Clinical Applications of Brachytherapy: Low-Dose Rate and Pulsed-Dose Rate
Brachytherapy was the first form of conformal radiation therapy, utilizing placement of radioactive sources within or very close to a tumor and allowing high cancer to normal tissue dose ratios. From the time that Roentgen discovered radiography, the effects of ionizing radiation on the skin were noticed. Those exposed to the early cathode ray tubes, both patients and radiographers, developed radiation dermatitis and hair loss. After the isolation of radium, Henri Bequerel and Pierre Curie reported radium burns similar to those experienced by the early x-ray users. Radium was first used to treat skin lesions and superficial tumors using boxes and tubes as applicators. From there, radium in metal needles or radon gas in glass seeds were placed in direct contact with tumors using surface applicators, intracavitary, and interstitial implants.1 Source positioning within the tumor allowed high doses within the cancer with small volumes of normal tissue irradiated and sufficient dose at the margin between cancer and normal tissue to eradicate microscopic tumor foci. Early results were promising and revolutionized cancer treatment.
In the 1950s, the use of brachytherapy was widespread; however, at that time brachytherapy had a number of disadvantages. Classical radium needles were rigid with a wide outer diameter (≥1.5 mm).2 Brachytherapists had to have a high level of surgical skill to site the needles accurately, to achieve good dosimetry and quickly, and to minimize their own and others’ radiation exposure. Therefore, the emergence of teletherapy, with its advantages of decreased staff radiation exposure and radiation accessibility to more areas of the body with no dependence on surgical techniques, led to a decrease in the use of brachytherapy.
However, brachytherapy has experienced a revival due to the emergence of newer artificial high-activity isotopes, afterloading systems, and improved radiological imaging with more sophisticated dose planning techniques. Modern radiotherapy techniques have focused on dose escalation to the target and decreasing the dose to normal tissues. Brachytherapy can deliver both of these objectives in a highly conformal manner over a wide variety of disease sites. Factors such as patient convenience, for example, prostate brachytherapy requiring one or two hospital visits compared to 7 to 8 weeks with prostate external beam radiotherapy (EBRT), and high reimbursement in nonnationalized health care systems have also played a part in the resurgence of brachytherapy.
Early texts describe the important principles of brachytherapy as uniformity in cross section, depth, and opportunity (time).2 These principles remain the cornerstone of modern brachytherapy practice, although modern techniques allow better assessment of these. CT and MRI scanning has enabled enhanced imaging in brachytherapy, allowing more accurate target definition, superior determination of applicator position, and improved evaluation of the position of normal tissues. In combination with computerized dosimetry, this has allowed better determination of the dosimetric coverage of a brachytherapy implant and an estimation of the subsequent risk of normal tissue toxicity.
TABLE 23.1 THE ADVANTAGES AND DISADVANTAGES OF LOW-DOSE RATE (LDR) AND PULSED-DOSE RATE (PDR) BRACHYTHERAPY
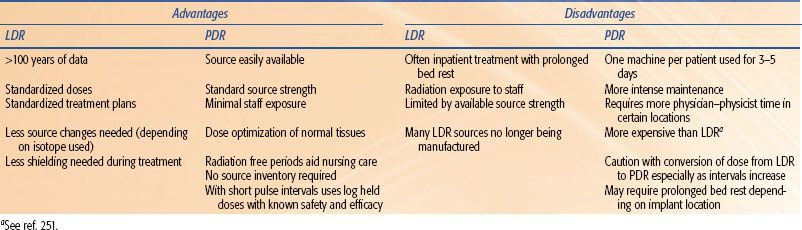
DOSE RATE DEFINITIONS
Three categories of brachytherapy were defined in Report 38 of the International Commission on Radiation Units and Measurements3:
• Low-dose rate (LDR): a range of 0.4 to 2 Gy per hour. In clinical practice the usual range is 0.4 to 1 Gy per hour.
• Medium-dose rate (MDR): a range of 2 to 12 Gy per hour.
• High-dose rate (HDR): over 12 Gy per hour.
Permanent seed implants deliver a high total dose at a very low-dose rate (vLDR), usually at <0.4 Gy per hour. Pulsed-dose rate (PDR) brachytherapy was developed in an effort to simulate the radiobiological advantages and dosimetric properties of LDR, but with the advantages of computer optimization of dose, a stepping source, and remote afterloading usually achieved by HDR. A source with activity in the realm of one-tenth of HDR activity is used. Generally, the same total dose and total time as LDR are prescribed but the radiation is administered in a large number of small fractions, usually a pulse every 1 to 4 hours. There are indications that the toxicity of PDR can be the same as for LDR or HDR in clinical practice.4,5 It also has the advantage of a single afterloaded source compared to an inventory of LDR sources of different strengths. This will become increasingly important as manufacture of traditional LDR sources ceases and disposal costs of existing LDR sources rise. Table 23.1 demonstrates the advantages and disadvantages of LDR and PDR brachytherapy.
TABLE 23.2 CHARACTERISTICS OF RADIOISOTOPES THAT CAN BE USED FOR BRACHYTHERAPY
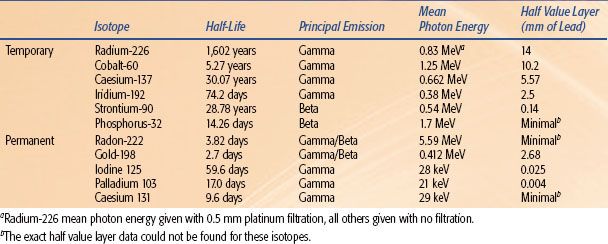
CLINICAL USES OF BRACHYTHERAPY ISOTOPES
Brachytherapy uses radioactive isotopes to deliver therapeutic doses of radiation (Table 23.2). The ideal radioisotope for brachytherapy should have a relatively short half-life in order to deliver the radiation in as short a time as possible and a high specific activity so that the source is small and therefore more versatile to implant. The emissions produced should have an adequate penetration to deliver the dose to the depth desired with rapid falloff to prevent damage to the surrounding normal tissue. Particular source characteristics such as half-life or specific activity can be tailored to the tumor and the surrounding organs at risk (OAR).
