As a discipline, radiation oncology primarily deals with the use of ionizing radiation in the treatment of malignant diseases. Occasionally therapeutic doses of radiation may be used to treat benign processes, such as heterotopic bone formation, meningiomas, and other benign proliferative diseases. Radiation has a very prominent role in the management of breast cancer after lumpectomy, as adjuvant therapy after mastectomy, in the management of locoregional relapse of disease, and in the palliation of metastatic disease. Management of breast cancers can typically cover up to 20% to 25% of a general radiation oncology practice. The next few chapters will cover in more detail the role of radiation therapy after breast conserving therapy, the rapidly evolving role of partial breast irradiation, postmastectomy radiation, and the role of radiation in palliation and metastatic disease.
A basic review of the underlying principles of the physics, radiation biology, and radiation planning is essential to understanding the role of radiation therapy in the management of breast cancer. It is beyond the scope of this text to comprehensively review the underlying principles of radiation physics and biology, and the reader is referred to more comprehensive textbooks for more detailed information.1-5 However, in this chapter we will highlight some of the basic principles of physics and radiation biology and outline some of the basics of radiation planning to give the reader an appreciation of the underlying principles, rationale, and process of radiation in the management of breast cancer.
Radiation therapy uses a form of electromagnetic radiation, commonly referred to as ionizing radiation, generated from x-rays, gamma rays, electrons, and other forms of particles. Ionizing radiation consists of highly energetic particles that can eject at least 1 electron from an atom. Ionizing ability depends on the energy of individual particles or waves. Although x-rays or gamma rays are the principle form of ionizing radiation used in conventional radiation therapy, other forms of ionizing radiation include protons, beta particles, neutrons, alpha particles, and heavy ions. The ability of photons to ionize an atom or molecule varies across the electromagnetic spectrum. Although x-rays and gamma rays have high enough energy to ionize almost any molecule or atom, near ultraviolet and visible light are ionizing to very few molecules, and microwaves and radiowaves are nonionizing radiation within the electromagnetic spectrum.3,4
Ionizing radiation ejects electrons from atoms. These fast electrons continue on to produce additional ionizations, amplifying the effect of the initial photon interactions. One of the more critical and essential interactions of x-rays is the creation of ion pairs from interactions with the abundant water in cells and tissues that lead to the formation of free radicals. This process represents the primary mechanism through which damage is created in irradiated cells. Certain substances, such as antioxidants or high doses of certain vitamins, theoretically can absorb some of these free radicals, thereby counteracting the effects of radiation.6,7
Although radiation interaction in human tissues can damage a variety of cellular components, DNA remains the essential and primary target of radiation in the management of malignant disease. DNA double-strand breaks, caused by ionizing radiation interaction in cells, likely represent the most critical lesions. As discussed below, lack of repair of double-strand breaks, or misrepair, results in cell death and other biologic effects of radiation. The chemical and biological changes in tissue result from exposure to ionizing radiation, caused by the deposition of energy from the radiation into the tissue. The absorbed dose is the energy actually absorbed per unit mass in the irradiated volume. The unit of absorbed dose is the Gray (Gy), defined as 1 J/kg of irradiated medium.3-5
The practice of radiation therapy began very soon after Roentgen’s discovery of x-rays in the 1890s. Within just a few years of the discovery of x-rays, reports of altering the course of malignant disease and palliation of malignancy with the use of therapeutic doses of radiation appeared in the early literature.3 External-beam radiation units are commonly categorized by the energy range of the beam: contact units (40-50 kV), superficial (50-150 kV), orthovoltage (150-500 kV), supervoltage (500-1000 kV), and megavoltage (> 1000 kV or > 1 MV). In general, the higher the energy, the more penetrating the beam will be. Although higher-energy beams have advantages with respect to sparing skin and treating more deeply seated tumors, in the earlier years of therapy, most radiation therapy was delivered with x-rays generated at relatively low-energy units below approximately 300 kV, due to the limitations of the available technology of the time.
