Corticosteroids in Treatment of Allergic Diseases
Sai R. Nimmagadda
History of Corticosteroids
Corticosteroids—the synthetic analogs of the glucocorticoid hormones of the adrenal cortex—have emerged as the single most effective class of drugs for treatment of inflammatory diseases. Although it was as early as 1885 that Addison described a “wasting disease” after destruction of the adrenal gland (1), it wasn’t until the 20th century that researchers defined the activity of the adrenal steroids (2). In 1949, Hench et al. introduced corticosteroid treatment for arthritis and other diseases (3,4), which soon expanded to the use of corticosteroids as treatments for nearly all inflammatory diseases. Enthusiasm for systemic corticosteroid therapy waned with the discovery that chronic use caused numerous debilitating adverse effects, but the 1957 introduction of topically active corticosteroids with greatly diminished side effects (5) renewed interest in their widespread use.
Inhaled corticosteroid (ICS) therapy was introduced in the 1970s, initially targeted to patients with severe asthma who required treatment with oral corticosteroids (6,7). Later, ICS therapy was extended to patients for whom sympathomimetics and methylxanthines were ineffective (8), but the major focus was still on patients with severe disease.
The recognition of asthma as an inflammatory disease changed treatment strategy. Reports showing similarities in infiltrations of lymphocytes, mast cells, and eosinophils in the bronchial mucosa, regardless of the severity of asthma (9,10), extended ICS use to populations with only mild persistent disease (11). Clinical reports showing greater reductions in bronchial hyperresponsiveness with the regular use of an ICS in comparison with the regular use of an inhaled β2 agonist (12,13) added to the impetus. In 1991, the Guidelines for the Diagnosis and Management of Asthma of the National Asthma Education and Prevention Program (NAEPP) recommended ICS therapy for patients with both severe and moderate asthma (14). By 1997, the recommendations also included patients with mild persistent disease (15), and the 2007 Guidelines state that ICSs are the most consistently effective long-term control medications at all steps of care for persistent asthma in both children and adults, regardless of severity (16).
Pharmacology of Corticosteroids
There are two general classes of corticosteroids: mineralocorticoids (MCs) and glucocorticoids (GCs). MCs principally affect the regulation of fluid and electrolyte balance and have no clinical use in the treatment of allergic disease. However, MC activity in corticosteroid medications may produce fluid and electrolyte side effects, so they are not entirely without relevance.
The basic chemical structure of GCs consists of 21 carbon atoms with a total of four rings, three six-carbon rings and a five-carbon ring (17). Hydrocortisone (cortisol) is the parent molecule from which other natural and synthetic GCs derive. Essential features of the anti-inflammatory GC consist of the following: (a) a two-carbon chain at the 17th position, (b) methyl groups at carbons 10 and 13, (c) a ketone oxygen at C3, (d) an unsaturated bond between C-4 and C-5, (e) a ketone oxygen at C-20, and (f) a hydroxyl group at C-11. Modifications of either the nucleus or the side chains produce different GC agents with varying anti-inflammatory and MC activity as compared to cortisol. Further alterations at the C-17 and C-21 positions result in corticosteroids with high topical activity and minimal systemic adverse effects.
Cortisol secretion results from a cascade of stimulatory events in the hypothalamic-pituitary-adrenal (HPA) axis (18). The process begins in the hypothalamus with the secretion of corticotropin-releasing factor (CRF), which stimulates the release of adrenocorticotropic hormone (ACTH), a product of the basophil cells of the anterior pituitary gland. In turn, ACTH
stimulates the production of GCs, which are primarily produced in the zona fasciculata of the adrenal cortex. Cortisol and ACTH secretion normally reaches peak levels in the early morning, then declines throughout the day to a low point in the early-to-late evening (2). Daily secretion of cortisol is about 10 mg to 20 mg (28 μmol to 55 μ (mol), but environmental stress or increased circulating levels of cytokines, such as interleukin (IL)-1, IL-2, IL-6, or tumor necrosis factor-α (TNFα), can raise levels to as high as 400 mg to 500 mg (19).
stimulates the production of GCs, which are primarily produced in the zona fasciculata of the adrenal cortex. Cortisol and ACTH secretion normally reaches peak levels in the early morning, then declines throughout the day to a low point in the early-to-late evening (2). Daily secretion of cortisol is about 10 mg to 20 mg (28 μmol to 55 μ (mol), but environmental stress or increased circulating levels of cytokines, such as interleukin (IL)-1, IL-2, IL-6, or tumor necrosis factor-α (TNFα), can raise levels to as high as 400 mg to 500 mg (19).