Temporary Implants
The first temporary isotope used was radium-226 (226Ra), which was generally implanted in metal tubes with 1-mm platinum filtration. These were bulky, inflexible needles that were difficult to implant and resulted in a high radiation exposure to surrounding staff. Radium-226 also has an extremely long half-life for future disposal concerns. Cobalt-60 (60Co) was one of the first artificial radionuclides used, available in interstitial needles and wires, but its usefulness for brachytherapy was limited by its low activity and short half-life, although it is used in afterloaders in the developing world with the advantage of decreased pressure on quality assurance and cost.6,7 Cesium-137 (137Cs) sealed into a ceramic or glass pellets was in use from the 1960s. From the 1970s, 137Cs was the predominant isotope used worldwide for intracavitary gynecologic implants. However, production of this isotope ceased in 2002 and most departments wishing to continue using LDR-style dose and characteristics have switched to isotopes more suitable for remote afterloading, often using PDR techniques. Strontium-90 (90Sr) is a pure beta-emitter; therefore, it is suitable for very superficial applications and commonly used for coronary brachytherapy and ophthalmic problems such as pterygium. It has a half-life of 28.1 years, so many departments are still using applicators purchased in the 1980s. Ruthenium-106 (106Ru) is also a beta-emitter but with a much shorter half-life (1 year), lessening disposal concerns and making treatment delivery faster.
Iridium-192 (192Ir) has been used since the 1960s in afterloading systems—both manual and remote. Its high specific activity makes it extremely suitable for remote afterloading due to the increased flexibility available with a smaller source. The small source size has enabled implants in areas where cesium sources would be too large, and the afterloading machine allows implants into much longer applicators, such as the esophagus and bronchus, than was easily attainable with manually afterloaded 192Ir wires.
Permanent Implants
Radon-222 gas (222Rn) sealed into glass seeds was used in the first permanent implants. This had a very short half-life and required harvesting from radium sources. Gold-198 (198Au) was the first artificial isotope to be used in permanent implants. It matched the classical LDR dose rate of 0.3 to 1 Gy per hour but with such a short half-life did not overcome the logistical problems of 222Rn (i.e., time and radiation protection). The newer artificial isotopes—iodine-125 (125I), palladium-103 (103 Pd), and cesium-131 (131Cs)—are all used in modern permanent vLDR implants. The isotope used can be chosen according to its individual characteristics, which may be desirable in different implants (e.g., slower dose delivery may decrease normal tissue toxicity or a tumor with a higher alpha/beta ratio may theoretically have more cell kill using an isotope with a shorter half-life). All three isotopes are almost completely shielded with 0.2 cm of lead foil, making radiation protection easier.
Clinical Suitability for Brachytherapy
Cancers with clinically and radiologically well-defined margins with a low risk of regional and metastatic spread are the most suitable for brachytherapy as a single modality. However, brachytherapy is becoming increasingly important when integrated with EBRT to give a highly localized boost. EBRT is used to sterilize a larger area of possible microscopic or nodal spread with brachytherapy used for areas of gross macroscopic or microscopic residual disease. This ensures that high doses are achieved within tumors while normal tissues are not taken beyond recognized organ tolerance levels. It also has the potential for highly conformal localized dose escalation to areas at high risk of tumor recurrence.
In EBRT the dosimetric goal is a homogeneous dose distribution with doses ranging from 95% to 107% of the prescribed dose.8 In contrast, brachytherapy has an extremely heterogeneous dose distribution with isolated areas receiving in excess of 200% of the dose. The steep gradients are a consequence of the proximity of the clinical target volume (CTV) to the sources and decreasing dose with distance secondary to the inverse square law. The normal tissues benefit from the inherent heterogeneity of the brachytherapy dose. As the dose falls off with distance, the normal tissue experiences not only a reduced dose but also a reduced dose rate, which results in enhanced cell sparing. The sharp dose fall off with brachytherapy contrasts with the more gradual dose fall off seen with EBRT.
Brachytherapy can be given over a short duration (e.g., using LDR a radical head and neck treatment course can be administered over 5 to 6 days compared to 5 to 7 weeks for a conventional EBRT head and neck regime). Brachytherapy can also be used as a method of retreatment when a patient has received irradiation to normal tissue tolerance using EBRT. Using the principles described above of sharp dose falloff and highly conformal dosing, a clinically useful radiation dose can be administered while minimizing the risk of increased late toxicity resulting from reirradiation.
Brachytherapy can be used anywhere in the body that can be accessed for direct source placement. For many years, surface applicators and intracavitary gynecologic brachytherapy were the predominant modes of brachytherapy. However, with the advent of modern surgical techniques and interventional radiology, many more areas of the body are now accessible to the brachytherapist. Brachytherapy applicators can be placed within tubular organs in the body, either under direct vision (e.g., cervix or vagina) or with the use of radiological or endoscopic guidance (e.g., bile ducts or esophagus).
Interstitial implants can be placed with or without image guidance (e.g., prostate implants using rectal ultrasound guidance and a template or a freehand extremity sarcoma implant). Interstitial implants can take the form of free seeds, seeds within linked strands, or catheters that will be loaded with the radioactive source. Of course, surface applicator techniques are still used, with the added benefits of modern imaging and dosimetry techniques to ensure target coverage.
LDR sources can be placed directly into the body; however, with higher activity sources and large implants, this can result in a high radiation exposure to the brachytherapist and operating room staff. To overcome this problem, afterloading techniques were developed. Initial techniques required applicator placement in the operating room and manual afterloading of sources in a shielded room at a later time. This has mainly been supplanted by remote afterloading techniques, where the patient has the applicators placed while in the operating room and is later connected to a remote afterloading machine in a shielded room. The sources will only enter the patient when all personnel are at a safe distance from the patient; this can be used for LDR and is essential for PDR.