It was not until the 1950s and the availability of high-activity sources of cobalt 60 (60Co) that megavoltage units came into widespread use. Cobalt units contain a source of 60Co, which emits gamma rays of 1.17 and 1.33 MV, and were therefore more suitable for treating more deeply seated tumors while providing some sparing of the skin, as compared with the lower-energy orthovoltage units. Although linear accelerators have replaced cobalt units in the majority of facilities in the United States, cobalt units continue to be used in many facilities throughout the world. Because the cobalt source is always active and cannot be turned on or off, the radiation beam is turned on and off by moving the source from a shielded position to an exposed position. Although linear accelerators have predominantly replaced cobalt units in the majority of external-beam facilities, cobalt sources are still routinely used in the “gamma knife” stereotactic radiation device commonly used to deliver stereotactic radiosurgical treatments in a variety of brain applications.
When the megavoltage cobalt units were being developed, many were manufactured with isocentric gantries. The gantry of an isocentric unit rotates about a horizontal axis, so that the source remains in a plane perpendicular to this axis, and the radiation beam always passes through a single point on the axis in space, known as the isocenter, around which both treatment table and collimator also rotate, as shown in Figures 93-1A through C. This is the fundamental design of current modern linear accelerators as well.
Modern linear accelerators (also known as linacs) are now found in virtually all radiation therapy departments and have replaced most low-energy x-rays units and isotope teletherapy units. Most medical linacs accelerate electrons and the resulting electron beam strikes a target, producing x-rays that are collimated and directed to the patient. Modern linear accelerators generate x-ray beams generally ranging from 4 to 18 MV, with the use of 6-MV photons being most commonly used for external-beam whole-breast irradiation. Many of the current machines have 2 energies to choose from, as more deeply seated tumors, such as prostate or pelvic tumors, may be better treated by more penetrating beams of 10 MV or more. Figures 93-2A through C show a modern linear accelerator with a patient under treatment for breast cancer.
Figure 93-2
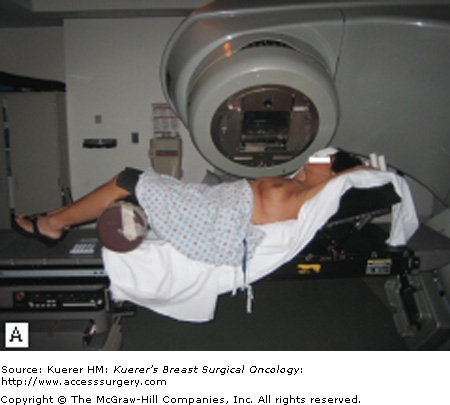
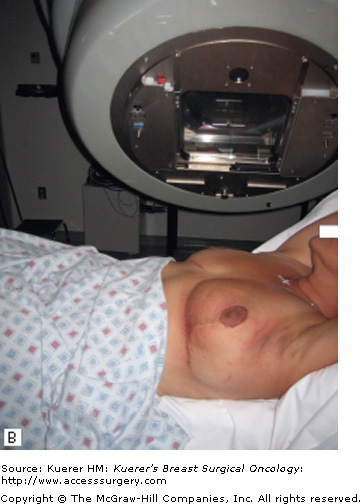
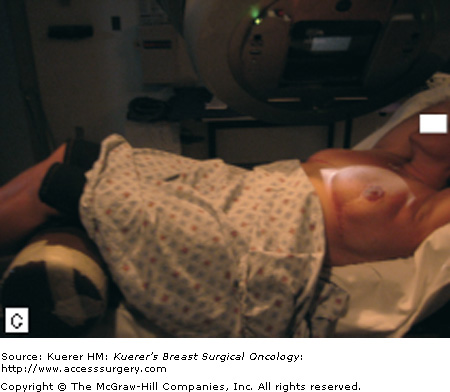
A. A breast cancer patient is receiving external-beam radiation treatment on a modern linac. B. Close-up of a patient under external-beam radiation treatment on a modern linac. C. Close-up of a patient under external-beam radiation treatment on a modern linac, showing 1 of the treatment light fields.
