Abstract
Neonatal transfusion therapy requires an understanding of the dynamic interactions of the fetomaternal unit, the physiologic changes that accompany the transition from fetus to neonate to infant, and the underlying pathophysiology of different hematologic disorders. Guidelines for neonatal transfusions remain controversial, since most have been extrapolated from evidence in adults or based on small studies in neonates with marginal statistical validity. Compared to older children and adults, neonates have small total blood volumes but high blood volume per body weight. Because of the limited capacity to expand their blood volume to compensate for their rapid growth, many sick and/or premature infants require significant blood component support, especially within the first weeks of life. Immaturity of many organ systems predisposes them to metabolic derangements from blood products and their additive solutions, and to the infectious and immunomodulatory hazards of transfusion, such as transfusion-acquired CMV (TA-CMV) infection and transfusion-associated graft versus host disease (TA-GVHD). Therefore, component modifications are often required to compensate for the infant’s small blood volume, immunologic immaturity, and/or compromised organ function, and constitute the uniqueness of neonatal transfusion therapy.
Neonatal transfusion therapy requires an understanding of the dynamic interactions of the fetomaternal unit, the physiologic changes that accompany the transition from fetus to neonate to infant, and the underlying pathophysiology of different hematologic disorders. Guidelines for neonatal transfusions remain controversial, since most have been extrapolated from evidence in adults or based on small studies in neonates with marginal statistical validity. Compared to older children and adults, neonates have small total blood volumes but high blood volume per body weight. Because of the limited capacity to expand their blood volume to compensate for their rapid growth, many sick and/or premature infants require significant blood component support, especially within the first weeks of life. Immaturity of many organ systems predisposes them to metabolic derangements from blood products and their additive solutions, and to the infectious and immunomodulatory hazards of transfusion, such as transfusion-acquired CMV (TA-CMV) infection and transfusion-associated graft versus host disease (TA-GVHD). Therefore, component modifications are often required to compensate for the infant’s small blood volume, immunologic immaturity, and/or compromised organ function, and constitute the uniqueness of neonatal transfusion therapy.
Pretransfusion Testing
A sample of cord blood is often collected in all newborn infants at the time of delivery, but routine testing of cord blood for ABO group and Rh type is not necessary for healthy newborn infants unless the mother is Rh-negative and/or has a positive antibody screen [1]. For sick infants, ABO and Rh type should be determined on samples obtained from both mother and baby. Cord blood may be used for initial testing, but should be confirmed with an infant’s sample. The infant’s blood group is determined from the red blood cells (RBCs) alone, since the corresponding isoagglutinins anti-A and anti-B in the serum/plasma are usually weak or absent. Screening for atypical antibodies may be performed on maternal blood if available, or in the neonatal serum/plasma. A conventional cross-match is unnecessary if atypical antibodies are not demonstrable. Further compatibility testing during any one hospital course up to 4 months of age can be omitted for repeated small-volume transfusions since the formation of alloantibodies in the first 4 months of life is extremely rare [1]. However, this requires that the initial antibody screen is negative and all transfused RBCs are group O, Rh-negative, or ABO- and Rh-compatible. If the infant is supported with plasma and platelets, then passive acquisition of antibody may occur, and cross-matching is indicated, particularly for major surgical procedures, where large volumes of blood may be transfused [2]. If the antibody screen is positive, then serological investigation to identify the antibody is necessary. Full compatibility testing should be performed with appropriately selected blood, negative for the antigen(s) to which the antibody is directed.
Red Blood Cell Transfusions
Anemia in the neonatal period may be secondary to blood loss, hemolysis, or impaired production of RBCs. The usual postnatal decline in RBC mass that occurs in all newborns is more pronounced in preterm infants, resulting in hemoglobin levels that drop to 8 g/dL in VLBW infants (very low birth weight, 1,000–1,500 g), and to 7 g/dl in ELBW infants (extremely low birth weight, <1,000 g), by 4 to 8 weeks of age [3]. This phenomenon, termed anemia of prematurity (AOP), is primarily due to lower hemoglobin concentrations at birth, frequent blood sampling, low total blood volume to blood sampling ratio, increased risk for other comorbidities, and a diminished erythropoietic response [4]. In sick infants requiring intensive care, frequent blood sampling for laboratory tests and blood gas monitoring results in significant iatrogenic anemia and have been correlated directly to transfusion requirements [5]. Approximately 65%–90% of VLBW infants require multiple small volume RBC transfusions [6]. Controlling for the degree of prematurity and severity of illness, there is considerable variation in transfusion practice in different centers, which significantly impacts the number and volume of RBCs transfused. This indicates a large discretionary element in the utilization of RBC transfusions, and is not surprising given the paucity of convincing evidence based data to guide clinicians [7, 8].
The definition of “nonphysiologic” versus “physiologic” anemia in preterm neonates, and the hemoglobin levels at which to transfuse, is fraught with controversy. Tissue oxygen delivery is determined not only by the hemoglobin concentration but also by the proportion of fetal to adult hemoglobin, level of RBC 2,3-diphosphoglycerate (2,3-DPG), cardiac output, and arterial oxygen tension [3, 9]. Tachypnea, periodic breathing or apnea, tachycardia, poor feeding, poor growth, decreased activity, and anaerobic metabolism resulting in lactic acidosis all are considered to be indicators of significant anemia. However, many of these symptoms, signs, and tests are nonspecific and do not correlate well to hemoglobin levels or respond consistently to RBC transfusions [10]. Attempts to identify accurate indicators of peripheral oxygen delivery through the use of nonradioactive measures of circulating blood volume, and near-infrared spectroscopy to measure fractional oxygen extraction have had variable results. Neither method has proven to be an accurate predictor of transfusion, based at least in part on study design and technical aspects of the devices used [11, 12].
Risks of Transfusion Therapy
Advances in donor recruitment, blood screening, and processing have decreased the risks associated with blood transfusion. As part of patient blood management, current transfusion considerations and guidelines focus on reducing both transfusion number and donor exposures. Nevertheless, hematologic, immunologic, infectious, cardiovascular, and metabolic complications can occur. Many of these risks exist for transfusion recipients of any age, whereas others pose a greater threat to the neonatal recipient. These potential risks affect the choice and processing of blood products. Parents must be advised of the risks, benefits, and alternatives to transfusion, and informed consent should be documented in the medical record along with the indications for, and results of, the prescribed transfusion.