When using LDR or PDR, it is important that the patient can be safely left in a room without direct supervision, because during the period of source excursion radiation dose to staff must be kept to a minimum. During an LDR implant, careful monitoring of the sources must be maintained to detect source displacement. During a PDR implant, the patient should be monitored for applicator displacement between pulses; this may be under direct vision or using radiological confirmation. Devices aiding local protection of OAR may be used (e.g., leaded gum shield during lip brachytherapy).
Target Definition
Many of the same definitions for delineation of treatment volumes in EBRT are used for brachytherapy.8,9 There may be delineation of high-risk and low-risk areas if discrete areas of dose escalation are required within the CTV. The high-risk area has the highest risk of local recurrence, usually due to the presence of gross residual disease. An intermediate area may be defined that corresponds to areas where there was macroscopic tumor at the time of diagnosis that has regressed by the time of brachytherapy implant. The low-risk area includes potential microscopic spread (i.e., the traditionally defined CTV). In EBRT the planning target volume (PTV) traditionally consists of the CTV plus a margin that will allow for physiological movement and setup uncertainty. Generally, in brachytherapy these variations are minimized and therefore the PTV is usually the same as the CTV.
In a brachytherapy implant there will be high-dose regions around each source. Generally, the volume of the region receiving over 150% (V150) of dose is reported.3 Low-dose regions are those within the CTV receiving <90% of the prescribed dose. Due to the heterogeneity of dose in brachytherapy, the average dose within the prescribed volume is usually far higher than the prescribed dose at the reference isodose point on the periphery of the implant. This is tolerated due to the volume–effect relationship: very small normal tissue volumes (e.g., 1 to 2 cm3) can tolerate very high radiation doses that larger volumes would not tolerate. The dose to organs at risk should be reported, either as a total dose or a ratio of volume. Methods such as the minimum dose received by the most irradiated 2 cm3 (D2cc) of tissue or the volume receiving over 90% of the dose (V90) or dose received by 90% of the CTV (D90) are assessed.
RADIOBIOLOGIC CONSIDERATIONS
Radiobiological principles are important in the daily clinical use of all forms of brachytherapy. Brachytherapy was initially developed empirically with doses being determined by clinical effect. In the modern era, radiobiological modeling is used to predict the biological effect of varying dose prescriptions. The importance of radiobiology and its use within brachytherapy was emphasized by the move from LDR treatment to PDR and fractionated HDR treatment. Of course, it must be remembered that applicator and source placement remains the single most important factor in brachytherapy such that, in an implant with poor geometry, changing radiobiologic parameters, such as fractionation or dose rate, will not improve the outcome.
Factors contributing to the response of tissues to radiotherapy have been labeled the “4 Rs of radiotherapy”10: repair, reassortment, repopulation, and reoxygenation. The way in which these radiobiological characteristics relate clinically to the use of LDR and PDR either alone or in comparison to HDR follows.
Repair
Sublethally damaged cells are capable of repair if they are allowed sufficient time and if the cell contains all of the necessary DNA repair proteins and enzymes. If sublethally damaged cells are exposed to further irradiation before repair occurs, the damage may become lethal. Late-reacting normal tissues seem more capable of repair than tumor cells so, at a given therapeutic dose, tumor is preferentially killed over normal tissue. This is probably due to a loss of repair fidelity in addition to a lack of relevant repair proteins and enzymes in the tumor cell. The lower the dose rate of radiation that a cell is exposed to, the more likely it is that repair of normal tissues will occur within that cell before a second injury occurs.
The time course of LDR or PDR treatment over several days allows time for sublethal damage repair in normal tissues. In contrast, the short treatment time of HDR treatment prohibits this repair during the actual irradiation. Using PDR, generally the same total dose and total time as LDR are prescribed. If PDR is given at a pulse width of 10 minutes and a 1-hour pulse interval, the dose is equivalent to LDR 0.6 Gy per hour.11,12 If the dose per pulse is small (≤0.5 Gy) and the normal tissue repair half-time is over 30 minutes, the differential effect to LDR is <10%. If the dose per pulse is over 2 Gy or the tissue repair half-time is under half an hour, this is not the case and the PDR effect becomes biologically closer to a highly fractionated HDR treatment, especially in close proximity to the source.13 In this situation, a lower total PDR dose than LDR can be given in the same overall time to achieve equivalent clinical effect. Sometimes PDR is prescribed using an “extended office hours” schedule (i.e., 8 a.m. to 8 p.m.). This is done commonly to overcome regulatory issues where a physicist and physician must be present for every source excursion. One general rule when transferring PDR to LDR dose is not to exceed the overall dose rate that would be delivered by LDR in a day (e.g., at a dose rate of 0.5 Gy per hour, LDR delivers a total dose of 12 Gy per 24 hours), thus by using a daytime-only PDR schedule, providing a similar biological effect and normal tissue complication probability, the overall treatment time will commonly be slightly extended.14
Dose rate is a key factor in determining the biological effects of brachytherapy. In general, the effects of radiotherapy increase as the dose rate increases, predominantly due to a decrease in repair. It has been suggested that the total dose of LDR prescribed should be corrected for overall time of the implant,15 although some investigators have found no difference in local control with the same total dose administered using a dose rate ranging from 0.005 to 0.0167 Gy per minute.16 A randomized study in cervix carcinoma showed no difference in overall survival or local control for a dose rate of 0.4 versus 0.8 Gy per hour.17,18 However, there was a significant increase in late complications in the higher dose rate group. A similar trend has also been seen in LDR brachytherapy for breast cancer and head and neck carcinoma.19,20 Therefore, it is recommended that the LDR (and PDR equivalence) should be in the range of 0.3 to 1 Gy per hour due to the effects on late complications rather than local control. If the dose rate exceeds 1 Gy per hour, a reduction in the total dose can be calculated using the biologically equivalent dose (BED) equation (see Chapter 22).