Although linear accelerators most commonly operate in the photon or x-ray mode, the accelerated electron beam can be focused directly at the patient in the electron mode. Here, the target and flattening filter are moved out of the electron field, and the electron beam is directed through a scattering foil and then directly to the patient for electron beam therapy. The direct electron beam is not as penetrating as x-rays, and the dose falls off fairly rapidly from the surface of the patient. The penetration of an electron beam compared with that of a photon beam in tissue is shown in Figure 93-3, where the dose in tissue is near maximum at the surface for an electron beam, then rapidly falls off. For a photon beam, the skin is spared because the dose starts to buildup just below the skin surface, reaches its maximum at a certain depth, then gradually falls off. Therefore, electrons are useful in treating the skin and near the surface of the patient, while sparing deeper underlying tissues and as such are ideally suited to boost the tumor bed after whole-breast irradiation, to boost the scar after mastectomy, and by some, to treat the chest wall after mastectomy.
Most electron accelerators consist of a microwave power source (magnetron); a linear accelerator guide to accelerate the electrons; a bending magnet; an x-ray target, which the electrons hit to produce the x-rays; a flattening filter, which filters and flattens the beam for uniform clinical use; an ionization chamber to monitor dose delivered; and a collimator to delineate the final dimensions of the field. Fields could be further shaped and customized to the specific clinical situation with blocks, generally made from cerrobend, which were placed between the collimator and the patient, to achieve a final customized, shaped field based on the specific clinical case. When treating directly with electrons, the target and flattening filter are moved out of the electron beam, and a scattering foil is used for direct electron beam treatment. When shaping the electron field, the final stage of collimation of an electron beam must be close to the skin surface. Therefore, a cone or electron applicator is commonly used to shape electron beams.
Although custom cerrobend blocks were used routinely in the past to allow custom shaping of the field for each clinical case, most modern linear accelerators incorporate a multileaf collimator (MLC) within the head of the linear accelerator. These devices effectively allow custom shaping of the field within the linear accelerator itself and can be used to further shape and refine the beam. MLCs were originally envisioned as a field-shaping device to eliminate the hazards and tedious work of creating custom cerrobend blocks to shape the field. With advances in computer technology and treatment planning, the leaves of the MLC can be moved in and out of the field rapidly during treatment, effectively resulting in the ability to modulate the intensity of portions of the beam during treatment. Effectively, portions of the beam can be blocked during a specific treatment, attenuating the intensity of a given portion of the beam for part of the treatment. This results in a radiation beam that cannot only be shaped, but intensity-modulated during treatment to improve either the homogeneity of radiation distribution or radiation conformity during treatment. This is discussed in detail later, under radiation treatment planning.
Although the majority of radiation treatment is delivered by external-beam radiation generated from linear accelerators, radiation can also be delivered from radioactive sources placed directly in or adjacent to the target tissue. The short distance between the source of radiation and the tumor or target tissue relates directly to the root of the term brachytherapy, which is derived from the Greek word brachios, meaning short distance. It has been most commonly used in the treatment of prostate and gynecologic tumors, but more recently it has been used with increasing frequency in patients treated with accelerated partial-breast irradiation, with devices such as the MammoSite8 (Hologic; Bedford, Massachusetts). A potential advantage of brachytherapy is that a high dose can be delivered directly to the target, because the absorbed dose falls off very rapidly with increasing distance from the source.
The physics of brachytherapy is fundamentally based on the phenomenon of radioactive decay. More detailed discussion of the physics of brachytherapy can be found elsewhere, but some general concepts are introduced here.3,5 Most atoms are stable and not radioactive. However, in some atoms, the ratio of the number of neutrons to protons leads to instability, and these nuclei undergo changes that lead to a more stable configuration. These reactions are accompanied by the emission of particles and electromagnetic radiation as the nucleus releases energy. These changes are referred to as radioactive decay, and the process of emission of radiation is described as radioactivity. The rate of decay of a radionuclide is directly proportional to the number of atoms of a radionuclide present in a given sample. As the number of atoms of the radionuclide decreases (as they change into the progeny nuclide), the rate of decay, or radioactivity, of the sample decreases. The rate of radioactivity change of different radionuclides is most likely different and is normally quantified with half-life, which is defined as the time required for the activity to decay to half the initial value.