Indications
Many guidelines have been published regarding indications for RBC transfusion based on expert opinion from clinical experience rather than on actual statistically powered randomized controlled trials. Therefore, transfusion thresholds remain controversial because there are few studies which address the appropriateness of these transfusion triggers in neonates. Current guidelines for replacement transfusion therapy in neonates are given in Table 20.1 [2, 4]. Infants with significant cardiopulmonary disease generally require more aggressive RBC transfusion support, whereas infants receiving minimal cardiopulmonary support, with acceptable weight gain, and with minimal episodes of apnea and bradycardia, require less aggressive support [4, 6, 13]. Some have advocated the use of absolute reticulocyte counts in stable, growing infants with anemia of prematurity, proposing that an absolute reticulocyte count of >75–100 × 103/µL is a reliable predictor of a future rise in hemoglobin (Hb), and that RBC transfusion can be avoided in the absence of clinical signs of anemia and significant iatrogenic blood loss [6].
Table 20.1 Guidelines for red blood cell replacement for neonates
Clinical status | Target hematocrit |
---|---|
For severe cardiopulmonary disease (requiring mechanical ventilation with FiO2 >0.35) | >40%–45% |
For moderate cardiopulmonary disease | >30%–35% |
For major surgery | >30%–35% |
For infants with stable anemia with unexplained apnea/bradycardia, tachycardia, or poor growth | >20%–25% |
Restrictive versus Liberal RBC Transfusion Thresholds
Two studies attempted to address high versus low threshold transfusion guidelines based on level of respiratory support in ELBW and VLBW infants. Although very different in design and outcome, neither study clearly established an appropriate hemoglobin target. While the multi-institutional Canadian Premature Infants in Need of Transfusion (PINT) study demonstrated no advantage for liberal transfusion practices [14], the Bell study from Iowa suggested that restrictive transfusion was associated with more apneic episodes, intraparenchymal brain hemorrhage, and periventricular leukomalacia [15]. The discrepant results may be due to a greater Hb difference in restrictive/liberal transfusion groups (2.7 gm/dL versus 1.1 gm/dL), and a higher overall Hb value in the liberal transfusion group in the Bell study compared to the PINT study.
In contrast to short-term effects, long-term (average 12 years of age) neurodevelopmental measurements in the Bell study cohort showed reduced brain volumes for neonates transfused using liberal guidelines [16]. Meanwhile, previously enrolled ELBW infants from the PINT study were followed up at 18 to 21 months’ corrected age. Although liberal transfusion practices did not result in a statistically significant difference in death or the presence of cerebral palsy, severe cognitive delay (mental development index score [MDIC] <70), or severe visual or hearing impairment, post-hoc analysis with cognitive delay redefined (MDIC <85) showed a significant difference favoring the liberal threshold group [17].
More recently, a meta-analysis published in 2016 did not identify statistically significant differences in a range of harmful outcomes between neonates exposed to restrictive and liberal RBC transfusion practice [18]. It has been argued that a more liberal approach to RBC transfusion may be warranted, due to the decreasing infectious risks of RBC transfusions, the ability to decrease donor exposures using repeat RBC transfusions from the same donor, and the possible neuroprotective advantage of more liberal RBC transfusion practices. Further studies are needed to guide decisions for RBC transfusion support, given the unclear short- and long-term benefits and risks of liberal transfusion practices. The NICHD sponsored Transfusion of Premature (TOP) trial (NCT01702805) will determine whether higher hemoglobin thresholds for ELBW patients will result in improved neurodevelopmental outcomes.
RBC Preparations
RBCs are stored in one of several anticoagulant-preservative (AP) solutions to improve red cell viability and to extend storage time. All AP solutions contain citrate, phosphate, and dextrose (CPD), which function as an anticoagulant, a buffer and a source of RBC metabolic energy, respectively. The addition of mannitol and adenine to existing additive solutions has increased the shelf life of RBCs from 21 days (CPD) to 35 days (CPDA-1) and to 42 days for extended-storage AP solutions (Adsol® [AS-1], Nutricel® [AS-3], Optisol® [AS-5]) by stabilizing the RBC membrane and maintaining 2,3-DPG and adenosine triphoshate (ATP) within erythrocytes. RBC units collected in citrate–phosphate–dextrose–adenine (CPDA-1) usually have a hematocrit (Hct) of approximately 70%, whereas RBCs in newer extended-storage AP solutions have a Hct of 60% or less. Studies have shown that extended-storage preservative solutions are safe, and as efficacious as CPDA-1 RBCs in increasing the Hct in neonates receiving small-volume transfusions (10–15 mL/kg) [19–21]. However, there are no clinical studies that have confirmed or refuted the effect of an AP on metabolic abnormalities in massive transfusion (>20 mL/kg) for the neonate. Of particular concern is the potential for adenine-induced nephrotoxicity [20] and intolerable fluid shifts secondary to the diuretic effects of mannitol (present in AS-1, AS-5, and AS-7 but not AS-3)[19] in neonatal patients with limited blood volumes [2]. Therefore, some experts recommend avoiding RBCs stored in extended-storage media (AS-1, AS-3, AS-5) for large-volume transfusions until such data have been published.
All blood components must be filtered before transfusion. The standard 120–170 μm pore-size filter is adequate for red cells, plasma, and platelets. Microaggregate filters (10–20 μm pore size) can screen out 20–120 μm particles, which include clumped platelets, fibrin, and nonviable granulocytes that accumulate during storage. These filters are unnecessary when components undergo prestorage leukodepletion, which is recommended for recipients at risk for transfusion transmitted cytomegalovirus (TT-CMV) (see the subsection Cytomegalovirus under the section on Transfusion-Transmitted Infections on p. XXX). In addition to reducing the risk of TT-CMV, leukoreduction has dramatically decreased the incidence of febrile non-hemolytic transfusion reactions (FNHTRs) in RBC and PLT transfusions since being implemented, from 10% to approximately 0.1%–3% (0.2% for prestorage leukoreduction) [22, 23]. Leukocyte reduction has also been proven to reduce the incidence of HLA alloimmunization in adults [24].
Dose and Administration
There is little information on the optimal volume of RBCs to be transfused to correct anemia; however, most infants are transfused 10–15 mL/kg, depending upon their cardiovascular status. One small study indicated that transfusion of 20 mL/kg of packed red blood cells (PRBCs) results in a significantly greater rise in hematocrit in VLBW infants, as compared with transfusion of 10 ml/kg, without detrimental effects on pulmonary function, vital signs, or serum potassium; however, all infants in this study received furosemide immediately following transfusion [25]. Conclusions on the safety of transfusing 20 mL/kg RBCs has not yet been established. Formulae used to calculate volumes for transfusions are provided in Table 20.2.
|
|
|
|
* (CPDA-1RBCs Hct ~70%: whole blood withdrawn is replaced with packed RBCs.)
Warming small-volume RBC aliquots before transfusion is not necessary, particularly if the transfusion is given slowly over 2 to 3 hours. However, hypothermia can develop after massive transfusion unless the RBCs are first warmed. Controlled blood warming devices should be used for large-volume transfusions, particularly exchange and intra-operative transfusions. Blood components dispensed in syringes cannot be warmed in water baths because of the risk of contamination, but they may be warmed adequately when placed in warm-air incubators for 30 minutes before transfusion [2, 26]. Overheating, with resultant hemolysis, may occur when syringe aliquots are placed under radiant warmers or phototherapy lights [26]. When phototherapy is in progress, the blood component and tubing should be shielded from the UV light by using aluminum foil to prevent overheating and hemolysis. At the other extreme, freezing and lysis may occur if RBC products are stored in unmonitored refrigerators or freezers.