At least 90% of circulating cortisol is protein-bound, principally to cortisol-binding globulin or transcortin (17). The unbound fraction is biologically active and may bind to transcortin (high affinity/low capacity) or to serum albumin (low affinity/high capacity). Transcortin has a binding capacity of only 0.7 μmol (250 μg) cortisol per liter serum. Thus at low concentrations, approximately 90% of cortisol is plasma-protein-bound, and at higher concentrations of cortisol, transcortin binding becomes saturated. Some synthetic GCs, such as dexamethasone, exhibit little or no binding to transcortin. Because pharmacologic actions, metabolism, and excretion of corticosteroids all relate to unbound steroid concentrations, the binding of circulating steroids to transcortin and albumin play important roles in modifying GC potency, half-life, and duration of effects (2).
The intrinsic pharmacokinetic properties of GCs are described by their volume of distribution (absorption) and clearance (metabolism, half-life, or excretion). Other factors may also include pro-drug conversion by pulmonary esterases to an active GC metabolite. For a specific corticosteroid, bioavailability is also part of the equation (Tables 35.1 and 35.2).
Table 35.1 Pharmacokinetic Variables and Equivalent Doses of Common Oral Glucocorticosteroids | ||||||||||||||||||||||||||||||||||||||||||
---|---|---|---|---|---|---|---|---|---|---|---|---|---|---|---|---|---|---|---|---|---|---|---|---|---|---|---|---|---|---|---|---|---|---|---|---|---|---|---|---|---|---|
|
Table 35.2 Pharmacokinetic Variables of Common Inhaled and Intranasal Glucocorticoids | ||||||||||||||||||||||||||||||||||||||||||||||||||||||||||||||||||||||||||||||||
---|---|---|---|---|---|---|---|---|---|---|---|---|---|---|---|---|---|---|---|---|---|---|---|---|---|---|---|---|---|---|---|---|---|---|---|---|---|---|---|---|---|---|---|---|---|---|---|---|---|---|---|---|---|---|---|---|---|---|---|---|---|---|---|---|---|---|---|---|---|---|---|---|---|---|---|---|---|---|---|---|
|
Natural and synthetic steroids are lipophilic compounds readily absorbed after intravenous, oral, subcutaneous, or topical administration. However, lipophilicity varies among preparations. In general, the systemic availability of both oral and intravenous GC preparations is high and is limited by first-pass liver metabolism rather than by incomplete absorption. However, with inhaled GCs, the pharmacokinetic profile and the method of delivery determine the extent and time to systemic absorption of a given GC. A portion of a dose of ICS is swallowed—unless rinsed out—and absorbed from the gastrointestinal (GI) tract. The rest reaches the lower airways and exerts the desired effect. The ratio of desirable/undesirable effects depends on:
Topical activity of drug in the airways (GC receptor-binding affinity)
Percentage of oropharyngeal versus lower airway deposition
Systemic activity of drug after absorption by the GI tract or lungs and first-pass metabolism (20)
Activation or conversion of a GC to an active metabolite or compound
Catabolism of corticosteroids is mainly in the liver, although other organs such as the kidney, placenta, lung, muscle, and skin may contribute to the metabolism of endogenous and synthetic GCs. Enzymatic coupling with a sulfate or glucuronic acid forms water-soluble compounds, which leads to renal excretion. There is minimal excretion via the biliary and fecal routes.
Molecular and Anti-Inflammatory Mechanisms of Glucocorticoid Action
As anti-inflammatory agents, GCs exert both direct and indirect inhibitory effects on multiple inflammatory genes (encoding cytokines, chemokines, adhesion molecules, inflammatory enzymes, receptors, and proteins) that have been activated during the inflammatory process (21).
