Abstract
The fetal–placental–maternal unit can produce significant abnormalities in the neonate’s hematologic health at birth. A newborn can have disorders of white blood cells, red blood cells, or platelets, or any combination thereof. Neonatal cytopenias can result from dilution, peripheral destruction, or a defect in cellular production [1]. Maternal illness can be the cause of such abnormalities (Table 23.1). Close communication between the obstetrical provider and the pediatrician is important. This can allow for anticipation of a problem in order to mitigate the consequences, or to discover the cause if an unexpected cytopenia is detected.
Introduction
The fetal–placental–maternal unit can produce significant abnormalities in the neonate’s hematologic health at birth. A newborn can have disorders of white blood cells, red blood cells, or platelets, or any combination thereof. Neonatal cytopenias can result from dilution, peripheral destruction, or a defect in cellular production [1]. Maternal illness can be the cause of such abnormalities (Table 23.1). Close communication between the obstetrical provider and the pediatrician is important. This can allow for anticipation of a problem in order to mitigate the consequences, or to discover the cause if an unexpected cytopenia is detected.
Table 23.1 Causes of cytopenia in the newborn related to the fetomaternal unit
Thrombocytopenia | Anemia | Neutropenia | |
---|---|---|---|
Maternal sepsis | XX | XX | XX |
Preeclampsia/eclampsia | XX | XX | |
Immune disorders | XX | XX | XX |
Congenital infection | XX | XX | XX |
Diabetes mellitus | X | XX | XX |
|
|
|
Thrombocytopenia
While only 1%–5% of all neonates will have a platelet count below 150,000/µl, as many as 35% of infants admitted to the neonatal intensive care unit will have this finding [2]. The most common cause of early onset thrombocytopenia is chronic fetal hypoxemia such as occurs with intrauterine preeclampsia and its variants, and uncontrolled diabetes. While the pediatrician should be open to other diagnostic possibilities, this type of thrombocytopenia is usually self-limited and mild and results from a bone marrow effect with a resulting decrease in megakaryocytes.
Additional causes related to the maternal–fetal unit include immune-mediated disorders such as neonatal alloimmune thrombocytopenia caused by isoimmunization of the mother against specific platelet antigens. Mothers with autoantibodies against platelet antigens can pass those antibodies transplacentally to the infant resulting in neonatal thrombocytopenia. Other causes include intrauterine infections, and neonatal sepsis.
Anemia
Neonatal anemia, defined as a hemoglobin or hematocrit more than two standard deviations below the mean for gestational age can occur due to blood loss, diminished production or increased destruction of red blood cells.
Blood loss can occur prior to delivery in the setting of twin-to-twin transfusion syndrome (TTTS), placental abruption or fetal-to-maternal hemorrhage (FMH). During delivery, blood loss can occur due to transplacental-incision during Cesarean delivery or with internal neonatal bleeding. Infants who suffer rapid, large volume losses typically present with acute distress and shock while those with chronic blood loss anemia may be without symptoms other than pallor. Decreased red blood cell (RBC) production due to abnormalities in the fetal–placental–maternal unit is most commonly related to maternal infections, such as with parvovirus B19 which suppresses the RBC progenitor cells, or Kell isoimmunization which behaves similarly.
Of the three mechanisms for neonatal anemia, increased RBC destruction is the most common. Immune-mediated hemolysis can result from RBC antigens such as D, Duffy, and other irregular antibodies, autoimmune disorders such as neonatal lupus, and maternal infection [3]. Intrinsic red cell disorders such as enzymopathies, membrane defects, hemoglobin disorders, mechanical destruction as seen in vascular disorders or DIC should also be considered in the infant with hemolysis.
Neutropenia
The most common variety of congenital neutropenia is decreased white blood cell (WBC) production related to maternal gestational hypertension. In this case, there is neither a left shift nor any morphologic abnormalities. Gestational hypertension-associated neutropenia is usually self-limited by day 5. An additional perinatal cause of congenital neutropenia is bacterial infection, resulting in accelerated neutrophil use, accompanied by a left shift and morphologic changes such as toxic granulation, vacuolization and Döhle bodies. In this case, the neutropenia resolves as the infection resolves. Finally, neonatal neutropenia can be caused by antineutrophil antibodies from the mother, as occurs with maternal systemic lupus erythematosus or in alloimmune neonatal neutropenia, a disorder similar to red blood cell isoimmunization except that the antibodies are directed against neutrophils, which leads to their destruction [4].
Placental Causes of Anemia
Placental abnormalities may lead to fetal blood loss (Table 23.2).
Table 23.2 Causes of fetal blood loss
Placental |
Abruptio placenta |
Placenta previa (if fetal vessels are torn) |
Placental laceration at operative delivery |
Umbilical-vessel injury during amniocentesis |
Umbilical-cord rupture |
Transfusion syndromes |
Fetomaternal transfusion |
Fetofetal transfusion (twin–twin transfusion) |
Abruptio Placenta and Placenta Previa
While the majority of blood loss with placenta previa or abruptio placenta is maternal, fetal blood loss can also occur [5, 6]. The frequency of neonatal anemia requiring transfusion increases with the severity of maternal bleeding [7]. Vaginal blood can be tested for fetal hemoglobin using the Apt test [8]. Vaso previa is a relatively rare phenomenon where fetal vessels within membranes, either due to a velamentous insertion of the vessels or bridging vessels from the main body of the placenta to a succenturiate lobe overlie the cervical os. The risk of severe fetal hemorrhage with delivery is very high with vasa previa and can be improved with antenatal diagnosis [9].
Placental or umbilical cord damage can cause neonatal anemia. For instance, umbilical cord blood rupture may occur [10], especially with traumatic delivery or vasa previa. Surgical laceration of the placenta, as may occur with cesarean section, may cause significant fetal blood loss. Vascular anomalies of the umbilical cord can lead to neonatal anemia [8]. Although uncommon, a traumatic amniocentesis or cordocentesis can result in significant fetal hemorrhage [11].
Infants who have experienced acute hemorrhage during fetal life present with clinical features of acute anemia: pallor, hypovolemia, and hypotension. Unlike patients with hemolytic disorders, these infants do not typically develop hyperbilirubinemia. A hemoglobin measurement immediately following birth often does not accurately reflect the severity of the bleeding. Furthermore, because capillary blood counts are generally higher than central measurements, especially in the acidotic infant, they may underestimate anemia [12]. The management of such infants initially consists of volume replacement, usually with volume expanders such as Ringer’s lactate or normal saline. Red blood cell transfusion may be indicated for large fetal blood loss.
Fetomaternal Bleeds
Small quantities of fetal erythrocytes pass into the maternal circulation in the majority of pregnancies [13]. In approximately 98% of pregnancies, less than 2 ml of fetal cells are found in the maternal blood. However, in about 0.1%–0.3% of pregnancies, this volume exceeds 20–30 ml [14, 15]. In a retrospective analysis of a large database of deliveries, Christensen et al. found an incidence of FMH of 1:9160 live births [16].
The estimated acute fetal blood loss can be calculated based on the number of fetal erythrocytes in the mother’s blood (see below) and may be underestimated with chronic bleeding or blood group incompatibility. The presence of pregnancy complications, preeclampsia, cesarean section, maternal trauma, placement of an intrauterine pressure catheter, or complicated delivery have been reported to cause significant fetomaternal bleeding [13, 17]. Massive fetomaternal hemorrhage can cause symptomatic anemia or even fetal demise [18, 19]. Chronic fetomaternal bleeding can cause nonimmune hydrops [20]. The presence of symptomatic anemia is dependent upon both the amount of and the time course of the hemorrhage, i.e., a large acute bleed presents with hypovolemic shock, whereas chronic bleeding may present with pallor or congestive heart failure.
Diagnosis
The usual approach is to examine maternal blood for fetal erythrocytes using the Kleihauer–Betke stain or flow-cytometric techniques [21, 22]. These two techniques correlate well [23, 24], but there are data to show that anti Hb F flow cytometry has greater precision [25] and some laboratories may only offer one of these tests. Anti-D flow cytometry is only useful in the setting of a mother who is D antigen negative and a fetus who is D antigen positive. The formula used to determine the amount of fetal blood in the maternal circulation is [26]:
Variations of this formula have been reported and a normal fetal Hct should be assumed rather than the actual fetal Hct as a massive FMH may cause severe fetal anemia [27].
Because fetal cells may be cleared quickly from the maternal circulation, delay in assessment may prevent the identification, or underestimate the volume, of fetal blood lost into the maternal circulation, especially if there is a blood-group incompatibility between the infant and mother. False-positive results of Kleihauer–Betke testing can occur if the mother has an increased percentage of hemoglobin F in her own cells, as may occur with the hereditary persistence of fetal hemoglobin, aplastic anemia, or use of cancer chemotherapy [28]. Combined antibody flow cytometry may occasionally useful in this setting [27]. Fetal ultrasound abnormalities and/or non-stress test may not be reliably sensitive to the identification of fetal anemia but Doppler studies of fetal middle cerebral artery (MCA) flow velocity are a useful technique to identify fetal anemia [29–31]. Fetal tachycardia and/or a sinusoidal wave pattern on fetal heart rate may be suggestive of fetal anemia [31, 32].
Management
As with acute hemorrhage, the initial management of the neonate with acute severe fetomaternal bleeding is volume expansion using isotonic crystalloid solutions and possibly red blood cell transfusion. Chronic bleeding, not resulting in severe or symptomatic anemia, can be managed with iron supplementation.
Outcomes
Rubod found that massive fetomaternal hemorrhage accounted for 1.6% of all fetal deaths identified over a 7 year review in two university hospitals for an overall rate of 1.3 deaths from this disorder per 10,000 live births [33]. Risk factors for adverse neonatal outcomes include an initial neonatal hemoglobin <4 g/dL, or an estimated fetal blood loss of >20 ml/kg [15, 33, 34]. Similarly, there was a 71% rate of adverse outcomes in Christensen’s series with risk factors including an initial Hb < 5 g/dL or birth at less than 35 weeks gestational age [16]. When the calculated fetal blood loss is corrected for the estimated fetoplacental blood volume, there is good correlation with the fetal/neonatal hemoglobin concentration [15].
Twin–Twin Transfusion Syndrome
Essentially all monochorionic placentas have intraplacental anastomatic vessels joining the circulation of one twin to the other. In the normal monochorionic, diamniotic twin placenta there is a mean of eight such anastomoses [35]. These anastomoses are the basis for many of the complications unique to this type of twinning. While this physical characteristic is fundamental to these disorders, the pathophysiological basis is as yet poorly understood. The nomenclature for these disorders is changing, with the umbrella term considered by many to be twin–twin transfusion syndrome (TTTS). Within this is what many still call twin-to-twin transfusion syndrome (the term used in this chapter) while others use twin oligohydramnios polyhydramnios syndrome (TOPS), twins anemia polycythemia syndrome (TAPS), and acute twin–twin transfusion syndrome.
Within monochorionic diamniotic placentas there can be arterio-arteriolar (AA), veno-venous (VV), and aterio-venous (AV) anastomosis. The AA and VV anastomosis allow for bidirectional flow, while the AV anastomosis allow for only unidirectional flow. It is the arterio-venous anastomoses that are the cause of these pathologic conditions. In fact, the presence of many arterio-arterio anastomoses is relatively protective against TTTS [36].