Reassortment
In normal cells, proliferation occurs in a sequence of events termed the cell cycle.21 There is a theoretical advantage of an improved effect on reassortment using LDR treatment because, during the overall treatment time, cells will pass out of the relatively radio-resistant cell cycle phases of late S and early G2 into the more radio-sensitive phases of late G2 and M. This has been shown in vitro,22 but in vivo the effect of reassortment has not been shown to give a true advantage, possibly due to a disruption of the mechanisms of the cell cycle in cancer cells.21
Repopulation
In squamous cell carcinoma, studies have shown improved tumor control and increased survival when a radiotherapy course is given in the shortest overall time.23–26 This may be because shorter treatment times allow less time for tumor cell repopulation or for accelerated repopulation to occur. The continuous administration of LDR and PDR probably prevents repopulation during treatment.
Reoxygenation
The response of cells to radiation is strongly dependent on oxygen. Radiation results in free-radical formation within a tumor, and oxygen reacts with these free radicals to make DNA damage irreparable. The effect of hypoxia on tumor control has been well documented with decreased survival in certain patients with a low initial hemoglobin levels.27–29 There are two hypoxic cell populations within tumors: chronic and transient. As a tumor outgrows its blood supply, a proportion of cells will become necrotic. Viable cells near this necrotic zone will be chronically hypoxic. Transient hypoxia may occur over minutes to hours as small vessels within the tumor open and close or small tumor emboli intermittently block blood vessels. When using LDR and PDR, transient hypoxia may correct during the treatment time,30 which is not possible during the short duration of HDR brachytherapy. If the brachytherapy is fractionated, tumor shrinkage and reoxygenation of areas of chronic hypoxia may occur between insertions.
MEASUREMENT OF BRACHYTHERAPY DOSE
Dosimetry systems aim to give the brachytherapist a set of guidelines to follow, which result in a prescribed dose being delivered to a patient in a predictable fashion. Initial brachytherapy dosimetry systems were developed for LDR implants using an empirical approach toward source placement and dose calculation. More modern techniques use computer programs to generate isodose distributions, which can then be analyzed in two dimensions, three dimensions, and volumetrically. In order to achieve the best ratio of cancer to normal tissue dose, the selection of an appropriate prescription point is essential. Applicator positioning is of primary importance, as differential loading cannot compensate for a poorly sited implant.
The Manchester dosimetry system was one of the first published dosimetry systems, with its origin dating from the 1930s.31 Guidelines for the use of LDR wires and tubes are set out for surface molds, interstitial planar implants, and volume implants. However, it required a range of isotope activities to be available, and at that time the available radium sources in the United States had a more limited range of activity. Therefore, the Quimby system modified the Manchester system to allow uniform source strengths to be used throughout the implant.32 The Paris dosimetry system developed guidelines in the 1970 s for modern LDR brachytherapy sources such as flexible 192Ir wires.33 It is difficult to achieve an implant that conforms exactly to the Paris rules without the use of a template (see Chapter 24).
Where LDR uses fixed source positions and strengths to calculate the dose at the prescription point, afterloading machines and computerized dosimetry systems allow optimization of source dwell times to customize dose delivery to the patient’s individual anatomy and tumor volume. PDR takes advantage of this dose optimization in a way that LDR cannot. Optimization results in nonuniform source loadings that give greater dose uniformity and CTV coverage that is often similar to an idealized Manchester dosimetry system implant.34 Prior to the introduction of computerized dosimetry, the dosimetry of an implant was based on the intended source position rather than the actual source position. This could be highly dependent on the expertise of the individual brachytherapist. Improved radiological imaging has allowed more accurate definition of the CTV and associated normal tissues, giving dose specification according to patient anatomy rather than applicator position. This is more likely to correlate with patient outcome than previous dosimetry systems. This may result in not only better tumor control but also decreased risk of late complications.
Now that computerized dosimetry is available, brachytherapists may be tempted to abandon knowledge of the previous dosimetric systems and place sources as they see fit, using the computer to calculate dose distribution. This approach risks overdosing part of the volume. There is a steep dose gradient around each wire and wide-spaced sources may form large high-dose regions and increase the risk of necrosis. In the same way, an isodose that covers the volume but is at a large distance from the sources may also cause necrosis in the vicinity of the sources. The maximum source separations and treatment thickness in the Paris system are useful rules to remember to decrease this risk. The principle of extending the sources beyond the target or crossing at the ends should also be remembered to overcome the inherent dose fall off at the end of the source. This can be achieved using optimization with PDR brachytherapy. The rules from individual systems should not be mixed, even if computerized dosimetry is used for dose calculation.
Brachytherapy dose delivery traditionally was thought not to be affected by alterations in the treatment position. Although this may be true for dose delivery to the target volume, the position of organs at risk in relation to the brachytherapy implant may be altered, thereby increasing the dose delivered (e.g., a patient undergoes simulation lying flat on a computed tomography [CT] scanner with a breast implant that falls laterally with gravity). On sitting up, this applicator may then fall more medially, potentially changing cardiac, lung, and skin doses.
CLINICAL SITES
There are areas where LDR techniques continue to predominate (e.g., vLDR prostate brachytherapy), areas where HDR techniques predominate (e.g., esophageal brachytherapy), and areas where the use of HDR and LDR (now moving to PDR) are generally equal (e.g., cervix cancer). The sections below present an overview of clinical uses for LDR and PDR with an emphasis on the brachytherapy dose rate rather than the treatment technique. Individual indications will be described in the site specific chapters.
FIGURE 23.1. Administration of a spinal anesthetic prior to a pelvic brachytherapy procedure.
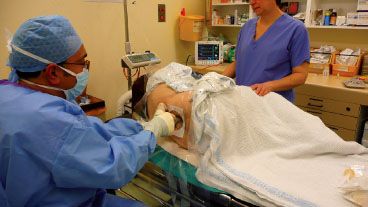
FIGURE 23.2. Anterior-posterior simulator radiograph demonstrating low-dose rate tandem and ovoid insertion with dummy sources in situ.