Whole-Blood Transfusion
A whole-blood (WB) unit contains approximately 450–500 mL of blood and 70 mL of AP solution. The shelf life of WB depends on the AP solution in which it is stored (i.e., 35 days in CPDA-1; 42 days in extended-storage media). Whole blood stored longer than 48 hours does not contain functional platelets or granulocytes, and coagulation factors (especially V and VIII) decrease throughout storage. Many blood centers rarely collect WB for allogeneic use because reconstituted WB, prepared by combining a unit of RBCs with an appropriate volume of compatible fresh frozen plasma (FFP), can be used for the same clinical effect. Whole blood or reconstituted WB is the product of choice in the setting of massive transfusion or acute blood loss, where restoration of oxygen-carrying capacity and blood volume are needed simultaneously. Primary indications for WB or reconstituted RB use in neonates include resuscitation of patients with acute blood loss in excess of 25% of their total blood volume (TBV) (i.e., ruptured vasa previa) [27], exchange transfusions, cardiopulmonary bypass, extracorporeal membrane oxygenation (ECMO), and continuous hemofiltration [28]. There is little advantage of WB in the emergency setting for the acute resuscitation of the neonate over packed RBCs and crystalloid or colloid solutions since other blood components replete in hemostatic elements may need to be subsequently transfused. When reconstituted WB is needed for large volume transfusion procedures, the neonate may be given plasma that is ABO compatible with the neonate’s RBCs, but RBCs that are compatible with maternal serum. This may mean that the ABO group of the RBC and plasma units may be different. An alternative used by some transfusion centers entails the use of low isohemagglutinin titer, group-O, Rh compatible WB, if available [4]. This decreases the risk of hemolysis in non-O patients transfused with the WB. The most recent AABB Standards set guidelines on the use of such low-titer WB [1].
The use of fresh WB (<48 hours old) to either prime the cardiopulmonary bypass (CPB) circuit and/or meet postoperative transfusion requirements have had conflicting results reported in randomized controlled trials. Transfusion of fresh WB has previously been associated with significantly less postoperative blood loss compared with the transfusion of multiple blood components separately following bypass surgery for complex congenital heart disease in children less than 2 years of age. This has been attributed to better platelet function in fresh WB, as measured by 30 minute and 3 hour postoperative platelet aggregation responses to ADP and epinephrine [29]. Friesen et al. also showed that the collection of fresh autologous WB (replacing with 5% albumin) prior to heparinization and reinfusion following CPB was associated with greater improvement of coagulation status after CPB in infants (>1 month old) with non-cyanotic heart disease undergoing noncomplex open heart surgery [30]. It is difficult to generalize the benefit in all infants because the CPB circuit was primed with a crystalloid solution, and cyanotic or complex patients may not tolerate the anemia and potential hemodynamic changes prior to CPB caused by this technique [30]. A subsequent prospective trial compared fresh WB to reconstituted WB for priming the CPB circuit in children <1 year of age (n = 200) undergoing open-heart surgery and found contrasting results. Infants who received reconstituted WB had a shorter stay in the intensive care unit than those who received fresh WB. There was no difference between the two groups in: early postoperative chest-tube output, transfusion requirements, levels of serum mediators of inflammation, or cardiac troponin I levels [31]. Unfortunately, there was no report of the age of the RBCs reported for the group who received reconstituted WB, making it difficult to attribute their findings to the age of RBCs used. Most recently, Gruenwald et al. prospectively compared the use of reconstituted fresh WB (RFWB), defined as <48 hours, versus standard blood component therapy (stored components used for reconstitution) both during CPB and within the first 24 hours postoperatively (n = 64) [32]. Lower chest tube loss in the first 24 hours postoperatively, shorter ventilation times, shorter hospital stay, and lower inotropic scores were reported for those neonates receiving RFWB versus those receiving standard blood component therapy [32].
Nonetheless, there currently exists no consensus within the United States on the use of fresh WB or RFWB for CPB pump priming or postoperative transfusion support in neonates with congenital heart disease. Decisions are often made by individual institutions based on inventory, the overall activity of cardiothoracic service, and the complexity of the patients treated. It should be noted that fresh WB (<48 hours old) is not universally available.
Donor Exposure
In the 1980s, 80%–90% of VLBW infants received multiple transfusions, often from different donors [33, 34]. Over the past two decades, a concerted effort to minimize the potential risks of transfusions by reducing the number of transfusions and donor exposures has been made through improvements in clinical care, decrease in laboratory blood draw volumes, noninvasive monitoring techniques, and the adoption of conservative transfusion guidelines. Using these methods, one tertiary-level nursery reported decreases in both the percentage of VLBW infants transfused, and number of RBC transfusions/VLBW infant from 88% to 65% and from 7.0 to 4.9 respectively [6].
Several studies have documented the safety of using RBCs stored in extended storage AP solutions until the expiration date of 35–42 days [35–37]. One or two preterm infants for whom multiple RBC transfusions are anticipated are assigned to dedicated units of freshly collected RBCs. Small aliquots are obtained repeatedly from the dedicated unit(s), using sterile connecting devices to transfer the RBCs into a separate bag, without compromising the integrity of the primary storage bag [38]. A closed-system filter-syringe set may alternatively be used in place of a transfer bag [39]. Previous studies have shown a 64% reduction in donor exposures in VLBW infants who received RBCs through a dedicated single-donor system [35]. Furthermore, multiple prospective studies have also shown that CPDA-1 and AS-3-preserved single-donor split RBC packs are safe for use in neonatal small-volume transfusions after 35 days and 42 days of storage respectively. No clinically significant changes in post-transfusion pH, ionized calcium, and potassium levels, and comparable hematocrit increments to fresh and or washed RBC products were demonstrated [35, 40, 41]. Many limited-donor programs report the reduction of donor exposures to approximately 2.0 per VLBW infant, with those neonates born before 28 weeks’ gestation and those between 28 and 31 weeks with IUGR at highest risk of needing more than one donor [42]. To minimize blood waste without compromising the goal of limiting donor exposure, many have devised models to predict each infant’s transfusion requirements, whereby those infants predicted to have high transfusion requirements are assigned to receive blood from a dedicated RBC unit, while other infants are assigned to receive blood from a unit that is shared among as many as four similar infants [43].