Twin–twin transfusion syndrome occurs in 5.5%–17.5% of monochorionic, diamniotic monozygotic twins [36]. It results from imbalanced blood traversing from the donor twin to the recipient twin through arterio-venous anastomoses. The donor twin develops anemia, hypovolemia, oliguria, and oligohydramnios while the recipient twin develops polycythemia, hypervolemia, polyuria, polyhydramios and in severe forms, hydrops fetalis. The hormonal response includes activation of the renin-angiotensin system in the donor, with increase tubular reabsorption, increased angiotensin 2 production, worsening oligohydramnios and vasoconstriction [37].
The increased blood volume of the recipient twin increases atrial pressure, resulting in cardiac atrial natriuretic peptide synthesis. The hormonal response ultimately suppresses antidiuretic hormone production due to increased glomerular filtration rate and a decrease in tubular reabsorption, resulting in an increase in urine production and resulting polyhydramnios. Because the donor’s angiotensin 2 crosses through the anastomosis to the recipient, there can be hypertensive microangiopathy in both twins [37].
Historically, neonatal TTTS was diagnosed when there was a >5 g/dl difference in hemoglobin concentration and a >20% difference in birth weight between the twins. These criteria are no longer used as they may be present in both monochorionic twins without TTTS and in dichorionic twins [36]. TTTS can develop rapidly or indolently, and at various times in pregnancy and as such, after 16 weeks, ultrasound examination of monochorionic diamniotic twins is recommended every other week [38]. The goal is to identify those twins at high risk of extreme prematurity, fetal or neonatal death, or neurologic injury so that in utero intervention, or delivery if appropriate, can be offered.
Sonographic findings include discordant fetal growth with abnormal amniotic fluid volumes, inability to visualize the bladder or stomach of the smaller twin, a large and possibly hydropic twin with polyhydramnios and a persistently full bladder. Fetal Doppler is useful as well to help predict outcomes. Severely abnormal umbilical artery Doppler findings, such as absent or reversal of the end diastolic flow of the cord of the donor predicts a high risk of perinatal death. Measurement of the peak systolic velocity in the middle cerebral artery (MCA) is useful is used to determine the presence and degree of fetal anemia. Staging systems using these findings, and in some centers fetal echocardiogram findings, are used to predict outcomes and to determine interventions [39].
In 2004, Senat and colleagues reported a randomized trial of selective fetal laser ablation of fetoscopically identified placental surface anastomosis versus amnioreduction of the polyhydramniotic sac [40]. There was a significant improvement in the survival of at least one twin on day 28 of life (76% vs. 56%) and a lower risk of neurologic injury among survivors (6% vs. 15%) in those who underwent laser intervention. Since this trial, modifications in the instrumentation and technique have further improved outcomes. The current most commonly used method is the so-called Solomon technique which was developed to decrease the risk of incomplete closure of significant anastomoses which could lead to recurrent TTTS or twin anemia polycythemia syndrome (TAPS). In the Solomon technique, after selective laser ablation of identified anastomoses, the placental equator is lasered to attempt to completely separate the two circulations. The Solomon technique reduces the persistence of anastomoses after selective laser ablation from 34% to 19%, recurrent TTTS from 7% to 1% and TAPS from 16% to 3%, without increased complications [41]. This technique is the preferred approach. For patients without access to laser therapy or who present after 26–28 weeks, serial amnioreduction may be used.
Twin Anemia Polycythemia Syndrome
Twin anemia polycythemia syndrome (TAPS) occurs most commonly after laser therapy for TTTS but can occur spontaneously. Very small, <1 mm diameter, AV anastomoses are required for TAPS to occur. Through these very small connections, chronic low volume transfusion occurs. Flow has been measured using Doppler technology to be 5–15 ml/24 hours [35]. There are a variety of definitions for TAPS but in general, there are large intertwin differences in hemoglobin levels without polyhydramnios and oligohydramnios. Prenatally, the diagnosis is made when the peak systolic velocity of the MCA in the anemic fetus is >1.5 multiples of the median (MoM; corrected for gestational age) and < 1.0 MoM in the polycythemic one. Postnatally, the diagnosis is made when there is an intertwin hemoglobin difference of ≥8 g/dL and at least one of the following: small residual anastomoses identified on the placental surface using a vessel-injection technique OR a reticulocyte count ratio of >1.7:
Robyr reports that in 12/13 such cases, the original recipient twin became the donor [42]. Persistent hemoglobin differences after laser therapy is considered a failure of the ablation and have been significantly reduced in frequency with the Solomon technique, but still can occur. Close observation with twice weekly MCA Doppler studies is recommended after laser therapy to detect either recurrent TTTS or TAPS. Treatment depends on gestational age and whether a repeat laser treatment is possible. Without the polyhydramnios to improve visibility fetoscopically, a repeat procedure can be quite difficult. Other therapies which have been suggested include either of both intrauterine transfusion for the anemic fetus with partial exchange transfusion for the polycythemic fetus to decrease the hypercoagulation problems [43].
Acute Intertwin Transfusion
Another relatively rare form of TTTS occurs when there is an acute transfusion of a significant volume of blood from one twin to the other at the time of delivery. While the diagnosis may be suggested by a difference in hemoglobin concentration, it should be recognized that in acute blood loss, hypovolemia may precede anemia. The donor infants may require early intervention with volume expanders and/or blood transfusion [36].
When one of a monochorionic twin pair dies in utero, that fetal–placental unit becomes a low pressure sink for the surviving twin. Via the anastomosis, there can be an immediate exsanguination from the surviving twin, resulting in acute anemia and hypovolemia with resulting death, or possible intracerebral and renal ischemic injury. The neonatal hematologic status will depend at least in part on how remote from the event that delivery occurs.
Clinical Manifestations
Most infants with TTTS are born early and suffer complications of prematurity. Following laser therapy, prematurity is still a problem, occurring in 17% prior to 28 weeks, 30% before 32 weeks and 50% before 34 weeks [37]. The neonatal findings will then not only reflect the placental anastomosis-mediated diseases, but also the consequences of prematurity.
In a study of patients treated in their center, Verbeek et al. looked at the hematologic outcomes of the donor and recipient twins as to whether they had been treated conservatively (expectant management or serial amnioreduction), treated with complete laser coagulation [44]. In addition, they assessed those treated with complete laser coagulation but who had persistent TTTS or TAPS. The results, shown in Table 23.3 show the incidence of anemia in the donor twin and polycythemia in the recipient twin by whether the twins were treated conservatively, had a complete laser, had persistent TTTS after laser (incomplete laser), spontaneous TAPS, or acute peripartum TTTS. Table 23.4 shows the need for blood transfusions in donor twins and plasma exchange transfusion in recipient twins if treated conservatively or having persistent TTTS or TAPS, but much lower rates for twin pairs treated successfully.
Table 23.3 Requirement for transfusion (donor twin) or partial exchange transfusion (recipient twin) on day 1 in TTTS
Transfusion day 1 donor | Partial exchange transfusion day 1 recipient | |
---|---|---|
| 33% (14 of 43) | 24% (10 of 42) |
TTTS with laser treatment | 5% (13 of 251) | 1% (2 of 252) |
TTTS + TAPS | 55% (26 of 47) | 35% (16 of 46) |
Notes: TTTS, twin-to-twin transfusion syndrome
TAPS, twin anemia–polycythemia syndrome
Adapted from [44].
Table 23.4 Neonatal hematologic outcome in TTTS, TAPS, and acute TTTS
Anemia | Polycythemia | Either twin pair | |
---|---|---|---|
TTTS conservative | 32 | 24 | 42 |
TTTS incomplete laser (TAPS) | 55 | 35 | 78 |
TTTS Complete laser | 5 | 1 | 7 |
Spontaneous TAPS | 82 | 53 | 82 |
Acute peripartum TTTS | 28 | 28 | 55 |
Note: Adapted from [44].
In addition to plethora, a number of other systems may be involved in the recipient. Cardiac findings include biventricular hypertrophy and tricuspid regurgitation [45]. Hyperviscosity secondary to polycythemia perhaps with the addition of vasoconstriction due to activation of the renal angiostensin system can result in vascular occlusion which may manifest with limb necrosis [46, 47]. Broadbent identified 16 cases of postnatal limb ischemia in one of a twin pair [48]. In nine cases, TTTS was identified and in all the limb ischemia occurred in the recipient twin. In the remaining cases, sepsis was the cause in one and TTTS was neither reported nor excluded in the others [48]. Hypoxic/ischemic events have also been noted [39].
The donor twin may exhibit cutaneous erythropoiesis (manifested as blueberry-muffin rash [49, 50], neutropenia [51], and/or renal failure [52, 53]. Thrombocytopenia, usually self-limited, occurs more commonly in neonates affected by TAPS than in uncomplicated monochorionic twins [44].
Neonatal Management
The type of antenatal therapy, if any, and results of post procedure can inform the neonatologist and hematologist of the most likely concerns for the neonate. The maternal fetal medicine and obstetrical teams should discuss this with in detail prior to delivery with the neonatologist. Depending on the acuity process preceding delivery, one or both of the neonates may be very non-euvolemic.
Postnatally, the donor twin may require volume expansion. If the twin is severely hypovolemic and/or anemic, then red blood cell transfusion may be indicated. Glucose infusions may be necessary for hypoglycemia in the donor twin. Long-term iron supplementation should be administered to the donor twin. The recipient twin should be evaluated for complications of the polycythemia/hyperviscosity syndrome, such as respiratory distress, jitteriness, seizures, hypocalcemia, hypoglycemia, and hyperbilirubinemia. The affected twin may require partial exchange transfusion. The complications and management of the polycythemia/hyperviscosity syndrome are discussed in Chapter 11.
Placental Tumors
In neonatal anemia with no obvious explanation, pathologic examination of the placenta may provide an explanation and should be requested promptly prior to disposal of the placenta. Disorders that alter the fetal vascular integrity of the placenta can cause significant fetal blood loss, either as FMH or potentially into the amniotic fluid, depending on the site of the abnormality. Chorioangiomas are the most common placental tumour and are typically small, single and of no significant consequence [54]. Giant placental chorioangioma, typically defined as greater than 4 cm in size, however, can cause microangiopathic hemolytic anemia, thrombocytopenia, and non-immune hydrops fetalis [55, 56]. Multiple chorioangiomas (also known as chorioangiomatosis) are extremely rare and have been associated with severe anemia, thrombocytopenia and fetal growth restriction [57]. Despite their similar morphology, in a case-controlled survey study, infantile hemagiomas were not seen in increased frequency in infants of pregnancies complicated by placental chorioangiomas [58].
Choriocarcinoma within the placenta is extremely rare, and can cause fetal to maternal hemorrhage, as well as metastatic fetal and maternal disease. Intraplacental choriocarcinoma is accompanied by FMH quite frequently and can results in fetal anemia and death [59, 60]. These rare tumors may present with cutaneous nodules that can be mistaken for hemagiomas [61].
Fetal Growth Restriction
A variety of maternal or placental conditions can cause fetal growth restriction (FGR). In general, maternal vasculopathies (systemic or local) can result in poor placental development, which ultimately results in FGR. Examples of maternal systemic vasculopathic disorders include: hypertension, long-standing diabetes, and lupus and lupus-like disorders. The neonatal hematologic effects of these disorders on the fetus may by compounded if the maternal disease results in fetal growth restriction. Local vasculopathies, such as Müllerian abnormalities in the uterus or uterine fibroids may result in placentation in an area of abnormal maternal vasculature.