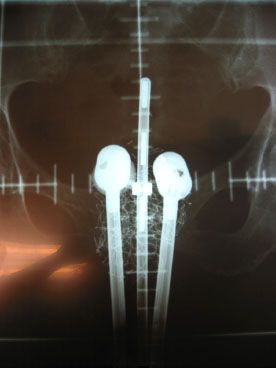
Cervix Cancer
The use of LDR for cervix cancer treatment was first described with intracavitary implants in 1903 and with interstitial implants in 1913.35 It has withstood technological innovations to remain an essential part of curative cervix cancer treatment.36,37 for all but the very earliest stages of the disease. The use of LDR has many years of safety and efficacy data, and physicians can be confident in their choice of brachytherapy dose. These doses have been shown to be biologically equivalent using PDR as long as the rules governing pulse length and pulse interval are carefully followed.14,38 In contrast, a wide variety of HDR dose and fractionation schemes are used,39 with shorter follow-up data for efficacy and toxicity. Randomized clinical trials have shown the equivalence of HDR brachytherapy and LDR brachytherapy,40 and some studies suggest a lower morbidity with HDR brachytherapy, which could possibly be due to the ability to optimize the dose away from normal tissues.41,42
Several trials demonstrated the superiority for chemoradiotherapy over radiotherapy alone in cervix cancer treatment.43 All of these trials used LDR brachytherapy, and there are no prospective randomized safety data on the use of HDR brachytherapy and chemoradiotherapy. PDR brachytherapy utilizes the dose and scheduling of LDR and thus should have a similar efficacy profile while allowing the dose optimization capability of HDR, which may improve normal tissue toxicity. Studies have shown this to be the case in clinical practice.44–46
With the use of CT- and magnetic resonance imaging–based target volume and organ at risk definition, dose reporting in cervix cancer brachytherapy has changed from being point based to volume based.47,48 This means that tumor coverage is improved and the doses to normal tissues can be decreased. This method relies on the use of dose optimization and thus moves away from LDR and toward PDR as an equivalent treatment modality. The longer time taken to deliver 1 overall fraction of PDR than HDR is important when optimizing dose away from normal tissues, and it is important to recognize that the dose delivered to normal tissues over the whole treatment time may differ from that predicted, up to 33% more.49 A comprehensive description of this volume-based dosing is given in Chapter 25 (see Table 25.3). American Brachytherapy Society guidelines for the use of LDR and PDR in cervix cancer have recently been updated,50 incorporating the volume-based dosimetry and updated quality control recommendations.
The applicator is generally placed under operative conditions. Review of preoperative imaging is essential to determine which applicator is most appropriate. The patient may require a general anesthetic, but spinal anesthesia (Fig. 23.1) has been shown to provide excellent analgesia, which can be maintained throughout the length of the implant using a spinal catheter.51 Spinal anesthesia does not affect tumor oxygenation during an HDR implant,52 thus it is unlikely to during the whole duration of an LDR or PDR implant. With the patient in the lithotomy position, examination under anesthesia confirms preoperative imaging findings. The cervix is then dilated and a uterine tandem is placed, preferably using ultrasound guidance.53 A variety of commercial applicators are available for intracavitary brachytherapy (Fig. 23.2), with the tandem and ring applicator becoming increasingly popular for tumors <5 cm. For tumors over this size, the addition of interstitial needles into specialized applicators (e.g., the Vienna applicator)54 is becoming more common because it has brought the ability to deliver interstitial brachytherapy to a wider group. Interstitial template applicators such as the Syed-Neblett55 or the multiple site perineal applicator56 applicator can be used to treat disease with lateral extension, although it is important to maintain a degree of central dose heterogeneity (in contrast to the heterogeneity preferred in interstitial implants in other areas of the body) to maintain the central cervix doses needed for cure (Fig. 23.3).57 Customized vaginal molds can be used and are particularly prevalent in France,58 offering truly customized brachytherapy dosing.
An LDR applicator is typically afterloaded once the patient returns to the shielded isolated patient room. The patient will undergo CT scanning and using PDR dose-optimized planning before dose delivery. When the patient is receiving the dose, she will be nursed lying flat with prophylaxis against venous thromboembolism. Typically, agents are given to slow bowel motility, and it may be preferable to follow a low-irritant fiber diet before and during the implant. A urinary catheter is maintained throughout treatment. Prophylactic antibiotics are not routinely required. Pain and discomfort may be managed by epidural or intravenous patient controlled analgesia. Care is taken to minimize exposure to the medical staff caring for the patient through the use of mobile shielding placed around the bed and by the training of the staff as to the time and distance radiation safety rules. Pregnant staff members are not allowed in the area so as to minimize risk of dose to fetus.
FIGURE 23.3. A: Low-dose rate (LDR) template interstitial implant for a vaginal vault recurrence of cervical carcinoma. B: Axial computed tomography slice demonstrating the target volume and dosimetry of an LDR vaginal vault template interstitial implant.
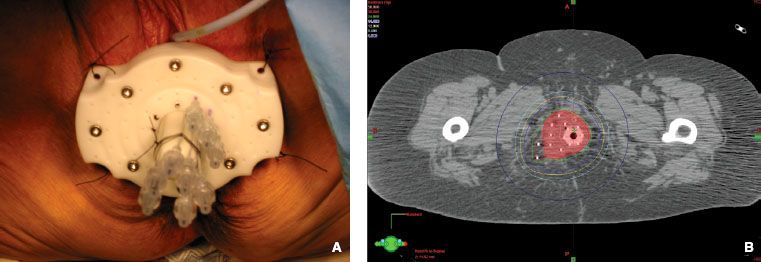
FIGURE 23.4. A: Double tandem Martinez applicator in place with three Heyman’s capsules placed within the uterus. B: Anterior-posterior simulator radiograph demonstrating the brachytherapy insertion pictured in Figure 23.5, with isodose lines overlaid. (Courtesy of Dr Akila Viswanathan.)