In general radiation oncology practice, most commonly used radionuclides are photon emitters in the energy range of 20 keV to 1.0 MeV, although brachytherapy can be performed by using photon emitters, beta, or other particle emitters. Photons with energy lower than 20 keV and beta rays with energy lower than 500 keV can be easily attenuated in superficial layers of tissue and are of limited clinical value. In clinical practice, the radionuclide is normally made into sealed source, encapsulated with an inert metal. This metallic encapsulation may filter out low-energy photons and beta particles, leading to a possible different energy spectrum of sealed source from its corresponding pure radionuclide. It is common that for low-energy photon emitters, such as iodine-125 (125I) and palladium 103 (103Pd), different models of sealed source manufactured by different vendors possess different photon spectra because of different design of the source encapsulation. A useful physical quantity to describe the photon energy of sealed source is half-value layer in lead, which is the thickness of lead that would reduce the photon intensity to half the initial value.3
In Table 93-1, the properties of several commonly used sealed brachytherapy sources are listed. Their range of dominant photon energies is as follows: iridium 192 (192Ir) (0.06-0.88 MeV), cesium 137 (137Cs) (0.662 MeV), 125I (Model 6711-OncoSeed) (0.022-0.035 MeV), 103Pd (Model 200) (0.020-0.023 MeV), 131Cs (Model Cs-1) (0.017-0.034 MeV), gold 198 (0.071-1.09 MeV, primarily 0.412 MeV), and radium 226 (226Ra) (0.047-2.45 MeV). 