell fate specification. As cells delaminate from the epiblast and migrate through the primitive streak, they become committed to either a mesodermal or an endodermal cell fate (51). Lineage tracing shows that the definitive endoderm in mice and humans originates from a group of cells at the most distal end of the primitive streak (52). In chick embryos, the endoderm originates from the posterior third of the epiblast (53).
In mice, chimeric embryo experiments demonstrate that TGF-β signaling is required for the specification of the definitive endoderm. In these experiments, Smad2 mutant embryonic stem (ES) cells extensively colonize ectodermal and mesodermal derivatives of the early embryo but are not recruited into the endoderm lineage during gastrulation (54). This reveals that Smad2 signals promote recruitment of epiblast cells into the definitive gut endoderm. FGF signaling has also been implicated during endoderm formation. FGF-4 is expressed in the primitive streak, and gene-targeting experiments demonstrate that FGF-4 is required for initial outgrowth of the epiblast (55). FGF-4 is also capable of inducing endoderm differentiation in a concentration-dependent manner and may therefore act as a posterior morphogen for endoderm in the embryo (56). In addition, FGF receptor 1 (FGFR-1) mutant ES cells fail to populate endodermal derivatives in chimeric embryo experiments (57). A direct role for other growth factors in mammalian endoderm formation remains under investigation.
Neural Origin of Endocrine Cells Refuted
For many years, it was postulated that endocrine cells might originate from the neural crest rather than from the endoderm (58,59,60), a theory based on the shared characteristics of islet and neuronal cells of “amine precursor uptake and decarboxylation” (APUD) (61). The APUD hypothesis received considerable attention because it seemed to be supported by numerous lines of evidence. Endocrine and neural cells express many of the same genes and share cytochemical and ultrastructural characteristics. For instance, pancreatic endocrine cells express the neuronal marker tyrosine hydroxylase, whereas neurons located in the neural tube express the insulin gene (62). In fact, a surprising number of genes are expressed in both tissues, including an L-amino acid decarboxylase (63), a specific acetylcholinesterase (64), a neuron-specific enolase (65), the glycoprotein synaptophysin (66), and the antigens PGP9.5 (67) and A2B5 (68). More recently identified genes that also are found in both tissues include Pax6, Isl1, Nkx2.2, and HNF-3β (see discussion below). Endocrine cells will extend neurites when grown in culture under certain conditions (69,70).
In addition to similar gene expression patterns of neural and endocrine cell types, analogies have been drawn between the mechanisms underlying the specification of these cell types. The neurectoderm and pancreatic endoderm are controlled, at least in part, by inductive interactions with the notochord (see below) (71). In addition, comparative studies in vertebrates and invertebrates have reinforced this intriguing link between endocrine and neural cells (33,72). For instance, somatostatin-expressing cells are found in the paired cerebral ganglia of the flatworm Dugesia lugubris (73). In hornworm moths and blowflies, all four pancreatic endocrine cell types are detected in the brain and the corpus cardiacum (74,75). In the invertebrate chordates, tunicates and amphioxus, both the brain and the gastrointestinal tract contain somatostatin-, PP-, and glucagon-expressing cells (72).
Despite all the evidence for a similarity and coincidence in gene expression, it is a mistake to conclude that cells expressing similar genes derive from a common precursor. Indeed, the neural origin of pancreatic endocrine cells has no direct support from experimental studies and, in fact, the hypothesis has been rendered untenable on the basis of chick-quail chimera lineage studies. In chick-quail chimera experiments, quail neural tube or neurectoderm is grafted to the endomesoderm of the chick and allowed to develop. Invariably, both pancreas and gut endocrine cells are shown to be of chick origin, thus demonstrating that the endocrine cells of the pancreas do not arise as a result of cellular migration from neural tissues (76,77).