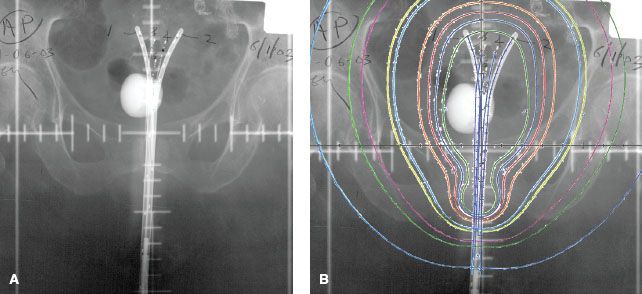
Endometrium
Endometrial carcinoma is the most common female pelvic malignancy in Western countries.59,60 Brachytherapy for the management of endometrial carcinoma was first described by Heyman61 in 1935, prior to the routine use of hysterectomy for uterine cancer. The majority of endometrial cancer patients now present at an early stage, and the use of radiation alone has evolved. The primary treatment for endometrial cancer is surgery (total abdominal hysterectomy and bilateral salpingo-oophorectomy) with or without adjuvant radiotherapy and chemotherapy based on the histology of the tumor and stage of presentation. As endometrial carcinoma is linked to obesity and hypertension, some patients have medical comorbidities that preclude surgery; for those, radiation therapy may be the definitive treatment of choice. In this group, treatment is delivered via intracavitary uterine brachytherapy, with or without EBRT.62–64
For definitive treatment, packing the uterus with capsules allows excellent coverage of the entire endometrial cavity that can be adapted to every patient’s individual anatomy.65,66 Standardized applicators may provide more straightforward and reproducible dosimetry. Double channel applicators such as the Rotte applicator67 can be used with CT planning, and computerized optimization with HDR delivers a very similar dose to the serosa,68 which could be replicated with PDR (Fig. 23.4). Various whole uterus prescription points have been described69,70; however, CT planning allows contouring of the outer contour of the uterus for volume-based dose prescription. A typical dose is 70to 80 Gy prescribed to the outer contour of the uterus alone or 35 to 50 Gy in combination with 30 to 45 Gy EBRT.71
Vaginal vault brachytherapy can be used in the adjuvant treatment of endometrial cancer and vaginal vault recurrence of endometrial carcinoma and also in other gynecologic malignancies such postoperative early-stage cervix cancer or early-stage vaginal cancer.60,72–74 The target for adjuvant vaginal vault brachytherapy is the vaginal mucosa and the operative scar. Ninety percent of recurrences occur at the vaginal vault and 10% in the distal vagina75; therefore, in the majority of cases the upper third to half of the vagina is treated. This decreases the morbidity associated with treating the whole vagina, such as vaginal dryness or shortening.71 Brachytherapy can be prescribed at the cylinder surface or at 5 mm into tissue, a depth that approximates the vaginal lymphatics.
Vaginal vault brachytherapy can be administered using a variety of different applicators. The commonly used single channel cylinder comes in a variety of widths chosen according to patient anatomy and comfort (Fig. 23.5). This may be a less favorable choice when using LDR as the lack of optimization leads to effects from source anisotropy. The vaginal apex is located along the longitudinal axis of the source; therefore, the vaginal apex is exposed to the greatest effects of source anisotropy. It is important that planning systems contain modifications for source anisotropy. Li et al.76 used Monte Carlo simulations to show that not accounting for anisotropy can result in underdosing by as much as 30% at the vaginal apex. The use of optimization with PDR to points off the cylinder apex can overcome this problem and may lead to decreased late toxicity.77 Of course it must be considered that the doses we commonly prescribe for brachytherapy to the vaginal apex are those formulated using years of empirical dosing, and the received dose at the vaginal apex may have actually been much higher than the prescribed dose. If we now use the same prescribed dose but lower the actual dose received by the vaginal mucosa using methods such as optimization to the cylinder apex or anisotropy correction, it is possible that it could be detrimental to tumor control. Vaginal colpostats are an alternative applicator that delivers a dose more localized to the vaginal vault and less dose to the midlower vagina.
The use of pelvic radiotherapy in selected stage Ia and Ib patients (intermediate risk) has been examined in randomized trials75,78–80 and a Cochrane meta-analysis.81 Selected patients may have vaginal cylinder brachytherapy alone as adjuvant radiotherapy, which gives similar regional control rates to pelvic EBRT with significantly less toxicity.82,83 Adjuvant primary vaginal vault irradiation has been shown to decrease the incidence of vaginal apex recurrence in endometrial cancer from 12% to 15% to as low as 0% in selected patients, although it has no impact on overall survival.72,82 The dose for LDR vaginal vault alone is 50 Gy prescribed at 5 mm over 4 to 5 days or 0.5 Gy per hour and approximately 15 Gy when combined with 45 Gy EBRT.71 The radiation dose received by the pelvic organs varies according to physiological variations (e.g., the bladder dose may vary according to the extent of bladder filling, which may also affect the amount of small bowel in the field).84,85
FIGURE 23.5. Lateral image of a digitally reconstructed radiograph demonstrating a vaginal cylinder in situ and its relationship to surrounding normal tissues.