125I, 103Pd, and 131Cs sources offer low-energy photons and have relatively short half-lives (in days), which make them desirable choices for permanent implants, in which the sources are placed and left inside the patient permanently. These are commonly used in the treatment of prostate cancer with permanent iodine or palladium seeds. Longer-lived sources, such as 192Ir, 137Cs, and 226Ra, are more suitable for both low dose rate (LDR) and high dose rate (HDR) temporary implants, in which the sources are placed inside or near the target volume to deliver the desired dose and then are removed from the patient after. Compared with other clinically available sources, 192Ir source has much higher specific radioactivity (radioactivity per unit mass). This unique characteristic of 192Ir makes it possible to manufacture a source with high radioactivity but small size and makes 192Ir a natural choice for the HDR brachytherapy treatment, in which the source is often transported to inside the patient via fairly narrow catheters or tubes. The HDR units are often used as sources to introduce into balloon-based brachytherapy, such as the MammoSite device, discussed in more detail in Chapter 95.
Isotope | Half-Life | Dominant Photon Energy Range (MeV) | Average Energy (MeV) | First Half-Value Layer (mm Pb) |
---|---|---|---|---|
Iridium 192 | 73.83 days | 0.06-0.88 | 0.35 | 2.2 |
Cesium 137 | 30 years | 0.662 | 0.662 | 5.6 |
Palladium 103 (Model 200) | 17.0 days | 0.020-0.023 | 0.021 | 0.008 |
Iodine 125 (6711- OncoSeed) | 59.4 days | 0.022-0.035 | 0.027 | 0.016 |
Gold 198 | 2.7 days | 0.071-1.09 | 0.412 | 2.5 |
Cesium 131 (Model Cs-1) | 9.7 days | 0.017-0.034 | 0.030 | 0.021 |
Radium 226 | 1600 years | 0.047-2.45 | 0.83 | 12.0 |
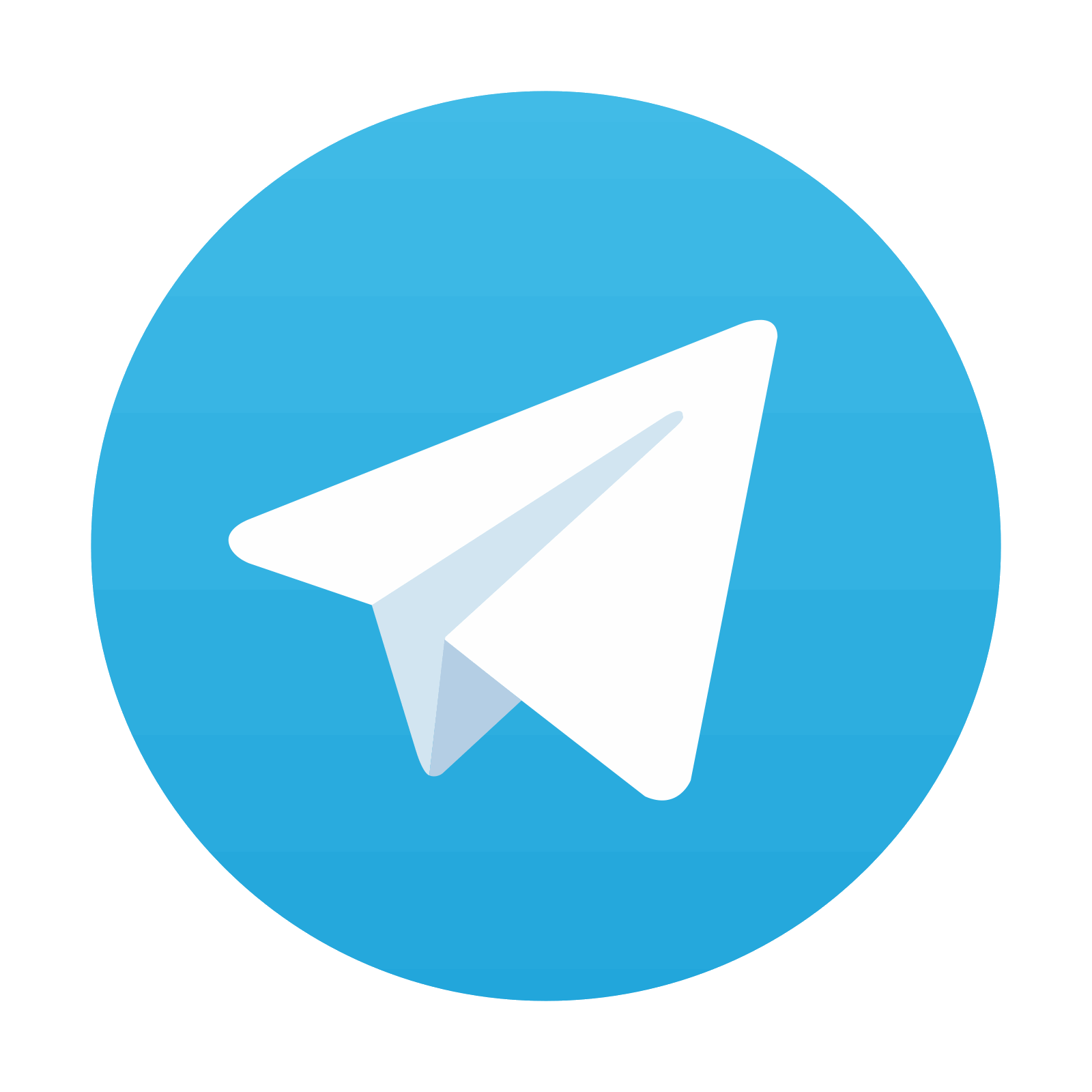
Stay updated, free articles. Join our Telegram channel
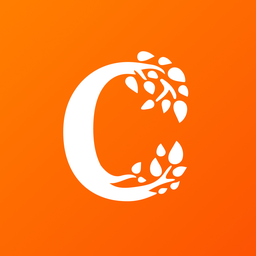
Full access? Get Clinical Tree
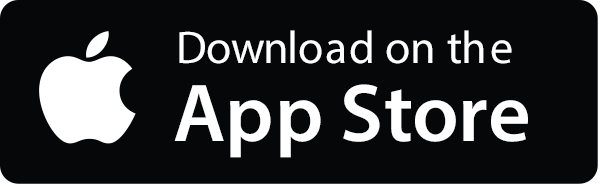
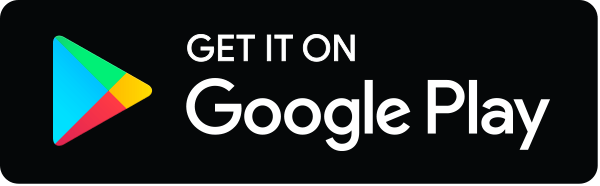
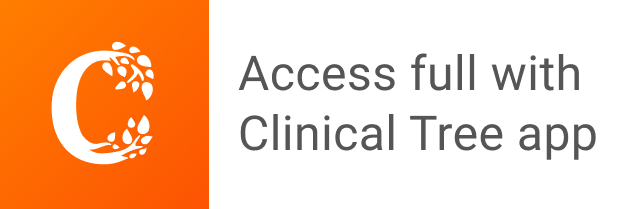