In the face of abnormal gas and nutrient exchange with placental insufficiency, there are many adaptive mechanisms. Blood flow to vital structures, such as the fetal brain, adrenal gland, heart, and placenta are preserved at the expense of normal perfusion of the bone marrow, lungs, gut, and musculature. This redistribution of blood flow results in cerebral vasodilation, peripheral vasoconstriction, and increased end-diastolic pressure in the ventricles. The hematologic effects, including polycythemia, thrombocytopenia and neutropenia, in the growth restricted fetus can vary and seem to be related to the severity of the fetal hypoxemia [62]. Elevated nucleated RBCs (adjusted for gestational age) are a marker for intrauterine hypoxia [63, 64].
Fetal Doppler measurements have been correlated with abnormal hematologic profiles in the FGR neonate. Critically abnormal umbilical artery Doppler studies (such as absent end diastolic flow or reversed diastolic flow) are evidence of very high resistance of flow through the placenta. Increases in the degree of pulsatility in the middle cerebral artery suggest cerebral vasodilation. In a study of 100 small for gestational age fetuses, Martinelli et al. showed that abnormal umbilical artery Doppler results correlated positively with elevations in neonatal nucleated RBC levels and negatively with platelet and white blood cell counts [65]. Similarly, Baschat and colleagues have shown that crucially abnormal umbilical artery Doppler measurements in the FGR fetus are associated with increasing nucleated RBC levels [66]. This group hypothesize that progressive fetal hypoxemia triggers the release of erythropoietin and RBC production and release at both intramedullary and extramedullary sites [67]. Furthering this point, they later showed that rate of progression of fetal Doppler abnormalities correlated with increased NRBCs and decreased Hb and platelets in the newborn after delivery [68].
There is also an effect on platelets. Stimulation of some antiplatelet factor by fetal acidosis, hypoxemia, or hypotension has been invoked by Baschat et al. as the mechanism to explain the 10 fold higher rate of neonatal thrombocytopenia in the growth-restricted fetus with absent or reversed umbilical artery Doppler measurement [66].
Diabetes
Maternal diabetes can have profound effects on fetal development. Maternal diabetes may predate the pregnancy or can develop during pregnancy (gestational diabetes). It is estimated that 0.2%–0.3% of pregnancies are affected by pre-existing diabetes mellitus and 1%–5% are affected by gestational diabetes [69]. Reported problems in infants of diabetic mothers (IDM) include decreased survival and increased rates of prematurity and frequency of both small for gestational age (SGA) and large for gestational age (LGA), although the LGA group predominates. Insulin does not cross the placenta, and hence maternal hyperglycemia causes fetal hyperglycemia and resultant fetal hyperinsulinemia. Polycythemia and thrombosis are the most significant hematologic complications in the IDM.
Polycythemia in Infants of Diabetic Mothers
Infants of diabetic mothers have an increased incidence of polycythemia [70, 71]. Mimouni and colleagues found that the incidence of polycythemia (venous hematocrit (Hct) ≥65% at 2 hours of age) in IDMs was 29% versus 6% for infants matched for gestational age, mode of delivery, and Apgar scores [72]. The hematocrit of the infant did not correlate with maternal glycosylated hemoglobin levels but did correlate with neonatal hypoglycemia. In contrast, Cordero et al. identified polycythemia in only 5% of IDMs in their cohort, although Hb were not done specifically at 2 hours and only done in roughly half of their cohort [73]. Green and colleagues found that the hematocrit of IDMs correlated with maternal glycosylated hemoglobin levels at term (average gestation 38 weeks) but not at 36 weeks’ gestational age [74]. This may suggest that the maternal glucose control in late gestation has the greatest influence on the incidence of polycythemia.
Several factors may contribute to polycythemia in the IDM. Fetal hyperglycemia causes fetal hyperinsulinemia and insulin itself may promote erythropoiesis [75, 76]. Widness and colleagues found that the umbilical-vein erythropoietin concentrations were elevated in IDMs and correlated with maternal HbA1c levels taken the month before delivery [77]. Fetal erythropoietin concentrations correlate with fetal insulin levels [78]. Nucleated RBC levels, which may be a marker of fetal hypoxia, are also elevated in the IDM [79, 80]. There is a delayed switch from gamma- to beta-chain production in the IDM [81, 82]. Cetin et al. found a correlation between maternal β-hydroxybutyrate levels at 34–36 weeks and polycythemia in their newborns [83].
The complications of the polycythemia/hyperviscosity syndrome such as hypocalcemia, hypoglycemia, and hyperbilirubinemia should be expected and managed (see Chapter 11).
Thrombosis in Infants of Diabetic Mothers
The incidence of thrombosis, especially renal-vein thrombosis, is increased in IDMs [71]. Clinical manifestations of renal vein thrombosis may include shock, vomiting, hematuria, and a palpable kidney. Both venous and arterial thrombosis can occur in the IDM. While reported cases of peripartum gangrene of the limb are rare, 22% of one series were reported to be in IDMs [84]. One study found an association of perinatal arterial ischemic stroke with gestational diabetes [85].
It is likely that the increased incidence of thrombosis in the IDM is multifactorial. Polycythemia causes increased blood viscosity. Birth trauma, in part caused by macrosomia, may lead to vessel damage. Both platelet and plasma factors place the IDM at increased risk for thrombosis. Hathaway and colleagues suggested that there is increased platelet consumption [86]. Stuart and colleagues documented increased platelet reactivity and platelet endoperoxide formation in diabetic mothers and transiently in their infants [87]. Umbilical arteries from IDMs born to mothers with elevated HbA1c values produce significantly less prostacyclin, a potent inhibitor of platelet aggregation, than those obtained from control infants or IDMs of mothers with normal values for HbA1c [88]. Sarkar et al. found that protein C levels were lower in IDMs-either gestational diet controlled or insulin dependent, but there was no difference in the frequency of factor V Leiden, prothrombin gene mutation P20210A, methylenetetrahydrofolate reductase C677 T, or other common genetic risk factors [89]. Easa and Coen failed to find a difference in the prothrombin time, activated partial thromboplastin time, fibrinogen, factors V, X, or XII, or von Willebrand antigen [90]. Ironically, they found slightly lower levels for factor VIII and increased levels of antithrombin. Antiplasmin concentrations are increased in mothers with diabetes and their infants [91]. Elevated levels of homocysteine, a known risk factor for thrombosis have also been reported in IDM [92].
Thrombocytopenia in Infants of Diabetic Mothers
Mild thrombocytopenia is seen in IDM [86, 90]. The mean platelet count in IDMs was shown to be lower than in matched controls and did not correlate with maternal glycemic control [80]. Usually, no specific therapy is indicated.
Immunologic Changes in Infants of Diabetic Mothers
A few reports of immunologic abnormalities in IDMs have been reported, but their significance is not yet clear. Mehta and Petrova reported decreased neutrophil chemotaxis, random motility and chemiluminescence in IDM when compared to neonatal controls [93]. Infants of IDDM and gestational diabetes have reduced natural killer cells compared to healthy controls [94]. Roll et al. reported decreased numbers of both T and B lymphocytes in infants of insulin-dependent mothers [95].
Hypertensive Disorders of Pregnancy
Maternal hypertensive disorders (MH) is a well-recognized and potentially serious complication. Hypertension in pregnancy is divided into four categories: (1) preeclampsia/eclampsia, (2) chronic hypertension, (3) chronic hypertension with superimposed preeclampsia, and (4) gestational hypertension. Gestational hypertension is defined in women who develop elevated blood pressure without proteinuria after 20 weeks of gestation without symptoms of preeclampsia or eclampsia [96]. Risk factors for preeclampsia are noted in Table 23.5.
Table 23.5 Risk factors for preeclampsia
Nulliparity |
Extremes of maternal age |
Multiple gestation |
Chronic hypertension |
Pre-existing diabetes mellitus |
Collagen-vascular disease |
African American |
Antiphospholipid syndrome |
Obesity |
Prior pregnancy with preeclampsia |
Nephropathy |
Eclampsia is diagnosed when a woman with preeclampsia develops new onset of seizures or altered mental status. HELLP (hemolysis, elevated liver enzymes, and low platelets) syndrome is considered by most to be a variant of severe preeclampsia and is diagnosed in the presence of maternal hemolysis, elevated liver enzymes and low maternal platelets, usually with hypertension and proteinuria.
Neutropenia in Infants of Hypertensive Mothers
Although the association of neonatal neutropenia with maternal hypertensive disorders (particularly preeclampsia and its variants) is well established, there are conflicting data regarding the epidemiology of the association, risks of neonatal sepsis, and possible mechanisms.
In their classic paper defining the normal neonatal neutrophil count, Manroe and colleagues demonstrated a high incidence of neutropenia in the infants of the mothers with maternal hypertension (IMH) [97]. Since that time, other studies have demonstrated a 40%–50% incidence of neutropenia in infants of mothers with gestaional hypertension (IGH), using Manroe’s data for the normal range [98–100]. Doron and colleagues found an incidence of 48% using normative data developed for premature infants [101]. Of 95 extremely low birth weight (ELBW) infants found to have an ANC <1,000 per microliter in the first 3 days of life (from a total cohort of 388 infants) 68% were either SGA or IGH [102]. Smaller (<1,500 g), more premature (<30 weeks’ gestational age), and/or cesarean-section-delivered IGH are more likely to have neutropenia. The incidence of neutropenia increases with the severity of maternal hypertension [99, 101]. In contrast, some have failed to find an increased incidence of neutropenia in premature IGH compared with matched controls also using normative data developed for premature infants [103].
In a small series, Bolat et al. found neutropenia in 18 of 31 (58%) IMH vs. 6% in a control group. The neutropenia was mild in eight, moderate in six, and severe in four of the infants. Lymphopenia was noted in 36% of these infants and 19% of the control group [104].
It is yet unclear if it is the maternal hypertension or the SGA status of the infant that is the primary contributor to neonatal neutropenia and thrombocytopenia. Christensen et al. did a retrospective review of all infants born SGA with an absolute neutrophil count <1,000/mcL born within a large health system [105]. They excluded from analysis infants with other known causes of neutropenia including early-onset sepsis. Six percent of tested SGA infants had early-onset neutropenia vs. 1% of gestational-age matched controls. The I/T ratio of these infants was within the reference range and their neutrophil counts increased to the reference range by day 7. Sixty-four percent of these infants also had thrombocytopenia. The neutropenia was not independently associated with maternal hypertension beyond their SGA status [105]. In contrast, Bizerea found that neutropenia occurred in 33% of SGA infants of hypertensive mothers and 23% of AGA infants of hypertensive mothers as opposed to only 2.6% of SGA controls [106].
Koenig and Christensen identified decreased neutrophil production, perhaps due to an inhibitor of myelopoiesis, as the cause of neutropenia in the IMH [100]. Other possible causes of decreased white cell production in fetuses of hypertensive women include: a neutrophil production inhibitor in the placenta or in fetal blood; the erythrocyte steal phenomena with intrauterine hypoxemia in which there is a shift towards red cell production; down regulation of white cell production due to placental dysfunction; and FAS-FAS ligand interaction abnormalities [107]. Some studies have shown this neutropenia to last for less than 72 hours [98, 100], while another study reported more prolonged neutropenia [108]. Compared with infants with sepsis-induced neutropenia, the neutropenia in IMH occurs earlier in life and does not have an increased ratio of immature to total neutrophils [99]. Yet, Saini et al. reported that the expression of surface adhesion markers, indicating neutrophil activation, was increased in infants of preeclamptic mothers [109].