One prospective study has been conducted in premature infants to evaluate whether fresh RBCs (≤7 days) decreased morbidity and mortality in VLBW infants compared with standard RBCs. In the Age of Red Blood Cells in Premature Infants (ARIPI) trial conducted in Canada, 188 infants provided with fresh RBC transfusions (mean age of transfused RBCs 5.1 days, SD 2.0 days) did not demonstrate an improvement in a composite outcome measure of major neonatal morbidities (necrotizing enterocolitis [NEC], intraventricular hemorrhage [IVH], bronchopulmonary dysplasia [BPD], and retinopathy of prematurity [ROP]) or death at 30 and 90 days compared with the 189 infants who received standard RBC products (mean age of transfused RBCs 14.6 days, SD 8.3 days) despite having 60% more donor exposures [44]. Although an unblinded randomized control trial suggested an advantage in clinical outcomes in neonates with congenital heart disease receiving reconstituted fresh WB defined as less than 48 hours old when dispensed for cardiopulmonary bypass pump priming and postoperative transfusion support [32], the role of fresh RBCs for routine transfusion support in premature infants has not shown a clear benefit. ARIPI has subsequently been criticized for insufficient separation of mean RBC storage time between its two cohorts (<2 weeks) and for inadequately addressing the issue of storage lesion in the oldest additive solution units (35–42 days). The use of a relatively liberal transfusion strategy may also have contributed to better clinical outcomes than would be expected with restrictive practices [45]. Although the saline–adenine–glucose–mannitol (SAGM) additive solution used in the study is widely available in Canada and Europe, its restricted distribution in other regions may limit the relevance of its conclusions in countries like the United States where other types of additive solutions are utilized. However, based on the results of this and other recent randomized clinical trials in older children and adults such as ABLE [46], RECESS [47], TOTAL [48], and INFORM [49], recent guidelines for neonatal transfusion do not recommend limiting the age of transfused RBCs to <10 days [50, 51].
Directed (Including Parental) Donor Transfusions
Directed donations from first- and second-degree relatives rather than from voluntary blood donors are perceived by the lay public to have a lower risk of transmitting viral infections, although there are no scientific data to support this contention. One study showed that although biological parents were interested in donating for their infants, many were found to be ineligible for serological and medical reasons. However, those eligible were able to supply all small-volume RBC transfusions for their infants using a single-donor system in which RBCs were stored in AS-3 for 42 days or less [52, 53]. A retrospective review at a pediatric institution found that parental donors had higher rates of infectious disease testing positivity than community donors [54].
The transfusion of blood from biologic parents poses unique immunologic and serologic risks to the neonate. TA-GVHD is a well-recognized complication of the use of familial blood, therefore all blood components obtained from blood relatives should be irradiated before transfusion. Maternal plasma may contain antibodies directed against paternal red cell, leukocyte, and platelet antigens that are also expressed on neonatal cells. Anti-leukocyte and anti-platelet antibodies have been found in 16% and 12% of mothers, respectively [55]. Exposing the infant to these antibodies within maternal blood components can potentially result in hemolysis, thrombocytopenia, or transfusion-related acute lung injury (TRALI). Therefore, maternal RBCs and platelets should be washed or plasma-reduced prior to transfusion. Conversely, paternal blood products are a poor choice in neonates with immune mediated hemolysis (hemolytic disease of the fetus and newborn, or HDFN) or neonatal alloimmune thrombocytopenia (NAIT) because the transfused paternal cells express the antigens to which the neonate has passively acquired antibodies from the mother. Therefore, fathers and paternal blood relatives should not serve as donors for blood components containing cellular elements unless maternal serum is shown to lack lymphocytotoxic antibodies [4, 52].
Given these concerns, when parental-directed donation is considered for an infant, the following recommendation should be reviewed with families and the provider [56]:
All parental cellular blood components must be irradiated before transfusion to the neonate to prevent TA-GVHD.
If maternal RBCs or platelets are transfused, they should be given as washed cells or should be plasma reduced and irradiated.
Fathers are not recommended as RBC donors for their newborns.
Fathers should not donate granulocytes or platelets to their infants unless maternal serum is shown to lack lymphocytotoxic antibodies.
Recombinant Erythropoietin
Despite the fact that over 30 clinical studies have been performed to evaluate the efficacy and safety of recombinant human erythropoietin (rHuEpo) in neonates, there currently exists no clear consensus as to whether its use minimizes the need for blood transfusions without risk to the neonate. Reasons for the lack of consensus are multifactorial, including limitations in study designs; variation in infant demographics, transfusion practices, supportive care, and blood sampling techniques between studies, and the difficulty assessing the clinical impact of results [57].
Early randomized controlled trials demonstrated that most VLBW infants given rHuEPO received fewer and lower-volume RBC transfusion during the study period, with maximum benefit being seen in larger, more stable preterm infants [58–60]; however, conflicting results were reported for rHuEPO benefit in ELBW infants [61, 62]. Early (<8 days of age) and late (≥8 days of age) rHuEPO treatment protocols subsequently were designed to prevent and treat AOP respectively, by decreasing RBC transfusions. Although both regimens have been shown to reduce RBC transfusion, it remains uncertain whether the absolute reduction in transfusion volume achieved is clinically significant without preventing or decreasing donor exposures, in an era of single-donor, dedicated RBC unit transfusion practices [63].
The Cochrane Collaboration carried out three systematic reviews on the use of rHuEPO in neonates [64–66]. Their meta-analyses of both early and late rHuEPO use showed no clear benefit in terms of significantly decreasing donor exposures, nor was there any effect on incidence of comorbidities. Although the number and volume of RBC transfusions per infant were decreased, the clinical impact of these results was trivial (i.e., <1 transfusion/infant, 7 mL/kg of RBCs respectively in late rHuEPO-exposed infants) [64]. RBC transfusion was not avoided in early or late rHuEPO-exposed infants, when RBCs transfused prior to study entry were considered. Furthermore, a statistically significant increased risk of ROP (> grade 3) was noted in neonates who received early rHuEPO therapy [66]. rHuEPO alone exerts only modest effects on transfusion burden, and so therefore should be used cautiously in light of the risk of ROP. Its role for reducing transfusions in preterm infants in conjunction with methods to reduce iatrogenic blood loss, conservative transfusion practices, and autologous transfusion, remains to be determined. The role of rHuEPO as a neuroprotectant has been investigated by several groups; secondary analyses of these studies for transfusion may prove the additional information that will support its use in infant patient blood management [67].
Autologous Transfusion
Autologous transfusion in an infant can occur by collection, storage, and re-infusion of autologous cord blood (ACB) or by delaying cord clamping [68]. The placenta contains 75–125 ml of blood at birth depending on the gestational age of the infant. This serves as a substantial volume of fetal blood, and could be an ideal source for autologous RBCs for the neonate, eliminating the potential risks of transfusion transmitted diseases and TA-GVHD. However, high rates of bacterial contamination (15.8%) [69] and small collection volumes from infants less than 1,000 g have raised concerns as to whether such preparations are beneficial in ensuring safe care to neonates.