Glucocorticoids diffuse readily across cell membranes and bind to glucocorticoid receptors (GRs) in the cytoplasm (22). The GR is a 94-kD protein which exists in the cytoplasm as a multiprotein complex
containing several heat shock proteins (Hsp90, Hsp70, Hsp56, and Hsp40) (23). These heat shock proteins protect the receptor and prevent its nuclear localization by covering the sites on the receptor that are needed for transport across the nuclear membrane into the nucleus (23). Once corticosteroids have bound to GRs, changes in the receptor structure result in rapid transport of the GR-corticosteroid complex into the nucleus where it binds as a homodimer to specific DNA-binding sites, i.e., glucocorticoid responsive elements (GREs) (24). The relative potency of GCs is dependent on plasma protein-binding, intracellular receptor affinity, and receptor dissociation from activated receptors.
containing several heat shock proteins (Hsp90, Hsp70, Hsp56, and Hsp40) (23). These heat shock proteins protect the receptor and prevent its nuclear localization by covering the sites on the receptor that are needed for transport across the nuclear membrane into the nucleus (23). Once corticosteroids have bound to GRs, changes in the receptor structure result in rapid transport of the GR-corticosteroid complex into the nucleus where it binds as a homodimer to specific DNA-binding sites, i.e., glucocorticoid responsive elements (GREs) (24). The relative potency of GCs is dependent on plasma protein-binding, intracellular receptor affinity, and receptor dissociation from activated receptors.
After binding to GREs in the DNA, GCs can promote (transactivate) or inhibit (transrepress) gene expression (25). The number of genes directly regulated by GRs in any given cell is unknown, but studies place the number of steroid-responsive genes per cell at 10 to 100 (26,27). In addition, many genes are indirectly regulated through an interaction with other transcription factors and co-activators. The mechanisms of action of GCs are mediated by genomic effects, secondary nongenomic effects, and interactions with cellular-membrane-bound GRs (28). The classic genomic mechanism of GC action results in the following:
Transactivation: production of Annexin-1 (lipocortin-1) SLPI (secretory leukoprotease inhibitor), MKP-1 (mitogen-activated kinase phosphatase-1), IκB-α, glucocorticoid-induced leucine zipper protein (GILZ), and the ß2 adrenoceptor (21).
Transrepression: upregulation or downregulation of transcription factors that alter specific messenger ribonucleic acid (mRNA) production, which results in increased production of anti-inflammatory mediators and proteins and decreased production of proinflammatory mediators, including cytokines, chemokines, and adhesion molecules (21). Theoretically, high levels of transcription factors could suppress GC action by neutralizing receptors. This occurrence could be a potential mechanism of GC resistance (21).
Cisrepression: GR homodimer interaction with GREs to suppress genes associated with side effects of GC. These include: proopiomelanocortin (POMC), corticotrophin-releasing factor-1 (CRF-1), osteocalcin, and keratin (21).
These mechanisms are now thought to be among the most important in explaining GC anti-inflammatory action, but other factors come into play as well. Glucocorticoids hinder the recruitment and activation of T-lymphocytes, eosinophils, dendritic cells, macrophages, and other inflammatory cells, and they inhibit the survival of mast cells at the airway surface, though they do not prevent their activation (29). Airway epithelial cells are likely major targets for inhaled GCs because these cells release numerous inflammatory mediators (29).
Corticosteroid Therapy
Regardless of the route of administration, a general rule of thumb with GC therapy is that clinicians should use the lowest possible effective dose for the shortest time, and patients should undergo frequent reevaluation with the goal of eliminating GCs or reducing dosages. Complications of GC therapy relate to the pharmacology of the agent, dose, dosing interval, and duration of use. Local administration—topical cutaneous or inhaled nasal/bronchial—is recommended where possible to avoid or reduce systemic side effects. These eight broad principles apply:
If possible, treatment agents should have little or no MC activity.
Patients with nonlife-threatening disorders, e.g., atopic dermatitis or nasal polyps, should undergo long-term systemic GC therapy only when alternative and more conservative therapy has failed.
To facilitate rapid, safe reductions in dose and use of prolonged courses of systemic GC therapy, patients should receive concurrent maximal doses of topical preparations.