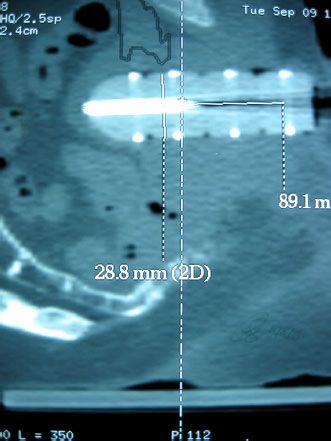
TABLE 23.3 INCLUSION CRITERIA FOR PROSTATE BRACHYTHERAPY
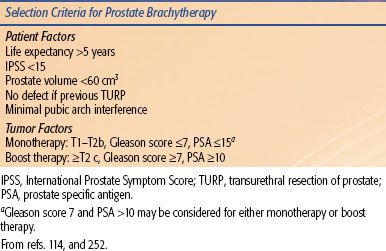
Prostate
Prostate brachytherapy has become part of the treatment paradigm in prostate cancer for all stages of localized disease (Table 23.3). It can be used as monotherapy or in combination with EBRT or hormone therapy for higher risk disease. The most common application for monotherapy is with LDR permanent seeds. When used as a boost, both LDR and HDR techniques are used as well as recent publications of PDR boost. Prostate brachytherapy is also being investigated as salvage therapy after external beam radiation86 and is currently under investigation through the Radiation Therapy Oncology Group (RTOG) study RTOG-0526.
A transrectal ultrasound-guided transperineal technique is the most popular technique for implanting the prostate. Other modalities incorporating MRI87 and MRI spectroscopy imaging have also been proposed.88 The techniques for implanting 125I and 103Pd have been well described.89–91 The patient criteria for selection include ability to undergo a general, spinal, or less commonly local anesthetic,92 prostate volume <60 cm3, favorable anatomy (minimal or no pubic arch interference, median lobe), and minimal obstructive uropathy. Significant transurethral resection of prostate defect is also a relative contraindication to a seed implant.93 Tumor criteria depend on whether monotherapy or boost therapy is planned. Typically monotherapy is reserved for patients with so-called favorable risk disease (e.g., low-intermediate risk disease: T1 to T2b disease, Gleason score <7, prostate specific antigen <15 ng/mL) and boost therapy for patients with intermediate- and high-risk disease. The role of EBRT and hormone therapy in combination with LDR brachytherapy is unclear and under investigation. Adjuvant androgen deprivation therapy (ADT) is used for prostatic cytoreduction in patients who do not have ideal prostatic geometry.94 Cytoreduction may be achieved with use of luteinizing hormone-releasing hormone agonists and antiandrogens or antiandrogen in combination with 5α-reductase inhibitors.95 ADT is also considered in patients thought to be at higher risk of prostate cancer recurrence, however, the benefit of ADT in this setting is not well defined.96–99
In transperineal techniques, treatment planning can be done either as a preplan or intraoperatively. In the preplan technique, a volume study is acquired prior to the procedure and the prostate volume and pubic arch interference are assessed. Axial images (5 mm) of the prostate from the base to the apex are taken and then used for brachytherapy planning (Fig. 23.6). Sagittal imaging can be used to help identify the base and apex. Reproducibility of the prostate position on the day of insertion must be ensured in this technique. Intraoperative techniques are defined as intraoperative preplanning, interactive planning, and dynamic dose calculation. Intraoperative preplanning avoids two separate ultrasound studies and involves the creation of a plan at the time of the implant procedure. It, like preplanned techniques, does not account for deviations of needle position or prostate geometry changes from the preplan.100 The time required for the implant tends to be more prolonged using this technique compared to the preoperative technique. Interactive planning allows for refinement of the treatment plan based on needle-position feedback and estimation of seed placement. Modifications can then be made to needle position or additional seeds can be added to optimize the plan. Dynamic dose calculation allows constant updating of the dose distribution based on the actual seed positions and takes into account changes in the prostate volume.101,102
Current permanent LDR techniques involve implanting 125I or 103Pd seeds. Cesium-131 is also approved for prostate brachytherapy and is used at some institutions. These isotopes have differing energies and half-lives and therefore initial dose rates (see Table 23.2). It had been postulated that 103Pd compared with 125I was more effective at treating dedifferentiated tumors as the dose rate is higher; however, studies evaluating clinical outcomes for patients with prostate cancer have shown no difference.103–105 Studies evaluating the toxicity to OAR, including rectum, bladder, and sexual function, have shown no difference in acute or long-term toxicity or difference in sexual function.106–108 There is a difference in the time for the International Prostate Symptom Score (IPSS) to return to the preimplant baseline, with 103Pd returning to baseline faster.109 Cesium-131 has not been studied as extensively. Commercially, 125I and 103Pd seeds are available as either loose or stranded seeds. Dosimetrically, the two are similar. The incidence of seed migration is decreased with stranded seeds as compared with that of loose seeds, with the greatest difference being in migration to the lung and perineum.110
The prescription dose for permanent LDR brachytherapy is dependent on the isotope and the indication for the implant, either monotherapy or as a boost. Table 23.4 describes the recommended doses for 125I, 103Pd, and 131Cs when used as monotherapy or when combined with 40 to 50 Gy EBRT.94,111,112 The prescription dose covers the entire prostate (CTV) and typically includes a margin. The dose for 131Cs is still investigational.
In the preoperative technique, the dose to the target volume as well as the organs at risk are evaluated. Different loading techniques are used to achieve these goals based on the needle placement, spacing of seeds, and seed energy.93 A peripheral loading technique is common to avoid excess dose to the urethra with 125I, and a modified uniform loading is used with 103Pd.113 The source placement with 131Cs has different guidelines from 125I and 103Pd, with needles placed farther from the urethra and rectum.111 Good preimplantation dosimetry and toxicity levels correlate with a V100 >95% of CTV, D90 >100%, and V150 ≤50% of CTV, rectum D2cc less than reference prescription dose, Dmax 150% of reference prescription dose, prostatic urethra D10 <150%, and D30 <130%.102 Dose limits to structures such as the penile bulb and neurovascular bundles are under investigation.
Postimplant dose reporting in a structured quality assurance program is highly recommended and should include dose parameters to both the target as well as the OAR.112,114 Timing of the postimplant imaging varies at different centers but ideally should relate to the time at which edema is at a minimum. Evaluation at either day 0 or day 30 is most common. Seed localization is crucial as is the contouring of the prostate and normal structures. CT-MRI fusion if possible allows for identification of both the seeds and accurate definition of the prostate, particularly at the apex and base, which tend to be difficult to delineate on CT (Fig. 23.7). Primary parameters to report and evaluate include the intended dose—D90, D100, V100, V150—to the prostate gland and rectal volume and dose and urethral doses. For patients with unacceptably cold implants, consideration for reimplantation should be given. There are no parameters at present for penile bulb and neurovascular bundles.