PATTERNING OF THE GUT TUBE
Formation of the Gut Tube
In fish, birds, and mammals, the result of gastrulation is the formation of an endodermal sheet, which must be transformed
into a proper gut tube. This sheet overlies the yolk sac and first develops tubelike folds at the anterior and posterior extremities. Once formed, these blind cavities are called the anterior and caudal intestinal portals. As development proceeds, the portals move toward each other and meet at the increasingly constricted yolk stalk, leaving an endodermally lined tube in their wake. Cells originally at the midline of the endodermal sheet now constitute the dorsal midline of the embryonic gut tube. Cells originally at the lateral edges of the sheet are now located on the ventral side of the gut. Gene-targeting experiments reveal that a number of genes are important for this gut folding and closure, including GATA4, its Drosophila homologue Serpent, the proprotein convertase gene Furin, and the bone morphogenic protein genes (BMP1, 2,4,5, and 7) (78,79,80,81,82).
into a proper gut tube. This sheet overlies the yolk sac and first develops tubelike folds at the anterior and posterior extremities. Once formed, these blind cavities are called the anterior and caudal intestinal portals. As development proceeds, the portals move toward each other and meet at the increasingly constricted yolk stalk, leaving an endodermally lined tube in their wake. Cells originally at the midline of the endodermal sheet now constitute the dorsal midline of the embryonic gut tube. Cells originally at the lateral edges of the sheet are now located on the ventral side of the gut. Gene-targeting experiments reveal that a number of genes are important for this gut folding and closure, including GATA4, its Drosophila homologue Serpent, the proprotein convertase gene Furin, and the bone morphogenic protein genes (BMP1, 2,4,5, and 7) (78,79,80,81,82).
Regionalization of the Gut Tube
How is this developing gut endoderm patterned along the anterior-posterior axis? The answer to this question is likely to be complex, and it is only beginning to be addressed. As previously mentioned, it is likely that a certain level of patterning occurs during gastrulation. Immediately after gastrulation, and before formation of the gut tube, the early mouse endoderm already expresses some genes in a regionalized manner. For instance, cerberus-like, Otx1, and Hesx1 are expressed in the anterior endoderm at this time, while the posterior endoderm expresses IFABP and Cdx2 (38,83,84,85,86). The factors that regu-late this early regional expression of genes remain largely unknown, although members of the FGF family have been implicated (38). Numerous studies demonstrate that the endoderm continues to receive signals from adjacent mesoderm and ectoderm long after gastrulation and that these tissues are dependent on reciprocal signaling for proper differentiation (see discussion below). Once the gut tube is formed, it must undergo further anterior-posterior and dorsal-ventral patterning to ensure that prospective organ territories are appropriately assigned and that derivative organs develop at their correct location. Early endoderm regionalization is thus translated into patterning along the gut tube as a requisite prelude to organogenesis. Recent fate-mapping experiments and gene-expression analyses in a number of different organisms indicate that organs have overlapping presumptive domains that become restricted during development (87). The subsequent onset of organogenesis is evident as localized thickening and budding of the endodermal epithelium.
HOX GENES
Hox genes are excellent candidates for patterning molecules in the endoderm. Hox genes are expressed in sequential, overlapping patterns in the vertebrate mesoderm and ectoderm, and particular combinations of these genes lead to positional commitment along the anterior-posterior axis in the limb, vertebrae, and neurectoderm. Recent gene-expression studies in the chick embryo reveal that only a small number of Hox genes can be detected in the endoderm by in situ hybridization (87). However, the anterior expression boundaries of Hox gene expression in the surrounding intestinal smooth muscle match anatomic boundaries in the intestinal epithelium (88,89). When a posterior mesodermal Hox gene, Hoxd-13, is misexpressed in the midgut mesoderm, the underlying endoderm acquires a morphology reminiscent of the hindgut. Therefore, Hox gene-directed positional information exists in the visceral meso-derm and this information is transmitted vertically to the underlying endoderm.
MORPHOGENESIS AND DIFFERENTIATION OF THE PANCREAS
Epithelial Budding
During vertebrate embryogenesis, the first morphologic sign of pancreatic development is an evagination of the dorsal endodermal epithelium and a condensation of the dorsal mesenchyme that lies above it. This pancreatic bud formation is evident at the 22- to 25-somite stage or E9.5 in mice (4,34), at the 30-somite stage or stage 15 in chickens (90,91), and during the fourth week of gestation in humans (92). Subsequently, approximately 12 hours later, the ventral bud becomes evident (Fig. 2.6A,B). The dorsal bud arises from the endodermal epithelium caudal to the stomach anlage and slightly caudal to the biliary duct. At this point, the liver has developed into a trilobular structure and is joined to the duodenum via the biliary duct. The ventral bud arises from epithelium at approximately the same anterior-posterior location as the dorsal bud but on the ventral side of the gut tube at the base of the biliary duct. Some organisms (such as the rat and human) have only one ventral bud and others have two (chick and frog) (93). In the mouse, two ventral buds are detectable transiently during bud evagination (93; E Lammert and DA Melton, unpublished observations).