There have been conflicting studies as to whether the neutropenia in IMH is associated with an increased risk of infection. Doron and colleagues reported an increased rate of bacterial infection in the first 48 hours of life in neutropenic compared with non-neutropenic IMH [101]. Other studies have shown an increased incidence of late-onset infection, usually after the neutropenia had resolved [99, 100]. Stoll reported from a review of the National Institute of Child Health and Human Development Neonatal Research Network that the presence of maternal hypertension/preeclampsia actually decreased the risk of neonatal infection [110]. Sharma et al. did a retrospective study of IMH [107]. The vast majority of neutropenic children were born prematurely, 86.4% of those from multiple gestations and 80% of the singletons. In this series, only the neutropenic multiple-gestation infants were at increased risk of sepsis compared to non-neutropenic multiples; this was not true of singletons [107].
Neutropenia in IMH may respond to granulocyte colony stimulating factor injections [111]. However, the need for routine use of this medication in the uninfected preterm infant with neutropenia has not been demonstrated to be necessary [103, 112]. Sixteen percent of the infants in Christensen’s series were treated with IVIg or G-CSF without an effect on outcome [105].
Thrombocytopenia in Infants of Mothers with Hypertensive Disorders of Pregnancy
Thrombocytopenia in neonates that presents in the first 72 hours of life is often found in the setting of placental insufficiency and maternal hypertension is a common cause. According to Chakravorty et al., this form of thrombocytopenia is usually benign and is rarely severe, uncommonly results in a rapid drop in platelet count and usually does not require treatment [113]. Early onset thrombocytopenia that differs from this pattern warrants further work up regarding etiology [114].
While thrombocytopenia is seen in 15%–36% of IMH [98–100, 104, 115), it is often but not always mild. In a prospective study from India, platelet counts were assessed in 97 IMH [116]. Of these, 36.1% had thrombocytopenia and in 20% the platelet count was <30,000/μL. Thrombocytopenia was significantly more likely in male children (OR 2.54, CI 1.08–5.95), with a trend towards increased risk associated with prematurity and low birth weight [116]. In a study of thrombocytopenia in hospitalized neonates, McPherson and Juul, using combined data for SGA and IMH, found the percentage of uninfected neonates with thrombocytopenia was higher in SGA/IMH vs. AGA infants for gestational ages <27 weeks (80% vs. 30%); 27<30 weeks (50% vs. 13%) and 30<34 weeks (30% vs. 8%) [117]. Most of their cases of thrombocytopenia attributed to SGA/IMH resolved within 2 weeks. In an analysis of ELBW infants, Christensen found that SGA or IMH was the most common diagnosed cause of thrombocytopenia, accounting for 37% of all cases [118]. There are similar rates of thrombocytopenia for infants born to mothers with HELLP as compared with maternal eclampsia/preeclampsia [119–121]. Disseminated intravascular coagulation (DIC) has been reported in IMH whose mothers’ platelet counts were <50,000/μl [115]. In studies to determine the etiology of thrombocytopenia in IMH, Tsao et al. showed that soluble fms-like tyrosine kinase 1 levels were higher in IMH and inversely correlated with infant platelet counts [122]. Infants with high levels of soluble fms-like tyrosine kinase 1 were more likely to have lower platelet counts, maternal preeclampsia and to be SGA [122]. Thrombopoietin levels did not differ between infants of mothers with and without MH [123]. Megakaryocytopoeitic defects have been postulated [124].
Polycythemia in Infants of Hypertensive Mothers
An increased incidence of polycythemia in IMH has been reported [125]. Brazy and colleagues found the mean hemoglobin level to be 5% higher in IMH [115]. The recommendations regarding the management of polycythemia are outlined in Chapter 11.
Autoimmune Syndromes
Neonatal Lupus Erythematosus
The neonatal lupus erythematosus (NLE) syndrome is believed to be due to the transplacental passage of autoantibodies, usually anti-Ro (SS-A) or anti-La (SS-B). Irreversible congenital heart block arising in the second trimester is the most devastating complication of the NLE syndrome. Cutaneous manifestations of NLE often begin after birth. Other complications include hepatitis and occasionally neurologic manifestations, including seizures.
Transient thrombocytopenia has been noted in roughly 10% of infants with NLE [126, 127]. Zuppa et al. followed 50 infants identified with anti-SS/Ro antibodies at birth; 90% of the antibodies resolved by 9 months of age [128]. Anemia was found in 2% at birth, 24% at 3 months, and 12% at 6 months. Neutropenia was found in 4% at birth, 24% at 3 months, 12% at 6 months, and 2% at 9 months. Thrombocytopenia was found in 2% at birth and none thereafter. None of the hematologic findings in Zuppa’s series caused complications or required treatment [128]. Hariharan and colleagues reported a case of NLE-associated microangiopathic anemia with severe thrombocytopenia that responded to intravenous gammaglobulin and corticosteroid treatment [129]. Recently, a newborn with a perinatal stroke was described who along with his mother had anti-SS/Ro antibodies [130]. There are multiple new agents available for management of individuals with autoimmune disease. Pediatricians should be aware of recommendations regarding the use of such agents in pregnancy and lactation as they develop [131].
Antiphospholipid Antibody Syndrome
An association between the presence of anticardiolipin antibodies and recurrent fetal loss has been well described. The presence of these antibodies in the mother may increase the risk for fetal growth restriction [132]. The presence of anticardiolipin antibody and/or the lupus anticoagulant is associated with an increased risk of maternal venous and arterial thrombosis, although the majority are venous. The cause of the increased thrombosis is not certain, but it appears to be related to platelet activation in the maternal circulation. The IgG isotype of the anticardiolipin antibody can cross the placenta [133].
There have been numerous case reports of fetal and neonatal thrombosis with the maternal antiphospholipid syndrome including the CNS vessels, renal vasculature, vena cava, and aorta [134]. Boffa and Lachassinne described 16 episodes of neonatal thrombosis in infants of mothers with APL: 13 (of 16) were arterial and 8 (of 16) were strokes [135].
The presence of the anticardiolipin antibodies in the newborn is usually transient but may persist for months due to the half-life of transplacentally acquired maternal IgG [136]. Persistent antiphospholipid antibodies have been reported in a cohort of infants with perinatal stroke [137].
Neonatal thrombosis associated with this syndrome should be managed as described in Chapter 19.
Maternal Malignancy
Cancer complicates approximately 1 in 1,000 pregnancies [138–140]. The potential effect of cancer treatment on the fetus, raises serious questions for the management of the mother with cancer [141]. Such cases should be referred whenever possible to a center with the full range of resources and knowledge available to provide the best possible outcomes for the mother and infant.
The most common cancers requiring systemic treatment during pregnancy include breast, ovarian and cervical cancer, lymphoma, leukemia, and sarcoma [138–140]. Chemotherapeutic agents and radiation therapy have been used to treat cancer during pregnancy and several reviews of this topic and specific malignancies are available [138, 142–144].
Antineoplastic agents used to treat cancer can cross the placenta, albeit that maternal pharmacodynamics and placental factors may affect and likely decrease fetal drug exposure [143]. There are differences in both the fetal and maternal physiology that might affect the toxicity of these agents [145, 146]. There is an increase in fetal malformations if chemotherapy in the first trimester [138, 142–144], but the relative risk of chemotherapy-induced malformation appears to decrease significantly when it is administered after the first trimester [139]. Infants exposed to chemotherapy during the second and third trimesters may be at increased risk for preterm birth [147, 148] and/or fetal growth restriction [149]. Maternal chemotherapy may cause fetal functional toxicity. Idarubicin-induced fetal cardiotoxicity has been reported [150], but a longer-term follow up study did not find cardiac issues in 26 infants exposed to anthracycline in utero [147]. Myelosuppressive chemotherapy may cause neonatal pancytopenia which may be present at birth or develop in the first few days of life [151], and hence some authors have recommended trying to avoid myelosuppressive chemotherapy after the 35th week of gestation [143].
Much still needs to be learned about the effects of newer chemotherapeutic agents on the fetus. For example, while infrequently studied in infants of mothers treated with rituximab, neonatal B cell depletion has been reported [152]. Pediatricians will need to follow the evolving literature regarding specific agents to determine what if any modifications to care will need to occur for infants exposed in utero.
There is limited, but growing, long-term follow up of children whose mothers were treated with chemotherapy [143, 147, 153]. In a follow-up study of 157 products of intrauterine chemotherapy exposure after the first trimester for maternal cancer (first exposure at 20.1±5.7 weeks) there was no increase in congenital anomalies, preterm delivery, or growth restriction when compared with general population norms [153]. The rate of congenital malformations and long term complications was not increased in survivors of maternal breast cancer reported in a long term registry [154]. Amant et al. failed to find neurocognitive long-term effects [147]. While such studies will need to continue, in particular as new anti-cancer therapies are used, the available data allows the pediatrician to share optimism with the family when a mother has been treated with chemotherapy while pregnant.
The topic of radiation administered during pregnancy has been reviewed [155, 156]. Fetal growth restriction, microcephaly, eye, and central nervous system (CNS) abnormalities are the predominant complications of intrauterine exposure to ionizing radiation in humans [157]. Hematopoietic, hepatic, renal, and cutaneous effects of radiation therapy have been reported when the fetus is exposed to radiation late in gestation. Factors that affect fetal dose include size of the radiation field, the target dose, the distance from the field edge to the fetus, shielding measures, radiation machine used, and leakage [155, 158, 159]. The pediatrician should be cognizant of potential fetal radiation exposure and include this on their problem list for long-term monitoring as the literature on such exposed infants evolves.
Placental Metastases
While primary placental malignancies arise from gestational trophoblastic neoplasia including partial and complete hydatiform moles and choriocarcinoma, maternal malignancy rarely spreads to the fetus or the placenta [160]. In 2002, Walker et al. reviewed 68 cases of maternal malignancy metastatic to the fetus (21%) and placenta (79%) [161]. Malignant melanoma is the most common malignancy to metastasize to products of conception, followed by breast cancer, leukemia/lymphoma, and lung cancer [161]. Hurley et al. describe a case where the mother died shortly of non-Hodgkins lymphoma shortly after delivery while the infant was diagnosed with a histologically identical tumor at 2 months of age [162]. Hence, the placenta should be carefully examined in cases of known maternal malignancy.
Maternal Medications
There is a vast array of medications, including prescription drugs, non-prescription medications, herbal supplements, native and traditional remedies, and others, that a mother may take during pregnancy. A few examples of medications that may affect the newborn’s hematologic status are noted below. Whenever a neonate presents with early-onset hematologic abnormalities, a careful history of maternal medication use should be obtained with an understanding of placental transfer and potential hematologic consequences.
Effects on Hemostasis
Vitamin K deficiency is seen in 10%–66% of newborns whose mothers have been treated with anticonvulsants, including phenobarbital, phenytoin, and carbamazepam [163–165]. Hemorrhagic disease of the newborn with intraventricular hemorrhage has been reported. The coagulopathy can be reversed with vitamin K administration. While there have been recommendations for pregnant women taking enzyme-inducing anti-epileptic drugs to receive prenatal vitamin K prior to delivery [166], an assessment of the evidence could not confirm or refute the efficacy of this practice [167]. Vitamin K deficiency can also be caused by maternal warfarin ingestion, rifampicin, and isoniazid [168].