Studies have shown that prostate brachytherapy is associated with a “learning curve” effect.115–117 As with any brachytherapy technique, outcomes and toxicities are dependent on where the radiation has been delivered. With permanent seed brachytherapy, there can be no dose optimization once the seeds are placed. A quality assurance process that enables closing the feedback loop for optimizing subsequent implants is therefore crucial. Peer review, assessments of dosimetry, toxicity and outcomes as well continuing education should be part of any prostate brachytherapy program.118–122
FIGURE 23.6. Prostate volume study with prostate and clinical target volume defined (A), preplan with peripheral loading technique (B).
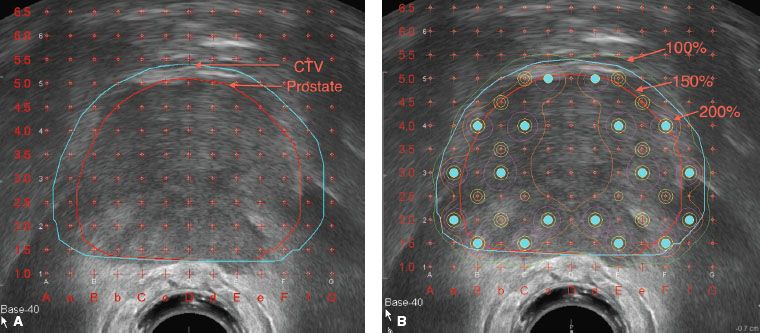
TABLE 23.4 PRESCRIPTION DOSE FOR PERMANENT LOW-DOSE RATE BRACHYTHERAPY WHEN USED AS MONOTHERAPY OR AS A BOOST COMBINED WITH 40–50 GY EXTERNAL BEAM RADIATION THERAPY

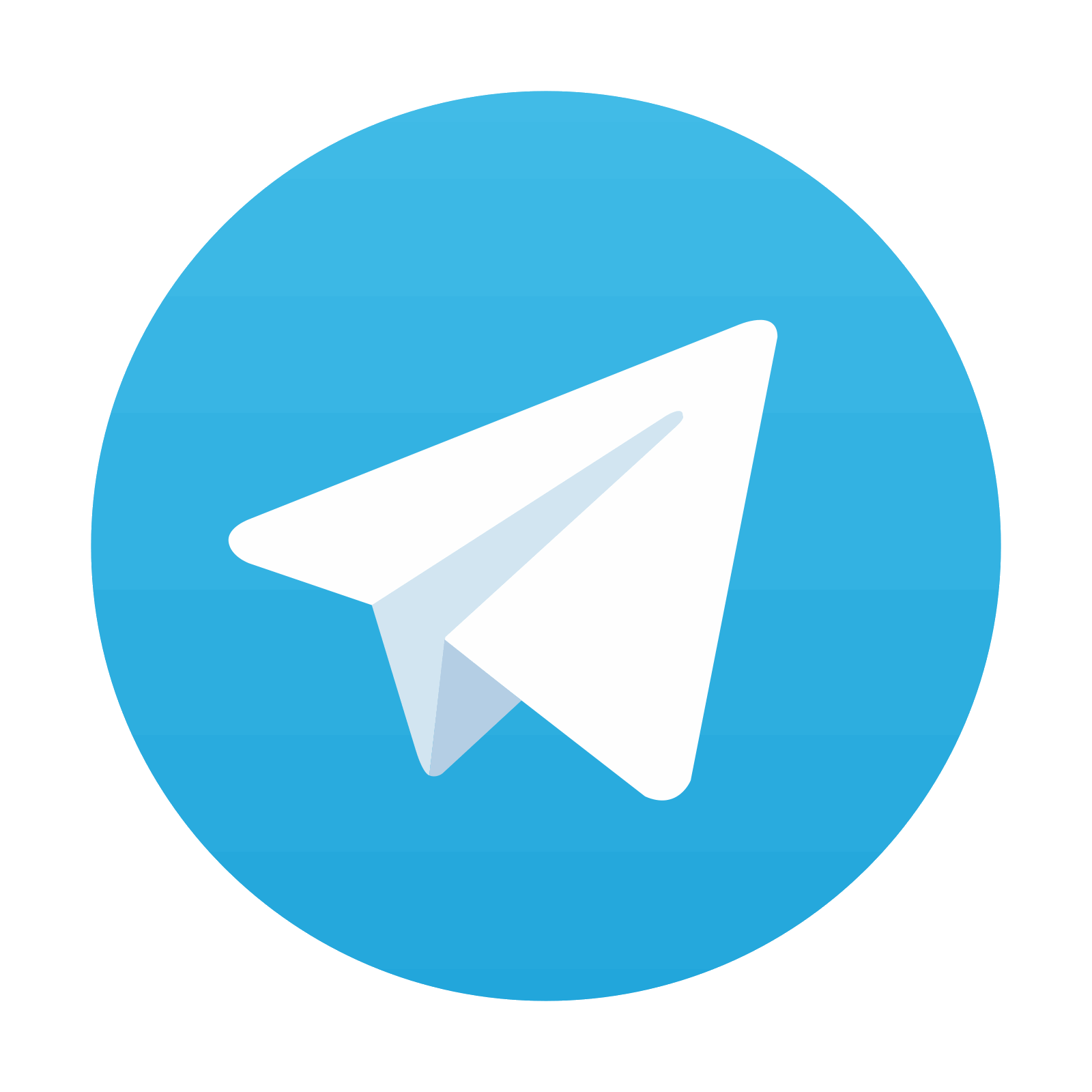