More recently, lower rates of bacterial contamination in stored ACB units (no contamination from ACB units derived from cesarean deliveries), acceptable volume yields, equivalent efficacy/safety of ACB-derived RBCs to allogeneic RBCs, and significant reduction of neonates with birth weight between 1,000 g and 2,500 g requiring allogeneic RBCs have increased interest in this blood product [70, 71]. Nonetheless, protocols for ensuring proper collection without bacterial contamination and adequate anticoagulation are still being refined; additional large, randomized controlled trials are needed to validate the safety, efficacy, and usefulness of this process [72, 73].
Delayed umbilical cord clamping (30 to 120 seconds) of premature infants has been reported as a successful variation on autologous transfusion. This simple maneuver has been shown to significantly increase RBC mass and circulating blood volume during the first 24 hours of life, while decreasing the immediate need of blood transfusions and the incidence of intraventricular hemorrhage in the preterm infant [74–76].
One limitation with delayed cord clamping is the required delay of 30 seconds or more in neonatal resuscitation during a VLBW delivery. As an alternative, “milking” or “stripping” the cord has been proposed. This is done by holding the placental end of the umbilical cord, and gently moving blood within the umbilical vessels toward the neonate. This “stripping” is performed one to four times prior to clamping and cutting the cord. In a randomized controlled trial of 40 VLBW infants (stripping versus immediate clamping), Hosono et al. reported that those infants in the stripped group had higher hemoglobin values and blood pressures at NICU admission, shorter duration of ventilation, lower odds of requiring a RBC transfusion, and lower odds of developing an IVH [77]. Subsequently, Rabe et al. compared delayed cord clamping versus cord stripping in a randomized trial (n = 58) and found no differences between the two groups in hemoglobin values, number of RBC transfusions, or morbidities [78]. A Cochrane review in 2012 showed that delayed cord clamping up to 180 seconds or umbilical cord milking versus immediate cord clamping resulted in 39% fewer transfusions for anemia, 41% fewer patients with IVH and 38% fewer patients with NEC [79, 80]. Similarly, a randomized clinical trial in 2017 found that delayed cord clamping reduced anemia at 8 and 12 months in infants at high risk for iron deficiency [81]. The American College of Obstetricians and Gynecologists currently recommends a delay in umbilical cord clamping in vigorous term and preterm infants for at least 30–60 seconds after birth [82].
Exchange Transfusion
Neonatal exchange transfusion is the replacement of the majority of RBC mass and plasma with compatible RBCs and plasma from one or more donors. The amount of blood exchanged generally is expressed in relation to the recipient’s blood volume (e.g., as a single or double-volume exchange, or a partial exchange).
Indications
The majority of exchange transfusions are performed for the treatment of hemolytic disease of the fetus and newborn (HDFN). Exchange transfusion procedures are frequently used in newborns with severe hyperbilirubinemia from other causes in order to prevent kernicterus and other toxicity [2]. Although not tested in RCTs, the procedure has also been used successfully, albeit infrequently, to remove exogenous (drugs) or endogenous (metabolic) toxins [83, 84], treat severe autoimmune hemolytic anemia [85, 86], correct life-threatening hyperkalemia [87, 88], and to treat neonatal sepsis unresponsive to other therapy [89].
When performed for HDFN, exchange transfusions correct anemia, replace the infant’s antibody coated RBCs with antigen-negative RBCs, remove free maternal antibody in the plasma, and decrease bilirubin levels. The American Academy of Pediatrics has established exchange therapy criteria for infants of ≥35 weeks’ gestation based on a nomogram incorporating post-natal age and total serum bilirubin (TSB) levels. Prematurity, hypoxemia, acidosis, hypothermia, and sepsis predispose to kernicterus at lower levels of bilirubin, and prompt earlier intervention.
A number of studies have been published on the use of intravenous immunoglobulin (IVIg, 0.5–1 gm/kg) as adjuvant treatment for Rhesus and ABO HDFN, and have demonstrated a reduction in the duration of phototherapy and the need for exchange transfusion [90–92]. The most recent Cochrane Collaboration meta-analysis, which included 658 infants in 9 studies, affirmed that IVIg administration can significantly reduce the need for exchange transfusion in term infants with Rhesus and ABO HDFN, although the authors could not provide even a weak recommendation for the use of IVIg based on the lack of demonstrable benefit in the least biased studies and overall low quality of the evidence [93]. However, in light of the potential for serious adverse outcomes in 5% of infants following exchange therapy, IVIg may be considered when serum bilirubin levels continue to rise despite aggressive phototherapy or when the bilirubin level is within 2–3 mg/dL of the exchange level [94].
The efficiency of exchange transfusion diminishes exponentially as the procedure continues. For this reason, a double-volume exchange is preferred for most procedures, as little is gained by exceeding two blood volumes. The kinetics of exchange are very similar, regardless of whether a continuous (simultaneous withdrawal and replacement) or discontinuous technique (alternating withdrawal and replacement) is used. The effectiveness of exchange transfusion varies with the component being removed, and is highest for RBCs. A double-volume exchange transfusion results in removal of approximately 85% of the neonate’s RBCs but only 25%–45% of bilirubin and/or maternal alloantibody. This is because of a slowly equilibrating tissue-bound pool for bilirubin, antibody, and similar substances. Repeat exchange transfusions are often needed in infants with severe HDFN because of rebound of bilirubin following the initial procedure, resulting from persistent maternal antibody destruction of sensitized infant RBCs and equilibration of extravascular and intravascular bilirubin. The use of albumin before exchange transfusion in an effort to mobilize tissue bilirubin has not been definitively demonstrated to improve efficiency of bilirubin removal [95].
Component Preparation
Either stored WB if available or reconstituted WB can be used for neonatal exchange transfusions. RBCs chosen for the exchange should be fresh (preferably < 7 days). If available, CPDA-1 units may be preferentially selected over additive solution units because the safety of extended storage media has not been amply studied for neonatal large-volume transfusions. If only older CPDA units or additive solution units are available, the RBC units may be volume reduced or washed. All components should be CMV risk reduced, irradiated, and sickle-negative, with final hematocrit of 40%–50% [4]. If the delivery of an infant with severe HDFN is anticipated, then group O Rh-negative blood cross-matched against the mother’s plasma may be prepared prior to birth. Blood prepared after delivery should be negative for the implicated antigen(s) and may be crossmatched against a maternal or neonatal sample. In ABO HDFN, the blood must be group O and Rh-compatible between mother and infant. If group O whole blood is selected, the products should have a low isoagglutinin titer. Group O RBCs are often reconstituted with AB plasma to ensure that no isoagglutinins are present, although this practice results in two donor exposures per exchange transfusion. Blood prepared for exchange transfusion for nonimmune indications such as nonimmune hyperbilirubinemia, drug overdose, and sepsis, are cross-matched against the infant only.