Branching and Bud Fusion
As development proceeds, the dorsal pancreatic bud begins to proliferate and branch, progressively forming a tree-like structure (Figs. 2.7 and 2.8) (5,6). This process begins around the 25- to 35-somite stage in mice (E10.5) and the 26-somite stage in chicks and continues throughout pancreatic development (6,90). Initially, the branching epithelium appears as a solid tissue; however, it actually consists of a compact bulb of highly folded epithelium. It has been hypothesized that branching occurs because the number of cells in the wall of the diverticulum increases while the bud remains the same size (6), causing lateral pressure until the epithelium buckles and produces a digitation. Unlike the branching that occurs in kidney epithelium, including both lateral and terminal bifid branching, or the asymmetric branching of lungs, pancreatic branching is not stereotyped (94,95).
After the 25-somite stage in mice (E10), mesodermal mesenchyme continues to condense around the pancreatic bud and accumulates on the left side of the gut tube, breaking the symmetrical morphology of the pancreatic rudiment (4). At this stage, islet structures begin to protrude from the pancreatic epithelium (14). Bud growth and elaboration is followed by a constriction at the base of the pancreatic diverticulum, near the duodenum, and formation of a narrow stalk (4). As development proceeds, between E11 and E12, the gut tube bends and rotates, swinging the ventral bud dorsally and bringing it into immediate proximity to the dorsal bud (Fig. 2.6C,D). The dorsal and ventral buds then fuse sometime around E13 (96). In humans, mice, and rats, this fusion occurs between a dorsal bud and a single ventral bud, whereas in chicks and amphibians, the dorsal bud fuses with paired ventral buds. At E14.5, acini and ducts become clearly visible as distinct structures embedded within the more abundant exocrine tissue. Subsequently, the pancreas continues to grow and extend into adulthood (Fig. 2.6E,F).
Dorsal and Ventral Bud Derivatives
The dorsal and ventral buds later develop into different portions of the mature pancreas. The dorsal bud connects to the duodenum via the duct of Santorini and forms the upper part
of the head of the pancreas; the body; and the tail, or splenic portion, of the pancreas. The ventral bud connects to the duodenum via the duct of Wirsung and forms the lower part of the head of the pancreas, or the uncinate process. Generally, after the fusion of the primordia, the dorsal pancreas appropriates the duct of the ventral pancreas proximally and it becomes the primary pancreatic duct, or the duct of Wirsung. More distally, the dorsal pancreas sometimes retains its own connection to the duodenum, or the duct of Santorini. Many of the smaller epithelial ductules anastomose during the fusion process, leading to functional connections between tissue of dorsal and ventral origin (97).
of the head of the pancreas; the body; and the tail, or splenic portion, of the pancreas. The ventral bud connects to the duodenum via the duct of Wirsung and forms the lower part of the head of the pancreas, or the uncinate process. Generally, after the fusion of the primordia, the dorsal pancreas appropriates the duct of the ventral pancreas proximally and it becomes the primary pancreatic duct, or the duct of Wirsung. More distally, the dorsal pancreas sometimes retains its own connection to the duodenum, or the duct of Santorini. Many of the smaller epithelial ductules anastomose during the fusion process, leading to functional connections between tissue of dorsal and ventral origin (97).
The dorsal and ventral anlagen, as well as their adult derivative portions of the mature pancreas, differ in their component cell types. The dorsal pancreas develops larger insulin-secreting islets that contain more insulin-producing and glucagon-producing cells and fewer PP-producing cells than do those of the ventral pancreas. The ventral pancreas develops mainly into exocrine acinar tissue interspersed with smaller islets that contain many more PP-cells (98). One study determined that the dorsal pancreas is composed of 82% β-cells, 13% α-cells, 4% δ-cells, and 1% PP-cells and that the ventral pancreas is composed of 79% PP-cells, 18% β-cells, 2% δ-cells, and 1% α-cells (99). However, the relative concentration of islets is more or less equivalent in the two tissues (100,101).