As described above, cancer chemotherapeutic agents can cause neonatal thrombocytopenia. Heparin-induced thrombocytopenia (HIT) occurs in a small percentage of individuals treated with heparin. It is manifested by mild thrombocytopenia and, paradoxically, an increased risk for thrombosis. HIT is caused by an antibody directed against the heparin–platelet factor 4 complex. HIT occurs in pregnant women; since the HIT antibody is an IgG antibody that is transported transplacentally, it has been found in cord blood [169].
The risk of venous thromboembolism increases four to five fold during pregnancy and even more in the 3 months following delivery [170]. Low molecular weight heparin and occasionally unfractionated heparin have been the mainstays of management and do not cross the placenta. Other than the potential for HIT as above, they should not affect neonatal hemostasis [170]. Both warfarin and direct thrombin inhibitors cross the placenta and warfarin has been associated with a fetal embryopathy. At this point, direct thrombin inhibitors are not recommended for use in pregnancy [170]. Women with very high thrombotic risk such as those with mechanical heart valves or HIT should be managed by a multidisciplinary team and the pediatrician should be aware of such treatment. Pediatricians should be aware that warfarin may be used in women with mechanical heart valves and be prepared to manage infants exposed in utero [170, 171].
Effects on Red Blood Cells
Glucose-6-phosphate dehydrogenase (G-6-PD) deficiency is by far the most prevalent genetically transmitted erythrocyte enzymopathy, with millions of individuals affected. Fetal hemolysis in G-6-PD deficient fetuses can be triggered by maternal ingestion of compounds known to induce hemolysis in deficient RBCs, including naphthalene, fava beans (broad beans), and several medications such as aspirin, some antimalarials, several sulfonamids, and others. The neonatal manifestations of G-6-PD deficiency are described in Chapter 10.
Anemia and thrombocytopenia was seen in 10/13 infants whose mothers received natalizumab for multiple sclerosis during pregnancy [172]. As more biologic and biosimilar agents are used for a variety of conditions, the pediatrician will need to stay current on potential hematologic toxicities in the newborn.
Effects on Leukocytes
Barak and colleagues reported that infants whose mothers received antenatal administration of betamethasone within 36 hours of delivery had higher leukocyte and neutrophil counts than control infants, and that this effect lasted for up to 7 days [173]. However, the role of antenatal betamethasone exposure in causing leukemoid reactions and their potential sequelae in small infants is less clear. Leukemoid reactions have been noted in infants whose mothers received antenatal dexamethasone [174]. Juul and colleagues found neutrophilia in 28% but also neutropenia in 26% of infants <32 weeks gestational age with antenatal betamethasone exposure [175]. Of 6 infants <34 weeks gestational age with leukemoid reaction in the first 4 days of life, four had antenatal betamethasone exposure within 48 hours of birth [176].
Maternal Nutritional Deficiencies
Anemia in pregnancy is defined as a Hb <11.0 g/dL or a Hct <33% during the first or third trimester or a Hb <10.5 g/dL or a Hct <32% in the second trimester [177, 178]. Nutritional anemias in pregnancy may have important clinical ramifications for both the mother and the developing fetus [178, 179].
Maternal Iron Deficiency Anemia
In 2011, the global prevalence of iron deficiency anemia was estimated to be 38% and in high income countries about 25% [180]. During pregnancy, the mother and the fetus both require iron. The mother’s blood volume increases, both the red cell volume and the plasma volume. The fetus requires iron for use in many cellular processes as well as for red cell production. The average daily requirement for absorbed iron during pregnancy is 4.4 mg/day and increases from 0.8 mg/day in the first 10 weeks to 7.5 mg/day in the last 10 weeks [181]. Iron is required to support the additional red cell volume in the mother, the fetal red cell volume, placental requirements, average daily losses and average perinatal blood loss with delivery [179]. The fetal iron endowment is 75 mg/kg [182]. Iron deficiency may be caused by inadequate nutritional intake, excessive blood losses, including those of preceding pregnancies, or a combination of these problems. In addition to maternal iron deficiency and perinatal blood loss, factors that increase erythropoiesis in the fetus such as maternal diabetes, maternal smoking and fetal growth restriction may be associated with fetal iron deficiency through increased iron demands by the erythron leaving less iron for other tissues [183, 184]. After birth, the supply of iron in breast milk for exclusively breast-fed infants is critical.
It would seem, therefore, that there would be clarity about the benefits of identifying pregnant women with iron deficiency anemia and treating them. The American College of Obstetricians and Gynecologists has recommended (and this was reaffirmed in 2017) that all pregnant women should be screened for anemia during pregnancy and that those with IDA should be supplemented [185]. Contrary to this, the United States Preventive Service Taskforce report in 2015 concluded that the “current evidence is insufficient to assess the balance of benefits and harms of routine iron supplementation for pregnant women or for screening for iron deficiency anemia” [186]. They cited an absence of any study that evaluated the direct effects of routine screening in asymptomatic women on either maternal health or birth outcomes, even though there is evidence that a pregnant woman’s blood counts and indices are improved with these measures.
Many studies of pregnancy outcomes, both maternal and neonatal, have been done in developing countries and may not be generalizable to high income countries. Data regarding the direct effect on neonatal anemia is not robust. There is conflicting data about the association of maternal iron deficiency anemia even indirectly with factors that influence neonatal health. The majority of iron transfer to the fetus occurs late in the 3rd trimester [187]. As such, any association of maternal iron deficiency anemia with preterm birth could influence total body iron for the infant.
In a meta-analysis done to evaluate the population attributable fraction of maternal anemia to poor birth outcomes, Rahman et al. found significant associations with low birth weight (RR 1.31, 95% CI 1.13–1.51), preterm birth (RR 1.63, 95% CI 1.33–2.01), perinatal mortality (RR 1.51, 95% CI 1.30–1.76), and neonatal mortality (RR 2.72, 95% Ci 1.19–6.25) [180]. They calculated that the population attributable fraction in low and middle income countries of maternal anemia to be 12% for low birth weight, 19% for preterm birth and 18% for perinatal mortality.
Counter to that, the Cochrane Collaboration in 2015 analyzed 61 randomized, or quasi-randomized trials to assess the association of maternal preventive iron supplementation on maternal and neonatal outcomes [188]. They found much of the work to be of low quality. Supplementation did significantly reduce maternal anemia at term by 70% (RR 0.30, 95% CI 0.19–0.46), iron deficiency anemia by 67% (RR 0.33, 95% CI 0.16–0.69) and iron deficiency at term (RR 0.43, 95% CI 0.27–0.66). They did report that low quality data showed a reduction in low birth weight babies (8.4% in supplemented patients, 10.3% in unsupplemented patients), they did not find a significant difference in preterm births. Similarly, in a retrospective cohort from a birth registry in the Aberdeen region of Scotland, investigators found no increased rate of premature births [189].
Diagnosis of Iron Deficiency
The diagnosis of iron deficiency can be difficult, because many standard diagnostic measures can be affected by pregnancy. Low ferritin values typically do indicate iron deficiency, however, ferritin is an acute-phase reactant whose levels may increase with many disorders, so a normal ferritin value does not exclude iron deficiency. While levels <12–15 ng/mL are typically used to indicate iron deficiency, higher values may have greater sensitivity [178]. The ratio of serum iron to iron-binding capacity (iron-saturation ratio) can be affected by inflammation, diurnal variation, and acute iron ingestion and is subject to significant variability. The soluble transferrin receptor increases with iron deficiency and is not affected by inflammation [178], and has been used by some to estimate incidence of iron deficiency with pregnancy [190], but is not specific for iron deficiency as levels also increase with ineffective erythropoiesis and may not be routinely evaluable. One small study found that low reticulocyte hemoglobin content correlated well with other measures of iron deficiency in pregnant women [191]. This test may also not be routinely available. Measurements of iron sufficiency and nutrition in the neonate are discussed in greater detail in Chapter 8.
Maternal Iron Status and Infant Iron Status
The effect of maternal iron deficiency on maternal and fetal health has been reviewed [192]. The fetus is quite effective at extracting iron from its mother, even when her iron stores are depleted. There is no correlation between the maternal and neonatal hemoglobin concentrations at birth [192]. Recent studies show that in fact infants born to mothers with iron deficiency likely have decreased iron stores, absorb greater amounts of supplemental iron and are more likely to develop iron deficiency later in infancy [193].
In view of the demonstration that neonatal iron deficiency may have significant impacts beyond anemia, such as neurodevelopment, understanding the impact of maternal iron deficiency on neonatal iron stores is relevant to their care. The neonatal ferritin levels are higher than the mothers in the absence of fetal blood loss, indicating that the placenta is capable of transporting iron across a gradient. The 5th percentile for ferritin for a term infant has been defined as <40 ng/ml and increases during gestation [194].
While the newborn’s hemoglobin concentration may not be affected unless the mother is very anemic, maternal iron supplementation during pregnancy may improve the infant’s hemoglobin levels later in life [193, 195]. Delayed cord clamping to >180 seconds after delivery has been shown to decrease iron deficiency at 3–6 months of age [196, 197].
Folate
Folate is a required cofactor in many one-carbon-transfer reactions. Such reactions are components of the purine and thymidine synthetic pathways and, hence, affect DNA synthesis. Because this step is critical for hematopoiesis, folate deficiency can be a cause of anemia, thrombocytopenia, and neutropenia. Folates are also important in the degradation of histidine and homocysteine. Folate is found in many foods, especially leafy vegetables, fruits, and yeast. It is also found in human and cow’s milk, but it is very low in goat’s milk [198].
In addition to diet, several other factors can affect maternal folate levels. Excessive cooking inactivates folate. Methotrexate, pyrimethamine, and trimethoprim inhibit dihydrofolate reductase and interfere with the production of 5,6,7,8-tetrahydrofolic acid, the active form of folate in one-carbon transfers [199]. Women with hemolytic anemias, tropical or nontropical sprue, other malabsorptive states, as well as those taking anticonvulsive medications and high-dose oral contraceptives have increased folate requirements [199]. Folate deficiency is also seen in women who have undergone bariatric surgery [178].
Folate deficiency in unsupplemented pregnant women is common but may be masked by concomitant iron deficiency. Maternal folate deficiency is associated with an increased risk for fetal neural-tube defects, and fetal growth restriction [200]. Current recommended daily intake for women of childbearing age for folate is 400 μg/day [201]. Folate is transported against a gradient in the placenta and cord levels of plasma folate are higher than maternal levels [199]. In many areas of the world, including North America, folate is added to foods to decrease the incidence of preconceptual maternal folate deficiency and subsequent neural tube defects, however, this is not done universally [202].
Because of the preferential transport of folate to the fetus, deficiency in the immediate newborn period is unlikely. Neonates of mothers with even severe megaloblastic anemia have normal hemoglobin concentrations [203]. Postnatal events such as hemolysis, infection, malnutrition, diarrhea, and liver disease increase the likelihood of folate deficiency in the neonate [199]. The diagnosis should be suspected in the malnourished infant with persistent anemia, especially when associated with thrombocytopenia and/or neutropenia. Bone-marrow examination may reveal megaloblastic hematopoiesis, and hypersegmented neutrophils may be seen on the peripheral blood smear. The diagnosis is confirmed by reduced serum or erythrocyte folate concentrations. Erythrocyte folate levels would be expected to decline more slowly than serum folate levels [199]. Total plasma homocysteine levels may be elevated in folate deficiency.
Cobalamin deficiency should be sought is patients diagnosed with folate deficiency as cobalamin deficiency causes reduced erythrocyte folate concentrations [199], and folate treatment may improve the hematologic parameters but neurologic damage may progress [204]. Inherited disorders of folate metabolism, in particular hereditary folate malabsorption, may affect folate levels or mimic folate deficiency [205].