Administration
The volume needed for administering a double-volume exchange can be calculated using the formula in Table 20.2. The blood is warmed through a temperature-controlled in-line blood warmer, and the exchange transfusion is either performed by the traditional push–pull method using a single vascular access (typically the umbilical vein), or by isovolumetric techniques utilizing two access sites for simultaneous removal of the infant’s blood and administration of replacement blood products [96]. Aliquots of 5–20 mL with a maximum of 5 mL/kg are withdrawn or infused in the discontinuous method, at a rate not exceeding 5 mL/kg every 3 minutes to avoid rapid fluctuations in intracranial pressure [97]. When an isovolumetric exchange is being done, volumes to be removed/reinfused should not exceed 2 mL/kg per minute. The duration of the exchange is usually 1 to 2 hours.
Complications
Potential complications of exchange transfusion include hypocalcemia, hyper- and hypoglycemia, hyperkalemia, dilutional thrombocytopenia and coagulopathy, umbilical vein/artery thrombosis, NEC, and infection. Previous estimates on the risk of death or permanent serious sequelae were relatively high for neonates, particularly those who were premature and/or those with underlying illnesses. In a retrospective study examining adverse events among 106 neonates undergoing 140 exchange transfusions performed between 1980 and 1995, overall mortality was 2%, and 8% in those infants classified as “ill.” Death or serious complications were noted in 12% of sick neonates, but less than 1% for healthy infants (defined as only having hyperbilirubinemia) [89].
More recent reviews show fewer serious complications. A recent retrospective review of 55 neonates undergoing 66 exchange transfusions between 1992 and 2002, reported the majority of adverse events were asymptomatic laboratory abnormalities, including thrombocytopenia (44%), hypocalcemia (29%), and metabolic acidosis (24%) [98]. Adverse events were more frequent in exchanges performed on preterm infants with gestational age < 32 weeks or infants with other significant comorbidities, and when umbilical catheters were used versus other methods of central venous access. There was a single death reported in a severely ill infant; however, it was unclear if this was directly related to exchange transfusion [98]. These improved rates of serious adverse events have been confirmed in other reports [99]. Considering the declining rate of exchange transfusions in the 21st century, current mortality rates range from 0.3% in term neonates up to 10% in preterm neonates [100, 101]. Regardless, the potential risk of adverse events from exchange transfusion needs to be balanced against the risk of bilirubin encephalopathy in ill infants.
Partial Exchange Transfusion
Partial exchange transfusion (PET) is used to correct severe anemia without the risk of fluid overload and heart failure in critically ill hydropic infants, but is more commonly used to decrease the Hct in neonates with polycythemia-hyperviscosity syndrome. This syndrome is diagnosed in infants with a Hct above 65%–70% and with symptoms attributed to polycythemia, which include hypoglycemia, tachypnea, congestive heart failure, hypotonia, tremors, seizures, renal insufficiency, and/or NEC [102]. Reducing the Hct to approximately 55% causes rapid amelioration of the clinical manifestations of polycythemia and is associated with reversal of cerebral blood-flow abnormalities in symptomatic infants. The long-term benefit of early PET for neonatal polycythemia is questionable. In a long-term follow up study of 93 polycythemic infants randomized to receive either PET or supportive care, fewer neurologic abnormalities and fine-motor delays were noted at 2 years, but only limited benefits were seen at 7 years for the infants who received PET [103, 104]. Common indications include a Hct ≥70%, or a Hct between 65% and 70% in a symptomatic infant. Use of isotonic crystalloid replacement solutions through peripheral vessels rather than the umbilical vein is preferred in most instances because it has been found to be as effective as 5% albumin and plasma for replacement [105]. The technique of partial exchange transfusion is similar to that used in larger volume exchange [106]. Exchange volume calculations are listed in Table 20.2. More recent publications have focused on continuous exchange as an alternative to the traditional push–pull methods [107].
Intrauterine Fetal Transfusion
Intrauterine transfusion (IUT) has been used for the correction of critical anemia resulting from HDFN, fetal parvovirus B19 infection, twin-to-twin transfusion, fetomaternal hemorrhage, and homozygous alpha-thalassemia. Fetal transfusions may be administered by intraperitoneal, or intravascular via cordocentesis or intrahepatic venous puncture [108, 109]. Intraperitoneal transfusion (IPT) has been largely replaced by direct intravascular transfusion (IVT), since IVT circumvents the problem of poor absorption of RBCs from the peritoneal cavity of severely hydropic fetuses, and also allows precise diagnostic evaluation of the fetal status by means of fetal blood sampling. IPT may be necessary when intravascular access is difficult, due to narrow umbilical vessels (gestational age, GA <20 weeks) or increased fetal size. Furthermore, IPT can also be used in conjunction with IVT to prolong the interval between procedures and produce a more stable fetal Hct [108, 109]. A recent study indicates that IVIg may be an effective non-invasive alternative to IUT prior to 20 weeks’ gestation [110].
Intrauterine transfusions are generally performed when the fetal middle cerebral artery peak systolic velocity (MCA-PSV) exceeds 1.5 multiples of the median (MoM) for gestational age or there is evidence of hydrops suggestive of moderate to severe anemia [111]. The usual transfusion goal is a fetal Hct of 40%–45%, not to exceed a fourfold increase in Hct so as to avoid acute changes in blood viscosity [4]. Hct levels fall by approximately 1% per day, but can decrease more rapidly in fetuses with severe HDFN. This necessitates repeat transfusion every 21–28 days or more frequently (i.e., 7–14 days) for fetuses with more brisk hemolysis until suppression of fetal erythropoiesis occurs [112]. Overall survival for hydropic fetuses undergoing IUTs is approximately 78%–90%, with more severely hydropic fetuses having lower survival rates (55%) [113]. The perinatal loss rate is approximately 1%–3%. Risk factors associated with procedure-related fetal death include very low pretransfusion fetal Hct, large increases in post-transfusion Hct (> fourfold), increases in umbilical venous pressure during IUT, umbilical arterial puncture, and transamniotic cord needling [113–115]. Fresh (<7 days) CPD(A), CMV risk-reduced, irradiated blood is recommended for all IUTs. Older CPD(A) units or extended storage RBC units may be volume reduced or washed prior to use. The blood should be cross-match compatible with maternal serum, and antigen-negative for the offending antibody (or antibodies) in cases of HDFN, warmed to physiologic body temperature, and packed to a Hct of 75%–85% in the appropriate volume as calculated using formulas in Table 20.2.