Normal Development of Embryonic Islets
Morphologically distinguishable islets of Langerhans arise late during embryogenesis (Fig. 2.9). However, the endocrine cells that will aggregate to form islets appear much earlier. The process by which these endocrine precursors delaminate from the endoderm, migrate, and coordinate to form a functional islet is one that has been noted repeatedly during the past century in fish, amphibians, birds, and mammals. Initially, endocrine precursors are observed as scattered individual cells, often still embedded in the epithelium of the ducts (16,97). These precursors have recently been referred to as islet precursor
cells and are thought to be multipotent (102). The first endocrine cells that can be identified in the developing pancreas are the α-cells or glucagon-expressing cells (30). Insulin-expressing cells are detectable soon after. These endocrine precursors remain associated with the ducts as individual cells until well into embryogenesis—as late as E15.5 in the mouse. These cells then begin to dissociate from the duct epithelium, migrate a short distance away from the ducts, and aggregate into small clusters. Shortly before birth, compact islets have formed and are recognizable by their characteristic concentric arrangement of β-cells surrounded by α, δ, and PP endocrine cell types. Studies with aggregation mouse chimeras clearly indicate that islets are not clonally derived but are rather the result of the clustering of individual endocrine cells or clusters of cells that arise independently of each other (103).
cells and are thought to be multipotent (102). The first endocrine cells that can be identified in the developing pancreas are the α-cells or glucagon-expressing cells (30). Insulin-expressing cells are detectable soon after. These endocrine precursors remain associated with the ducts as individual cells until well into embryogenesis—as late as E15.5 in the mouse. These cells then begin to dissociate from the duct epithelium, migrate a short distance away from the ducts, and aggregate into small clusters. Shortly before birth, compact islets have formed and are recognizable by their characteristic concentric arrangement of β-cells surrounded by α, δ, and PP endocrine cell types. Studies with aggregation mouse chimeras clearly indicate that islets are not clonally derived but are rather the result of the clustering of individual endocrine cells or clusters of cells that arise independently of each other (103).
INDUCTION OF PANCREATIC CELL FATE
Commitment to Pancreatic Cell Fate
The initiation of pancreatic commitment to a pancreatic cell fate occurs before overt morphogenesis of the pancreas. Mouse pancreatic buds cultured in vitro will grow and branch, revealing that foregut epithelial cells of the early pancreatic bud are already programmed to take on a pancreatic fate (4). However, the pancreas is said to be committed even earlier, at the 8- to 12-somite stage in mice, or E8.5 (4), and the 13-somite stage in chickens, or stage 11 (98). This commitment has been shown in experiments involving the culture of isolated mouse foregut tissues. For example, when the pancreatic endoderm and associated mesenchyme are excised from an eight-somite mouse embryo and cultured in isolation, morphologically distinct exocrine tissue develops, with acinar cells filled with distinguishable zymogen granules. This commitment is likely to be influenced by both intrinsic and extrinsic factors. The endoderm alone is said to be committed by the 15-somite stage, at which time it has the capacity to form exocrine tissue in response to signals from heterologous mesenchyme. In vitro culture of committed pancreatic endoderm in combination with either pancreatic or heterologous mesenchyme leads to the differentiation of pancreatic cell types; however, culture of isolated endoderm does not (4,34,96,104). This shows that the competence to form pancreas is intrinsic to the pancreatic epithelium but that inductive signals from adjacent mesenchyme or other tissues are required.