Single-dose oral GCs should be given in the morning to minimize disruption of the HPA axis.
Acute allergic disease exacerbations can usually be safely treated with 5- to 10-day courses of moderate-dose daily systemic GC therapy without significant adverse effects.
For alternate-day systemic GC therapy, the best choices are oral agents with tissue half-lives in the 12- to 36-hour (intermediate) range, such as prednisone, prednisolone, and methylprednisone.
Children receiving GC therapy should be regularly evaluated for growth, especially those using both intranasal and inhaled GC therapy.
All patients on GC treatment should undergo frequent reevaluation to attempt to reduce the dosage or eliminate steroids altogether.
Inhaled Corticosteroids and Asthma
The National Heart, Lung and Blood Institute Expert Panel Report 3 (EPR-3) guidelines recommend a stepwise approach for the treatment of persistent asthma (16,30). The guidelines stress (a) assessing severity before initiating treatment and (b) periodically assessing asthma control to adjust therapy, stepping up if necessary, and stepping down if possible (30,31). In almost all patients, with the possible exception of those with mild, intermittent symptoms, ICS therapy remains the first-line treatment in patients of all ages. Treatment with ICSs reduces local inflammation and bronchial hyperresponsiveness, improves pulmonary function, reduces or eliminates the need for oral steroids, decreases hospitalizations, and asthma mortality (30,32). It has been reported that low-dose ICSs reduced the risk of death in asthma by 50% when patients used at least six canisters per year of ICS (33).
While GCs have been known to reduce inflammation and control symptoms of asthma, there is mounting evidence that they are not disease-modifying agents. Studies have suggested that early use of GCs in children as young as 2 years of age can reduce symptoms and exacerbations (34). However, 2 years of GC therapy did not change the development of asthma symptoms or lung function after treatment was discontinued. Early use of inhaled fluticasone propionate for wheezing in preschool children had no effect on the natural history of asthma or wheeze later in childhood (35). Furthermore, it appears that ICSs do not prevent lung function decline or reduce airway reactivity in preschool children (34,36).
Current knowledge of the mechanisms of asthma suggests that the prudent treatment strategy is early introduction and relatively high initial doses of ICSs to gain maximum control quickly, followed by dose reduction to the minimum needed to maintain control. Contrary to the earlier belief that appropriate treatment would reverse airway obstruction, current evidence suggests that “airway remodeling,” i.e., structural changes and irreversible airway obstruction, occurs with chronic inflammation (37–42).
Some recent research postulates that inflammation and airway remodeling may not be as closely connected as many have believed. Bush suggests that eosinophilic inflammation and airway remodeling are parallel processes and that the primary abnormality is not airway inflammation but some form of disordered airway repair (43). In a review, Warner and Knight also found evidence that features of remodeling such as angiogenesis, goblet cell hyperplasia, and thickened lamina reticularis occur early in the disease and independently of inflammation (44). Another study presented evidence that the airway epithelium in asthma is fundamentally abnormal and has increased susceptibility to environmental injury and impaired repair (45).
Clearly, the study of the cause and effects of airway remodeling is still in its infancy. However, current research favors the early introduction of ICS therapy in all patients, given that irreversible damage can occur and that permanent changes may be preventable. Even in patients with mild persistent disease, studies show that the lowest effective dose of ICS therapy is safe, well-tolerated, and cost-effective (46–49).
Inhaled Corticosteroid Preparations
Seven ICS preparations are currently available for the treatment of asthma in the United States. They are: beclomethasone dipropionate (BDP), flunisolide (FL), fluticasone propionate (FP), triamcinolone acetonide (TA), budesonide (BUD), mometasone furoate (MF), and ciclesonide (CIC).
Not all ICSs have the same efficacy and safety profiles, however. Their efficacy and safety are shaped by their pharmacokinetic and pharmacodynamic effects. Characteristics that enhance the efficacy of ICSs include high glucocorticoid-receptor-binding affinity, small particle size, prolonged pulmonary residence time, and lipid conjugation (50). Characteristics that enhance the safety of an ICS include minimal oral bioavailability, on-site activation in the lung, low oropharyngeal exposure, high protein binding, and rapid systemic clearance (30,50). Clinicians should consider these characteristics when choosing an appropriate ICS. Pharmacokinetic variables are summarized in Table 35.2.