Intrauterine transfusions are usually initiated at 20 weeks’ gestation and continued until 34–35 weeks’ gestation with delivery at 37–38 weeks, depending on disease severity. Infants with severe HDFN, who receive multiple IUTs often require less phototherapy and fewer exchange transfusions in the neonatal period [116]. After several IUTs, suppression of erythropoiesis is common, rendering these infants virtually devoid of reticulocytes, with their red cell mass derived almost entirely from donor RBCs [108]. This results in a more dramatic nadir period in which the majority of transfused infants may need several RBC transfusions within the first 3 months of life [117]. Meticulous communication between the NICU and transfusion center is imperative as misleading initial blood grouping and false-negative DAT may occur in the heavily intrauterine transfused infant.
Intrauterine platelet transfusions have been used to treat severely thrombocytopenic fetuses with neonatal alloimmune thrombocytopenia (NAIT) to prevent antenatal intracranial hemorrhage [118, 119]. Predictors of disease severity include a maternal history of prior affected pregnancies and fetal/neonatal intracranial hemorrhage occurring at an early gestational age. Mothers may be treated with steroids and IVIg based on risk stratification. Repeated transfusions at weekly intervals often are necessary because of the short survival time of transfused platelets. Irradiated washed maternal platelets may be used for single transfusions, but for repeated transfusions, a panel of selected platelet antigen-negative donors should be recruited. All products should be CMV risk-reduced and irradiated.
Platelet Transfusions
Neonatal thrombocytopenia, defined as a platelet count <150 × 109/L, complicates 22%–35% of all NICU admissions [120], and results from decreased platelet production, increased peripheral destruction, or a combination of these two processes, as typically seen in sick infants. Neonates have different risks of bleeding given an equivalent degree of thrombocytopenia. For example, thrombocytopenic neonates with IUGR have a relatively low risk of major hemorrhage whereas those with sepsis or NEC have an intermediate risk, and those with neonatal alloimmune thrombocytopenia (NAIT) have a high risk as demonstrated by a 10%–20% incidence of intracranial hemorrhage (ICH). Differences in platelet function, concurrent coagulopathy, and immunological factors are likely causes for these discrepancies.
Indications and Transfusion Thresholds
Quantitative and qualitative platelet disorders may cause significant bleeding with resulting morbidity, the most serious being ICH. One randomized controlled trial addressing whether platelet transfusions reduce major bleeding in neonates showed no difference in the incidence of new ICHs or extension of existing hemorrhages between infants given prophylactic platelet transfusions to maintain platelet counts of at least 150 × 109/L or to control infants who were transfused to maintain platelet counts greater than 50 × 109/L without evidence of bleeding [121]. However, this study did not address bleeding risk or transfusion benefit for neonates with platelet counts below 50 × 109/L.
In a cross-sectional observational study of neonatal outcomes with severe thrombocytopenia, Stanworth et al. failed to show a clear relationship between nadir platelet count/degree of thrombocytopenia and major hemorrhage (IVH, pulmonary, intra-abdominal, hematuria) [122]. In the 169 neonates studied with severe thrombocytopenia (platelet count nadir <60,000/µL), 154 (91%) did not experience major hemorrhage. Of the 15 (9%) that experienced major hemorrhage, 12 patients (80%) had a platelet count nadir before the hemorrhage of greater than 20,000/µL [122], and follow-up analysis revealed that thrombocytopenia alone was not a strong indicator of bleeding risk, whereas gestational age (<34 weeks), early onset of postnatal thrombocytopenia (<10 days after birth), and coexistence of NEC were strong clinical risk factors for bleeding in this population [123]. Retrospective studies have also failed to establish a link between the severity of thrombocytopenia and risk of IVH [124] across both liberal and restrictive transfusion practices [125, 126]. A recent retrospective study of VLBW infants demonstrated that a restrictive platelet transfusion protocol using platelet count thresholds of 25,000/µL in clinically stable neonates and 50,000/µL in clinically unstable neonates, extremely premature neonates within the first week of life, or neonates at high risk of major bleeding was not associated with an increased risk for IVH compared with a more aggressive transfusion protocol [125].
Platelets for Neonatal Transfusion Study 2 (PlaNet 2) (ISRCTN 87736839), an RCT in the UK, Ireland, and the Netherlands comparing prophylactic platelet transfusion thresholds of 25,000/µL and 50,000/µL in terms of mortality and major bleeding complications in 660 premature infants (less than 34 weeks), reported results in 2019 [127]. Infants randomized to the higher transfusion threshold had higher occurrence of a new major bleeding episode or death than infants randomized to the lower threshold (26% versus 19%, p = 0.02) [127]. The authors are unsure of the etiology but suggest that cytokines, inflammatory mediators, fluid shifts or adult donor platelets interacting with the neonatal coagulation milieu may play a role. Of note, the neonates studied had few, if any, risk factors for bleeding, and represented a small percent (660 of 3731) of infants assessed for eligibility.
Nevertheless, in their recent retrospective review, Sparger and colleagues found that a majority of neonatal platelet transfusions in the US continue to be administered for pre-transfusion platelet counts of ≥50,000/µL in VLBW infants despite evidence that platelet transfusion has little appreciable impact on the risk of IVH after adjustment for underlying clinical variables [128].
Antibody-mediated perinatal thrombocytopenia may be secondary to transplacentally transmitted maternal antibodies directed against both maternal and neonatal platelet antigens (neonatal ITP), or against paternally inherited fetal/neonatal platelet antigen (NAIT). Each etiology carries a distinct degree of thrombocytopenia and distinct bleeding risk. Incidences of severe thrombocytopenia (platelet count <20 × 109/L) and ICH in infants born with neonatal ITP occurs in approximately 1%–5% and 1% respectively, with the majority of ICH occurring in infants having platelet count nadirs below 10 × 109/L [129, 130]. Platelet transfusions of any antigen type and/or maternal platelets are relatively ineffective in infants with neonatal ITP [131], and are advocated only in life-threatening bleeding emergencies, although thrombocytopenia usually responds to IVIg and/or corticosteroids [129, 132]. In contrast to neonatal ITP, NAIT carries a substantial risk of ICH, half occurring in utero before 28 weeks’ gestation [132, 133]. A 7 year prospective study reported an overall incidence of ICH in neonates with NAIT of 14%, and indicated that individuals with fetomaternal incompatibility at human platelet antigen-5b (HPA-5b) may bleed at higher platelet counts [134]. For this reason, the threshold for platelet transfusion for neonates with NAIT remains unclear. A generally accepted standard platelet threshold of 25–30 × 109/L for neonates without other bleeding risk factors, and a higher trigger (50–100 × 109/L) for preterm neonates, clinically unstable neonates, and neonates with NAIT [132, 135]. Hemorrhage associated with acquired (i.e., ECMO, CPB, antiplatelet medications, uremia) or congenital qualitative platelet abnormalities (i.e., Bernard–Soulier syndrome) are treated with platelet transfusions even if the platelet count is within normal range.