Treatment
Infants with folate deficiency will respond to folate supplementation. Often doses as low as 200–500 μg may be sufficient, but higher doses may be necessary in cases of intestinal malabsorption syndromes. Doses of 50–200 μg/kg have been recommended for infants [205]. Folate may also be added to hyperalimentation solutions.
Cobalamin
Cobalamin, or vitamin B12, is a cofactor in the conversion of homocysteine to methionine and methylmalonyl coenzyme A to succinyl coenzyme A. The former reaction is critical for creation of intracellular polyglutamated tetrahydrofolate, the functional metabolite of folic acid. Hence, cobalamin deficiency impairs DNA synthesis. In addition to megaloblastic anemia, a peripheral neuropathy with degeneration of the lateral and posterior columns of the spinal cord can occur.
Cobalamin is present primarily in foods of animal origin [206]. A complex series of reactions leads to the absorption and distribution of cobalamin in humans. Intrinsic factor, produced in saliva and gastric juice, combines in the gastrointestinal tract with cobalamin and facilitates binding to a specific receptor in the ileum. The complex is then absorbed, metabolized, and transported through the blood by transcobalamin II [199].
The RDA for cobalamin for pregnant women is 2.6 µg/day and for lactating women 2.8 µg/day [207]. There is preferential transport of cobalamin from the mother to the fetus [207]. While this causes a decline in maternal serum cobalamin levels during pregnancy, the fetal cobalamin content of 50 μg is but a small fraction of the usual maternal stores. Neonatal serum cobalamin levels are higher than maternal levels [208], but fall over the first 6 weeks of age [209]. Maternal cobalamin levels correlate with neonatal levels in infants of mothers with omnivorous diets [209].
Cobalamin deficiency in pregnant women is very common in many underdeveloped areas of the world including the Latin America, sub-Saharan Africa and South Asia [210]. Unlike folate, vitamin B12 is not routinely added to foods. Pernicious anemia is the most common cause of severe vitamin B12 deficiency [206]. Maternal dietary deficiency, due to vegetarian/vegan diets without supplementation may lead to eventual infantile B12 deficiency [211, 212], especially if the infants are exclusively breast fed [206]. In addition to nutritional deficits, other potential causes of maternal B12 deficiency include pernicious anemia, gastrectomy or gastric bypass surgery, ileal resection, inflammatory bowel disease, tropical sprue, gastritis, fish tapeworm infestation, sprue, intestinal bacterial overgrowth, or medications that block vitamin B12 absorption such as metformin or gastric acid inhibitors [199, 206, 210, 213].
Maternal B-12 deficiency is associated with an increased risk for adverse perinatal outcomes including early miscarriage, spontaneous abortion and neural tube defects [210]. It is important to obtain a nutritional, surgical, and medication history from the mother of an infant suspected of having B12 deficiency, however, mothers may be deficient in this vitamin without a history of veganism and without diagnostic abnormalities on their blood counts [214].
Hematologic manifestations of vitamin B12 deficiency include anemia, which is often macrocytic, thrombocytopenia, leucocytopenia and hypersegmented neutrophils [199, 204]. However, cobalamin deficiency was not seen in a series of infants and children with red cell macrocytosis [215]. Bone marrow megaloblastic changes may be seen [204]. Serum cobalamin levels are usually low, but can lack sensitivity and specificity. Additionally, folate deficiency can cause reduced serum cobalamin levels [204]. Therefore, especially in the absence of megaloblastic changes, the diagnosis is confirmed with metabolic markers of functional cobalamin deficiency including elevated homocysteine and methylmalonic acid levels [199]. Nonspecific findings of cobalamin deficiency include elevation of the serum lactate dehydrogenase (LDH), hyperbilirubinemia, and an elevated ferritin [204].
In addition to hematologic findings, symptoms of vitamin B12 deficiency in the infant can include neurologic manifestation that can be severe including hypotonia, neurodevelopmental delay, tremors, hyperirritability, and cerebral atrophy. These may not be fully reversible [199, 206, 214]. Hypogammaglobulinemia has been seen as well [216].
Other causes of B12 deficiency in an infant include malabsorption from resection of the terminal ileum as may occur with necrotizing enterocolitis or congenital disorders that affect cobalamin absorption such as intrinsic factor deficiency, Imerslund–Gräsbeck syndrome or transcobalamin II deficiency [199]. Congenital disorders of cobalamin metabolism may present with similar findings but normal cobalamin levels [199].
Treatment
The treatment of the infant with cobalamin deficiency depends in part on the degree of anemia. Severe anemia should be treated with slow transfusion, as rapid response to cobalamin is unlikely. Rapid transfusion in the severely anemic child may precipitate or aggravate congestive heart failure. Low initial doses, e.g., 0.2 μg/kg, of cyanocobalamin may be given subcutaneously [199]. Serum potassium levels should be monitored carefully, and potassium treatment initiated if indicated, as hypokalemia may develop, especially if large doses of cobalamin are administered. Unless there is documentation of a nutritional etiology for the cobalamin deficiency, the infant should be studied for a defect in cobalamin absorption, transport, or metabolism [199].
Intrauterine Infection
On occasion, the fetus can acquire bacterial, viral, or protozoal infections transplacentally from the mother (Table 23.6). This section will focus on the diagnosis and hematologic manifestations of perinatal infections. Anemia, thrombocytopenia, leukocyte abnormalities, and coagulation abnormalities are common in the fetus with intrauterine infection. As it is not yet possible to rapidly differentiate viral from bacterial infection in the newborn, the initial therapy for most of these patients will usually include empirical antibiotics. Unless specifically stated, the management of the hematologic problems found in these infants is outlined in other chapters. The reader is referred to other references, such as the Red Book®: 2018–2021 Report of the Committee on Infectious Diseases (31st edition) from the American Academy of Pediatrics [217], for specific recommendations regarding the treatment of infants with congenital infections.
Congenital Protozoal Infection
Toxoplasmosis
Toxoplasma gondii is a protozoal feline parasite that can infect humans and other animals. In almost all cases of fetal infection, the susceptible mother has experienced a primary infection that goes unnoticed. Immunocompromised mothers can transmit reactivated disease to the fetus. Maternal primary infection late in pregnancy increases the likelihood of fetal infection, but the consequences of late-gestation fetal infection are fewer [218]. Antibiotic treatment of the mother decreases the likelihood of fetal infection [218]. Toxoplasma infection in the newborn has been reviewed [218, 219].
In recent years, the prevalence of toxoplasmosis is decreasing both in the United States and in Europe [218, 220]. In France it has been estimated to be 2.9 cases per 10,000 live births while in the US it is about 0.5 cases per 10,000 live births. [220].
Clinical manifestations in symptomatic patients include seizures, hydrocephalus, chorioretinitis, fever, hepatomegaly, splenomegaly, and jaundice. Neonatal disease can be generalized or limited to the CNS. Generalized disease appears to be limited to those infants infected in the first two trimesters. The majority of infants with congenital toxoplasmosis have asymptomatic or subclinical disease [218].
Diagnosis
The diagnosis of congenital toxoplasmosis can be based on demonstration of the organism, serologic studies, or antigen testing. Histologic demonstration and/or tissue culture of the organism can be accomplished on several tissues or fluids, including the placenta and amniotic fluid [218]. Serologic tests include the Sabin–Feldman dye test, indirect hemagglutination, the indirect fluorescent antibody test, enzyme-linked immunosorbent assay (ELISA) tests, and others. The sensitivity and availability of these assays vary significantly between laboratories. There are several issues to consider when using humoral responses to diagnose congenital toxoplasmosis. Specific IgG in neonatal serum can be acquired transplacentally from the mother; hence, in the first few months of life, a single positive test for IgG does not confirm neonatal infection. Persistent or rising IgG titers in the infant are considered diagnostic but require serial testing over the first several months of life.
Testing for toxoplasma-specific IgM can be done by several different methods. The IFA test is significantly less sensitive than either the ELISA or the ISAGA; in congenitally infected neonates, the IFA is positive in only 25% versus 75% if ELISA is used. The IFA test can also be rendered falsely negative by high-titer maternal IgG, and is often falsely positive in the presence of rheumatoid factor or antinuclear antibody. The double-sandwich ELISA avoids these pitfalls. Measurement of IgA and IgE is at least comparable to IgM for diagnosing neonatal infection; occasionally, an infant born to a mother with IgA or IgM antibodies to toxoplasmosis will have a transiently false-positive IgA antibody test [221]. While a positive immunoglobulin M (IgM) or IgA serologic test for Toxoplasma in the newborn should be considered diagnostic for congenital toxoplasmosis, the fetus does not begin to make specific antibody until after the 15th to 20th week of gestation. The clinician should also know that most readily available commercial antibody tests for toxoplasmosis are not consistently reliable. In general, it is best to involve infectious diseases trained physicians early in the process and to send serology to a known reliable laboratory for testing.
Polymerase chain reaction (PCR) has been shown to have very high sensitivity and specificity for Toxoplasma [222]. PCR testing of amniotic fluid was shown to have a sensitivity of 64% and a specificity of 100% for the prenatal diagnosis of congenital toxoplasmosis [223]. Peripheral blood leukocytes and cerebrospinal fluid (CSF) amongst others can also be tested by PCR for Toxoplasma [217]. Often, a combination of tests is necessary to confirm infection.
Hematologic Manifestations of Congenital Toxoplasmosis
Anemia has been reported in 4%–64% of infants with congenital toxoplasmosis and is present in most symptomatic infants [224–226]. The incidence of anemia reflects the presence of other manifestations. For instance, 77% of a referral population with symptomatic generalized disease had anemia, as opposed to only 10% of infants identified through serologic screening [218, 225]. The presence of increased nucleated RBCs and reticulocytosis indicate that the anemia is due to hemolysis [224]. Congenital toxoplasmosis can cause nonimmune hydrops [227].
Thrombocytopenia can be seen in symptomatic infection. Alford and colleagues found thrombocytopenia in 10% of prospectively identified infants [225]. Hohlfeld and colleagues found thrombocytopenia in 26% of fetuses with congenital toxoplasmosis studied by fetal-blood sampling [228].
Leukocyte abnormalities have been described in congenital toxoplasmosis. Eighteen percent of infants with generalized disease had eosinophilia [218]. Hohlfeld and colleagues found leukocytosis and eosinophilia in 7% and 9%, respectively, of infected fetuses [222]. Infected infants had lower levels of CD4 lymphocytes and a lower ratio of CD4 to CD8 lymphocytes than uninfected infants of mothers with gestational toxoplasmosis [229].
Congenital Bacterial Infection
Syphilis
Congenital syphilis caused by the spirochete Treponema pallidum was one of the first recognized perinatal infections. In the USA, risk factors for congenital syphilis include young maternal age, low socioeconomic status, parental drug use, sexual promiscuity, and inadequate prenatal care [230]. Unfortunately, syphilis is once again on the rise in America [217]. In the UK, low maternal infection rates were noted in the mid 1990s. Risk factors identified in the UK included being born abroad, non-white ethnicity, and living near London [231]. High rates of gestational syphilis have also been reported from Bolivia (4.3%), and aboriginal Australia (28%). Rates declined slightly in only 54 of 132 countries from 2012 to 2016; declines were substantive in only five of these countries [232] .