Reviews of the literature are helpful, but clinicians should interpret comparative studies with caution because study parameters may differ in their methods of measuring adverse effects and their choices of delivery device. Either of these can result in false comparisons. A better method of comparing various ICSs or a single drug in different formulations is the therapeutic index, which is the ratio of desirable-to-undesirable effects. Desirable topical effects would include potency, the amount of GCs delivered to the lung, and pro-drug conversion. Undesirable effects would be due to mineralocorticoid activity, rate of clearance from the body, and the bioavailability of the GC after lung and gastrointestinal absorption. First-pass metabolism of the swallowed fraction of the GC is also of great importance in determining drug choice in asthma therapy.
Most newer ICS products have low oral bioavailability (Table 35.2). Budesonide, MF, and FP have a lower oral bioavailability than BDP because of their extensive hepatic first-pass metabolism, but studies suggest that the oral deposition of CIC is significantly less than with either FP (51) or BUD (52). The lower the oral bioavailability, the fewer the systemic side effects at equivalent doses. The relative anti-inflammatory potency of the ICS from most-potent to least-potent can be summarized as follows: CIC = FP = MF > BUD = BDP >TA = FL.
Delivery Devices
The type of delivery device plays an important role in determining the amount of drug delivered to the lungs; Chapter 38 reviews delivery devices in more detail. Lung deposition is influenced by the inhalation device, propellant, particle size, i.e., mass mean aerodynamic diameter (MMAD), and by whether the solution is an aerosol or suspension. Delivery devices are the metered-dose inhaler (MDI), dry powder inhaler (DPI), and the nebulizer (for infants, young children, and the elderly). Ease of use and less-frequent dosing are other factors to consider, as they lead to better compliance (Tables 35.3 and 35.4). Some devices have multidose capabilities. In MDIs, which may be either breath-activated or pressurized, hydrofluoroalkane (HFA) propellants have largely replaced chlorofluorocarbon (CFC) propellants due to a worldwide mandate. A spacer may be used with CFC/HFA-propelled MDIs to reduce oropharyngeal deposition.
Table 35.3 Comparison of Drug Deposition with Metered-dose Inhalers/ Hydrofluoroalkane and Dry-powder Inhalers (%) | ||||||||||||||||||||||||||||||||||||||||||||||||||||||||||||||||||
---|---|---|---|---|---|---|---|---|---|---|---|---|---|---|---|---|---|---|---|---|---|---|---|---|---|---|---|---|---|---|---|---|---|---|---|---|---|---|---|---|---|---|---|---|---|---|---|---|---|---|---|---|---|---|---|---|---|---|---|---|---|---|---|---|---|---|
|
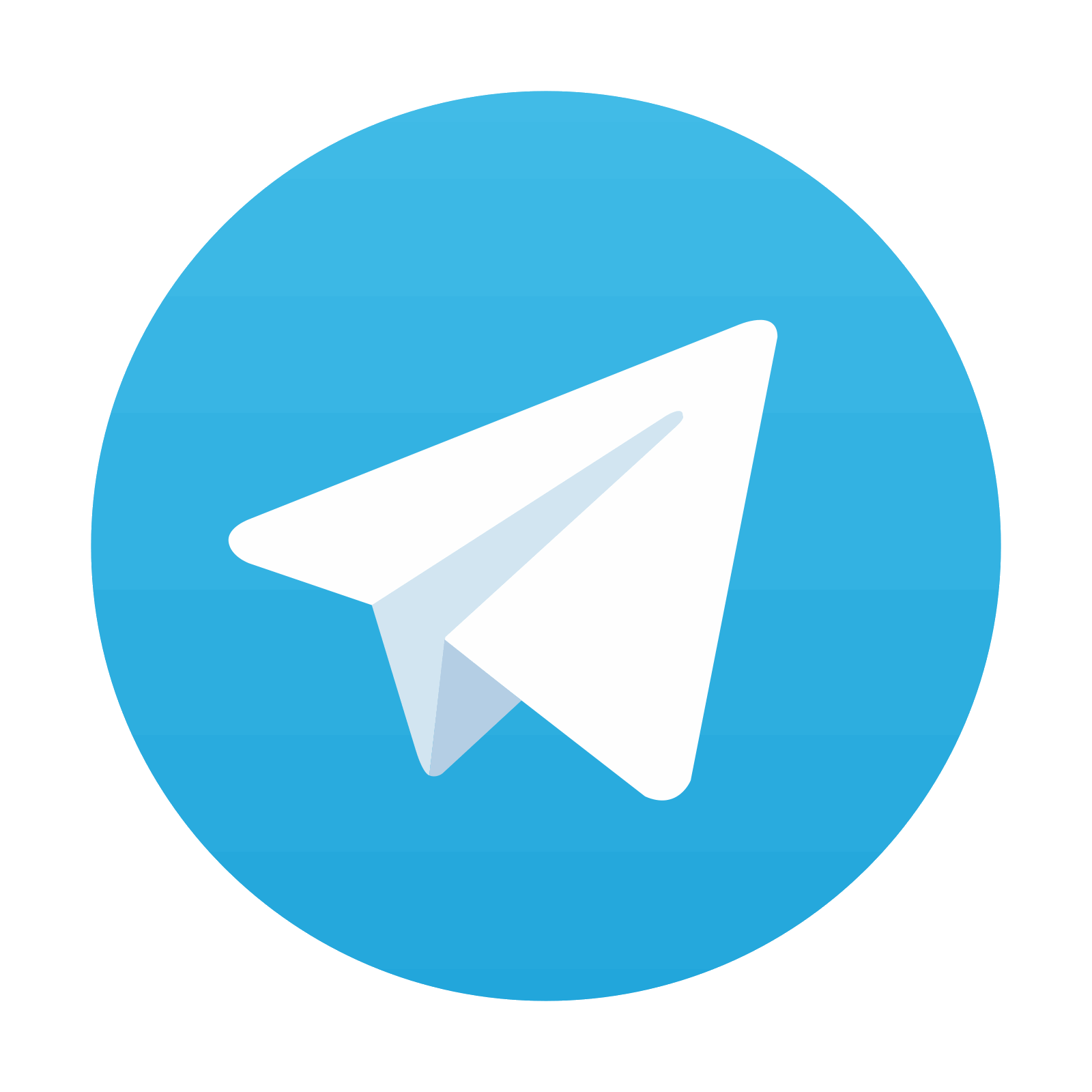
Stay updated, free articles. Join our Telegram channel
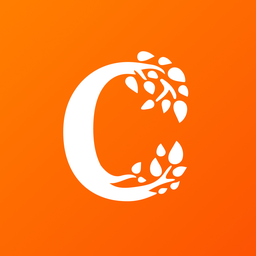
Full access? Get Clinical Tree
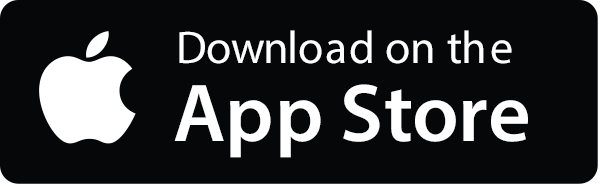
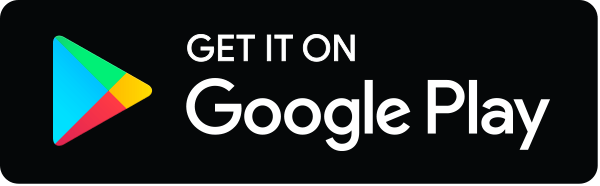
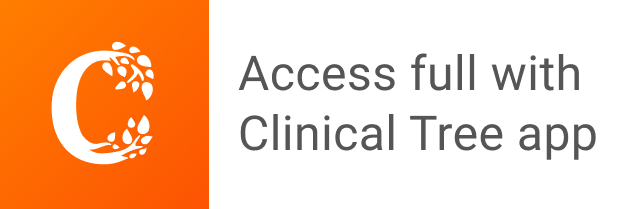