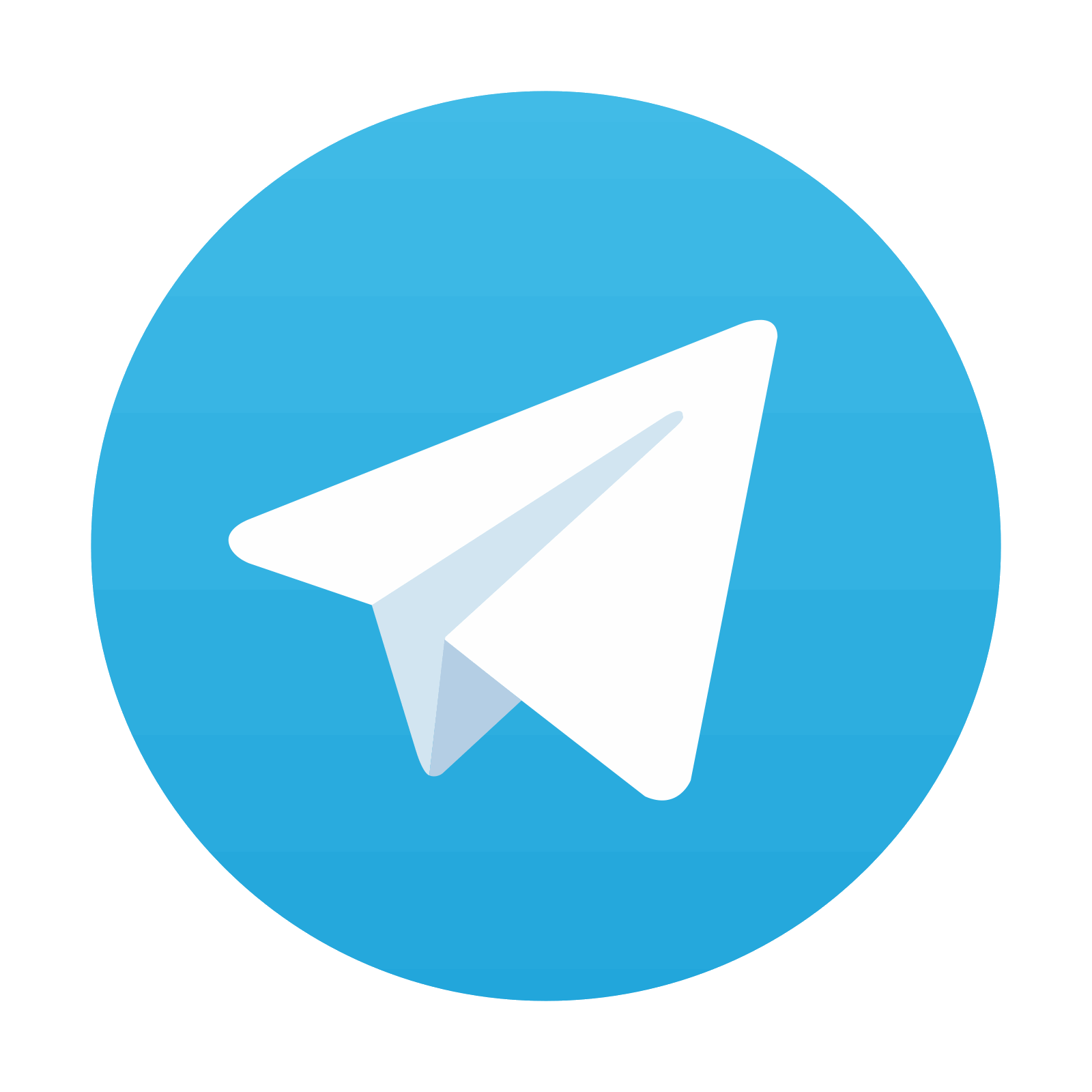
Stay updated, free articles. Join our Telegram channel
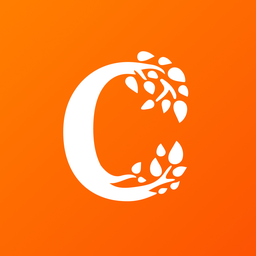
Full access? Get Clinical Tree
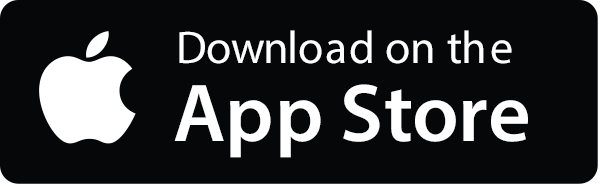
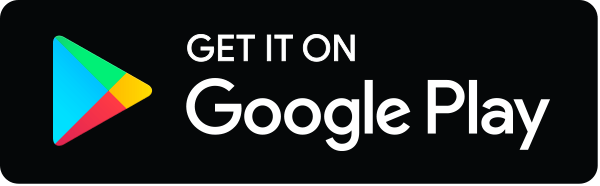