Clinical Manifestations
In endemic areas, the incidence of maternal syphilis infection in mothers of stillbirths is significantly greater than in mothers of live births. The probability of congenital syphilis and stillbirth is highest with secondary syphilis and early latent disease; the risk of disease is much lower in maternal late latent disease [233, 234]. Syphilis is a cause of stillbirth, nonimmune hydrops [233], and intrauterine growth retardation [234]. Many organs can be involved with congenital syphilis, and several clinical patterns of disease have been noted, including asymptomatic infection, maculopapular skin rash, mucoid and occasionally bloody rhinitis (“snuffles”), jaundice with hepatomegaly, monocytosis or lymphocytosis, hemolytic anemia, lytic bone lesions, nephritic syndrome, and neurosyphilis (which in newborns is often manifested only by a positive CSF VDRL) [234]. Congenital syphilis has been reviewed [230, 235].
Diagnosis
The diagnosis of congenital syphilis may require a high index of suspicion in the absence of obvious clinical stigmata of the disease. Maternal infection is often asymptomatic. Even if the characteristic chancres appear, they may be non-painful and hidden from sight. In one study of 155 women with syphilis during pregnancy, infection was asymptomatic in 121 (78%) [236]. Maternal screening serologies performed early in pregnancy will miss infection later in gestation. This is the basis for the recommendation that all pregnant women be screened for syphilis in the first trimester and again at the start of the third trimester. Women at high risk or who reside in high-prevalence areas should also be screened again at delivery [235]. High-risk women should also be screened at 28–32 weeks [233]. Placental abnormalities, such as the presence of plasma cells, may suggest the diagnosis of maternal infection. The Red Book®: 2018–2021 Report of the Committee on Infectious Diseases from the American Academy of Pediatrics notes that no newborn should be discharged from the hospital without determination of the mother’s serologic status for syphilis [217].
Diagnostic tests for the infant include X-ray examinations, tests for the organism, serologic tests, and, recently, PCR assays. Non-treponemal tests have long been used as screening tests for syphilis. The venereal disease research lab (VDRL) and rapid plasma reagin (RPR) identify antibody against cardiolipin. The RPR may be the more sensitive test for serum, but only the VDRL is recommended for detection in the CSF [227]. If the infant’s serum antibody titer is higher than the mother’s, a presumptive diagnosis of congenital infection is made, but it is important to use the same assay performed in the same laboratory. Problems with non-treponemal assays include a relatively high false-negative rate in early, latent, and tertiary syphilis, a potential for a false-negative reaction with very high titers of antibody (the “prozone phenomenon”), and false-positive results caused by acute processes such as hepatitis, lymphoma, viral infection, malaria, endocarditis, or connective tissue disease [233]. Chronic biologic false-positive reactions can be caused by drug abuse or systemic diseases such as chronic hepatitis or collagen vascular disease, especially systemic lupus erythematosus. Women with false-positive RPRs will almost always have negative specific treponemal tests (see below). Wharton jelly contamination can cause a false-positive non-treponemal antibody test from cord blood [217]. Testing cord-blood sera may be less sensitive than testing neonatal serum at 2 to 3 days of age [237]. Non-treponemal antibody titers decrease over time with successful treatment [217].
Specific treponemal antibody tests are also used; these include the fluorescent treponemal antibody absorption test (FTA-ABS) and the microhemagglutination T. pallidum (MHA-TP) test. These tests have a sensitivity of 75%–85% in early syphilis and 100% for later diagnosis [234]. Because these tests usually remain positive, even in treated patients, the presence of a positive test does not prove active disease and thus cannot be used to monitor response to therapy. Unless the disease is early, a negative test excludes the diagnosis. Because false-positive tests can be caused by infection with non-pallidum treponemes, treponemal antibody tests should be combined with a non-treponemal test [233]. Some institutions reverse the traditional order of testing, starting with treponemal testing such as the MHA-TP and then do RPRs only on those who screen positive. This “reverse testing algorithm” offers no obvious benefit to the clinician and sometimes results in confusion. Potentially exposed babies are still screened with RPR of serum, and VDRL of CSF.
Hematologic Features of Congenital Syphilis
Whitaker and Sartain described nine infants with congenital syphilis [238]. Eight had anemia. They observed that infants with the largest spleens had the most severe anemia, but splenectomy failed to resolve the anemia in one of these infants. Infants presenting in the first week of life have typical features of hemolysis; i.e., hyperbilirubinemia, increased reticulocyte counts, and peripheral blood-smear findings of polychromasia and increased numbers of nucleated RBCs. Hemolysis appeared to be most rapid in the first week of life. Hemoglobin levels generally became normal after 3 months of age. While hyperbilirubinemia is common, most infants have elevation of both the direct and indirect fractions of bilirubin.
Thrombocytopenia was found in 28% of South African infants with congenital syphilis [239]. Whitaker and Sartain documented thrombocytopenia in five of seven infants in whom platelet counts were performed [238]. Platelet counts as low as 17,000–20,000/μL were seen in both series. Thrombocytopenia is secondary to decreased survival, not to inadequate production of platelets. It is important to note that in some cases thrombocytopenia may be the only manifestation of congenital syphilis [230]. Neutrophilia with a left shift in the differential count was seen in 33% of Whitaker’s series. One infant had both vacuolization of the granulocytes and 5% peripheral blast forms that resolved in the first week of life [238]. Lymphocytosis or monocytosis and even leukemoid reaction may occur [240]. Rarely, a baby with congenital syphilis may develop paroxysmal cold hemoglobinuria [241]. Hemophagocytosis has also been described [242].
Congenital Viral Infections
Cytomegalovirus
Approximately 1% (range 0.2%–2.2%) of newborns are infected by cytomegalovirus (CMV) [243–245]. Maternal seropositivity rates vary with location and socioeconomic status. Females in developing countries or of lower socioeconomic status are more likely to become infected with CMV early in life and to be seropositive before pregnancy [245]. While most symptomatic intrauterine infections occur with primary infection in the mother [248], they may also occur in infants of seropositive mothers; in some studies, this rate was as high as 1%–2% [249].
Infants can also be infected during delivery from exposure to maternal secretions, and postnatally from breast milk of infected mothers, via blood transfusion, or from exposure to infected individuals [246]. Perinatally and postnatally acquired CMV infections are generally asymptomatic, but symptoms resembling congenital CMV may develop in premature neonates, particularly in those with a birth weight of less than 1,500 grams [247].
At birth, the majority of infants infected with CMV as fetuses are asymptomatic. In a study of 197 infants with congenital CMV, only 18% of infants showed signs of the disorder, and all of these were born to mothers with primary infection [248]. Severe generalized congenital CMV disease occurs in about half of symptomatic babies. CMV infection in the mother is more likely to have CNS sequelae when infection occurs in the first trimester [249]. Babies infected in utero with CMV may be born prematurely or have fetal growth restriction. Signs of symptomatic congenital CMV include petechiae, hepatomegaly, splenomegaly, and jaundice [250]. Neurologic problems are often prominent and include microcephaly, lethargy, poor suck, and seizures [250]. Intracerebral calcifications, generally periventricular, are a sequela of congenital infection [250]. Chorioretinitis is the most common ophthalmologic finding, but optic neuritis, cataracts (more common in rubella infection), and colobomas may be seen [250, 251]. Multiple other organs can be affected and infants may present with the classic “blueberry-muffin rash” due to dermal hematopoiesis [48]. Survival and/or long-term outcome of symptomatic infants is very poor.
Most infants with congenital CMV infection are asymptomatic at birth, but sequelae may appear in later life. Approximately 10%–15% of infants with congenital CMV infection who are asymptomatic at birth develop significant mental retardation or hearing deficit within the first 5 years of life [250].
Diagnosis
The demonstration of symptomatic CMV in infants of seropositive mothers indicates that maternal IgG cannot be used to exclude perinatal CMV infection [252, 253]. Diagnosis in the fetus can be challenging. While ultrasound abnormalities consistent with CMV combined with positive maternal serologies strongly support the diagnosis of intrauterine infection, a normal ultrasound does not exclude either congenital infection or sequelae [254]. IgG avidity assays are available; these are helpful in establishing the diagnosis of primary CMV infection in the mother because avidity is low for approximately 18–20 weeks after primary infection [254]. Early in gestation, infants are incapable of producing IgM [255]. Furthermore, even if the infected fetus does produce specific CMV IgM it may disappear before delivery [256]. The most sensitive techniques for prenatal diagnosis are viral culture from amniotic fluid and detection of CMV DNA via PCR, although this may be less sensitive in fetuses under 21 weeks’ gestation or less than 6 weeks from maternal infection [257) . Symptomatic congenital CMV may develop even though amniotic fluid PCR failed to demonstrate the presence of the virus, and the severity of congenital CMV disease in babies is not predictable by amniotic fluid viral load [258].
Postnatally, the diagnosis is now being established most commonly by salivary PCR, which is a rapid, sensitive, and non-invasive test. More traditional testing by cell culture of urine or other body fluids or tissues is time consuming, expensive, and requires expertise, and thus is being supplanted by PCR methodology [259, 260].
Hematologic Manifestations of Congenital CMV
Thrombocytopenia and petechiae are common in symptomatic infants [250]. Hohlfeld and colleagues found that 36% of infants with congenital CMV had thrombocytopenia, and that 38% of these had a platelet count below 50,000/μL [228]. In another study, 62/81 (77%) of babies with generalized CMV disease had a platelet count below 100,000/μL, and 42/81 (53%) had a platelet count less than 50,000/μL [252]. Several observations suggest there is accelerated platelet destruction with congenital CMV. Consumptive coagulopathy and thrombosis have been noted in infants with congenital CMV [261, 262]. Apparent immune thrombocytopenia following congenital CMV infection has been reported [263]. Hypersplenism may also contribute to the shortened platelet lifespan. However, human CMV infection can also impair megakaryocyte viability [264]. Hence, decreased platelet production may contribute to the thrombocytopenia. Thrombocytopenia, with or without petechiae, may persist for weeks to months in some affected babies. On rare occasions, petechiae may be the only sign of congenital CMV infection.
Hemolytic anemia is a common finding in infants with symptomatic CMV [250]. Erythroblastosis, polychromasia and abnormal RBC morphology may be seen in the peripheral blood of infants with congenital CMV [244, 262]. Contributing factors include RBC membrane damage and hypersplenism [265]. Autoimmune hemolytic anemia is a complication of infantile CMV [266]. CMV has been shown to infect hematopoietic precursors, and hence decreased erythrocyte production may play a role [267]. CMV may cause hydrops fetalis [268].
Leukocyte abnormalities are common. Atypical lymphocytosis can be seen [269]. Neutropenia following congenital CMV infection has been reported [270].
Rubella
Rubella is a member of the togavirus family. It has a worldwide distribution, but vaccination has dramatically decreased the prevalence of viral infection in the developed world. Maternal infection is usually confirmed serologically. However, epidemics still occur even in developed countries and an estimated 100,000 cases of congenital rubella syndrome occur annually [271]. Positive rubella-specific IgM is a good indication of a recent maternal infection, but false-positive and false-negative tests may be seen [272]. Maternal reinfection may occur, and while risk of fetal infection is small, estimated at <5%, and the risk of congenital defects is even smaller, it is not absent [273, 274]. The development of fetal embryopathic changes is highest with first trimester infection and decreases over the first two trimesters [272, 275].
Not all congenitally infected newborns are symptomatic, and defects, especially deafness, can develop over time. In most cases, however, infection in the newborn is suspected on clinical grounds. The common manifestations of congenital rubella syndrome include fetal growth restriction, hepatosplenomegaly, congenital heart disease (most commonly patent ductus arteriosus or pulmonary valvular stenosis), meningoencephalitis, mental retardation, bony lucencies on X-ray, sensorineural hearing loss, interstitial pneumonitis, and ophthalmologic findings, including cataracts, retinopathy, and/or congenital glaucoma [276]. These infants may also present with the blueberry-muffin rash of dermal erythropoiesis. Of these findings, the major defects (and the defects that most characterize congenital rubella syndrome) are heart defects, eye defects (cataracts, glaucoma, or microphthalmia), and deafness.
Diagnosis
Viral culture and PCR should be attempted; the virus is most readily detected in specimens from the pharynx or nasal swab specimens but it can also be found in conjunctival scrapings, cerebrospinal fluid, blood, or urine specimens [272, 276]. Congenital rubella is a chronic infection; viral shedding usually persists for months [272]. Virus may be cultured from the CNS of infants with encephalitis [276]. Rubella-specific IgM from the infant is a strong indicator of congenital rubella, but false-positive tests can occur with rheumatoid factor, parvovirus IgM, or heterophile antibodies [272]. Rubella-specific IgM persists for a longer time in the infant with congenital rubella syndrome [277]. Passive rubella IgG from the mother should decrease by about one dilution per month and be absent by 6–10 months; stable or increasing rubella-specific IgG in the baby over the course of several months usually indicates congenital infection [272]. PCR of amniotic fluid has been used to diagnose rubella in the fetus of infected mothers [276, 278].
Hematologic Manifestations of Congenital Rubella
Decreased platelet counts are seen in the congenital rubella syndrome (CRS) [276]. Petechiae and purpura are noted in 29%–100% of such infants [279, 280]. Cooper and colleagues reported that 17% of infants with congenital rubella had platelet counts below 20,000/μL [281]. Bone marrow studies from thrombocytopenic infants with congenital rubella showed decreased megakaryopoiesis with a shift to more juvenile megakaryocytes [280, 282]. Splenic sequestration may be a contributing factor. DIC has been reported [262]. Thrombocytopenia may be a marker of severe of congenital rubella syndrome [283].
Anemia is noted in less than 20% of babies with congenital rubella; it may be present at birth or develop over the first month [280, 281]. There are several features that suggest hemolysis. Peripheral blood smears show abnormal red-cell morphology and increased numbers of normoblasts. The reticulocyte count is increased. The bone marrow usually shows accelerated erythropoiesis with an increased erythroid:myeloid ratio [282]. Decreased RBC survival has been documented [280]. One report, however, described a transient bone marrow (BM) hypoplasia in an infant with congenital rubella [284]. Leukopenia and leukocytosis have been noted in patients with congenital rubella [280]. Lymphadenopathy has been noted in approximately 20% of patients. Hepatomegaly and/or splenomegaly are common in symptomatic infants [281].
Herpes Simplex
Herpes simplex virus (HSV), like all members of the herpesvirus family, establishes latency after acute infection and then may reactivate periodically, sometimes with symptoms and sometimes without. There are two distinct antigenic types of HSV. Although an old paradigm holds that HSV-1 usually infects the oral region while HSV-2 typically involves the genital region, there is much overlap in both the epidemiologic and clinical manifestations of the two viruses. Acquisition generally occurs through intimate contact. Seropositivity to HSV-2 parallels the onset of sexual activity [285]. Older serologic tests did not distinguish reliably between HSV-1 and HSV-2, but more recently approved assays are able to do so [286]. Unless the exposure was recent, a negative serologic test should exclude prior infection in the mother [287]. Recommendations for the diagnosis of neonatal HSV infection include surface swab specimen (mouth, nasopharynx, conjunctivae, and anus) HSV PCR, vesicular PCR, and CSF and blood PCR [288]. Cell culture may still be available in some places, but most institutions have replaced cell cultures with PCR testing. Most infants with congenital HSV are born to asymptomatic mothers [285]. Factors that increase the risk of neonatal infection include primary maternal infection during gestation, infection late in gestation (preventing the transmission of maternal HSV-specific antibody to the fetus), the use of fetal scalp electrodes, and prolonged rupture of membranes [285].
The incidence of perinatal HSV in the USA is approximately 11–33 per 100,000 live births [285]. Infection of the neonate can occur in utero, during delivery, or postnatally. Intrauterine transmission is uncommon but may cause signs and symptoms similar to those of other congenital infections, such as toxoplasmosis and CMV, or it may cause unusual skin manifestations that are not readily recognized as herpetic [289]. More commonly, the newborn is exposed to virus from maternal genital fluids during delivery [285]. Perinatal HSV usually presents in the first month of life, with two-thirds of cases presenting in the first week [290]. Infection may also occur with exposure to infected caregivers, including mothers, fathers, and other providers [285, 291]. Perinatal infections are classified as localized disease to the skin, eyes, and mucous membranes (often called “SEM” disease); encephalitis with or without SEM involvement; and disseminated disease [285]. Disseminated disease is often fatal and may involve the liver, lungs, heart, CNS, skin, and other organs.
Diagnosis
In the absence of skin vesicles, the diagnosis of disseminated neonatal HSV can be challenging. After 24 hours of age, swab specimens of conjunctivae, nasopharynx, and rectum should not be positive for HSV by either PCR or culture unless the neonate is infected. When skin lesions are present, the diagnosis can be confirmed by rupturing vesicles, scraping the base of the vesicles, and submitting the specimens to the laboratory for PCR testing (or viral culture, where available). Treatment should be instituted at the first suspicion of HSV disease. Cerebrospinal fluid cultures are often falsely negative, even in the presence of significant encephalitis, and therefore PCR is the gold standard for diagnosing CNS disease [288, 292]. Studies show a broad range of sensitivity for CSF PCR in HSV encephalitis [285]; acyclovir should be continued if there are other features strongly suggestive of HSV CNS disease, such as focal seizures, abnormalities localized to the temporal lobes on imaging studies, or paroxysmal lateralizing epileptiform discharges (“PLEDS”) on electroencephalography. Elevation of liver enzymes may be an early clue to the diagnosis of disseminated disease in febrile neonates [293].
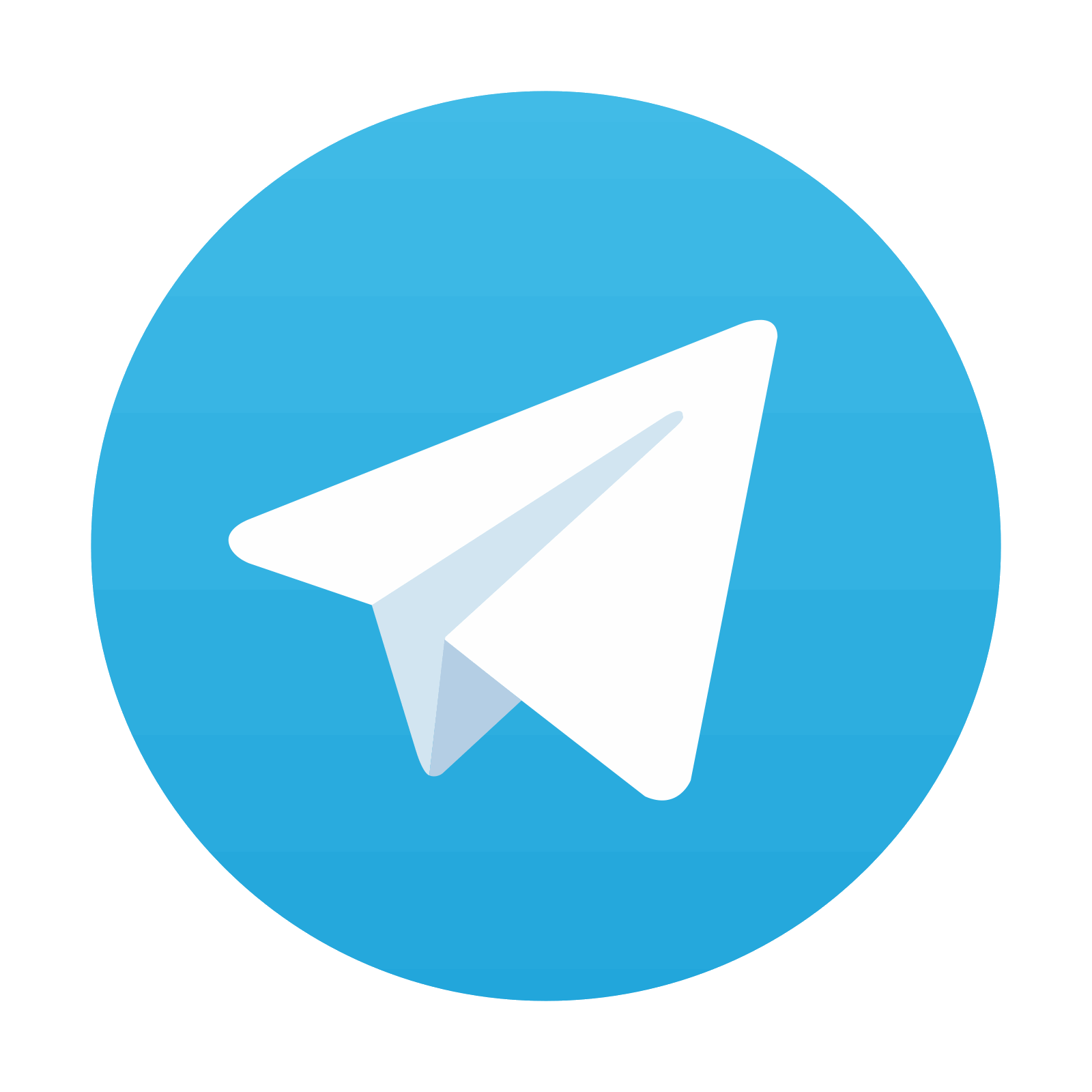
Stay updated, free articles. Join our Telegram channel
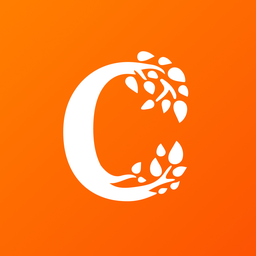
Full access? Get Clinical Tree
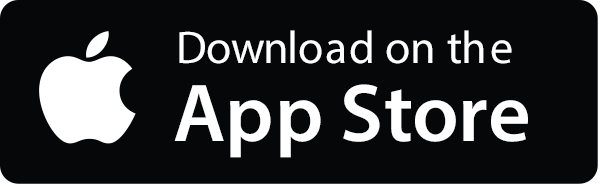
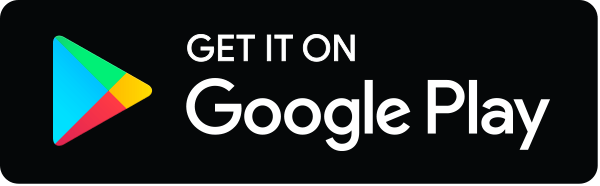
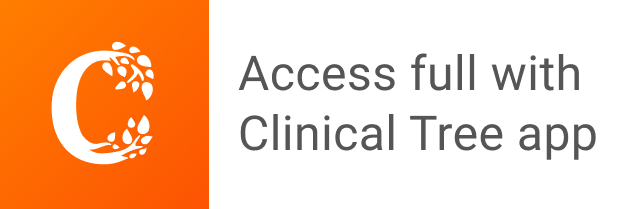