Preparations
A standard platelet unit from a single whole blood donation contains 5.5 × 1010 platelets in 50–70 mL of plasma. Apheresis platelets, also called single-donor platelets (SDPs), contain a minimum of 3.0 × 1011 platelets in approximately 250 mL of plasma (range: 200 to 400 mL). Apheresis platelets can be split into multiple small aliquots by means of a sterile connecting device in the same fashion as RBCs, offering the advantage of limiting donor exposures. Each whole blood unit (or whole blood equivalent of an apheresis unit) may be volume reduced to 15–20 mL for patients with significant fluid restrictions, but this process is associated with significant platelet loss of 15%–35% and may affect platelet function adversely. The shelf life of WB-derived and apheresis platelets ranges from 5 to 7 days, depending on additional testing for bacterial contamination; volume-reduced platelets expire 4 hours after manipulation. Table 20.3 lists various indications for irradiation of cellular blood products (RBCs, platelets, granulocytes) in the neonatal population, although many institutions elect to provide universally irradiated cellular blood components to all infants under the age of 12 months (or older) due to delayed presentation/diagnosis of congenital immunodeficiency syndromes.
Table 20.3 Indications for administering irradiated blood components to neonates*
|
Platelets express intrinsic ABO antigens, but not Rh antigens. Whenever possible, the platelets should be ABO- and Rh- compatible because intravascular hemolysis following transfusion of ABO-incompatible platelets has been reported in infants [136]. Although Rh matching does not affect post-transfusion platelet increment, small amounts of RBCs (up to 0.2 to 0.3 mL) are present in WB-derived platelets and can cause Rh sensitization in an Rh-negative recipient. Compared to WB-derived concentrates, apheresis platelets contain much less RBC volume (0.0004–0.0005 mL), which is below the minimal volume (0.03 mL) reported to cause Rh alloimmunization [137]. Overall, the frequency of RhD alloimmunization from transfusion of D+ platelets (both apheresis and WB-derived) to both immunocompetent and immunocompromised Rh negative patients has been demonstrated to be low (<1.5%) in recent studies [138]. Nevertheless, administration of Rh immune globulin (RhIg intramuscular dose: 100 IU/mL RBCs transfused, intravenous dose: 90 IU RhIG/mL RBCs) may be considered for Rh-negative neonates, especially females, within 72 hours of exposure to Rh-positive RBCs through platelet transfusion [139].
Dose and Administration
Platelet dosing in neonates may be calculated based on mL/kg or Equivalent Units/kg. An Equivalent Unit (EU) is the volume of a platelet aliquot that contains a minimum of 5.5 × 1010 platelets. Since one apheresis unit contains at least 3 × 1011 platelets, an apheresis platelet unit is equivalent to approximately 6 units of WB-derived platelets. The standard dose based on this method is 1 EU/5–10 kg with a minimum dose of one EU. EU-based dosing offers the advantage of reducing platelet content variability in the transfused product since a minimum dose of platelets will be administered regardless of its volume. This may be clinically significant in sick neonates with thrombocytopenia. A dose of 5–10 mL/kg of WB-derived platelets is expected to yield a platelet increment of 50–100 × 109/L. However, in neonates with increased platelet destruction (as seen in sepsis, DIC, and NAIT), actual post-transfusion increments may be significantly lower.
With NAIT, fetomaternal incompatibility for the HPA-1a and HPA-5b antigens is responsible for 80% and 15% of cases in white Europeans respectively, whereas other antigens may be responsible in other racial groups. If the HPA incompatibility is unknown, then the mother is likely to be the most readily available source of antigen-negative platelets. Maternal platelets should be washed free of antibody-containing plasma and irradiated before transfusion [4]. In the event that maternal platelets are unavailable and laboratory confirmation of the offending antigen is pending, then transfusion of platelets negative for both HPA-1a and HPA-5b is likely to be successful in 95% of white European infants. A retrospective analysis of neonates with severe NAIT demonstrated an increase above a threshold of 40 × 109/L in 24/27 newborns and an increase above a threshold of 80 × 109/L in 16/27 newborns. Therefore, platelet transfusions from random donors are an appropriate strategy in the management of severe NAIT when antigen-negative platelets are unavailable [140, 141]. Platelets should never be warmed or infused through umbilical arterial lines.
Plasma and Cryoprecipitate Transfusions
Plasma can be prepared by either WB separation or by apheresis. When the plasma product is frozen to −18 °C or colder within 8 or 24 hours of collection it is labeled as fresh frozen plasma (FFP) or plasma frozen within 24 hours after phlebotomy (PF24), respectively, and can be stored at this temperature for up to 1 year [4]. Both products are considered to be functionally equivalent for coagulation factor replacement and are used interchangeably in most transfusion practices. Plasma is available in volumes of approximately 250–300 mL if collected from whole-blood donations; or up to 600 mL if derived from apheresis; however, plasma can be separated into a system of multiple satellite bags, and frozen as aliquots for infants who receive only fractions of a plasma unit. Furthermore, plasma can be further subdivided into aliquots via sterile connecting devices after being thawed for multiple neonates, stored at 1–6 °C for up to 5 days, and transfused as thawed plasma (TP) [2]. Although TP has approximately 40% activity of heat labile factors (Factor V and VIII), effective hemostasis is retained at this level, making TP clinically similar to FFP [142].
Crossmatching is not performed, since type-specific or AB-negative product is typically issued. Because the freezing process renders the frozen-thawed plasma component free of viable leukocytes, leukoreduction and irradiation are unnecessary. Plasma is not screened for CMV IgG; therefore, passive transfer of CMV antibody in plasma may result in positive CMV IgG testing in the transfused neonate. This does not represent CMV infection, and the antibody disappears in a time course consistent with the 21 day half-life of gamma globulin [143]
Solvent/detergent-treated plasma serves as an alternative to standard plasma products with decreased risk of transmission of enveloped viruses [144]. Octaplas™ (genesis Biopharma) received Health Canada approval in 2005 and FDA approval in January 2013. It has been available in Europe since 1992 and several countries (e.g., Norway, Republic of Ireland, and Finland) have entirely converted their plasma supply from FFP to Octaplas™. Each lot is prepared from a pool of 630 to 1520 units of FFP, which provides a more consistent level of coagulation factors. The risk of allergic reactions and TRALI appears reduced due to the process of filtration to remove cellular debris as well as the dilution of individual donor plasma proteins. The product is available in 200 mL aliquots, but these cannot be divided into smaller portions, limiting their use in smaller patients. Octaplas™ preparation includes affinity ligand chromatography (to remove prions), several filtration steps, and S/D treatment with trinitrobutyl phosphate and Triton X-100. Through such preparation processes, Octaplas™ is associated with reduced risk of bacterial contamination [145]. In the United States, the shelf-life is 3 years when stored below −18 °C (compared to 1 year for FFP or PF24).
Plasma is used primarily to treat acquired coagulation factor deficiencies as a result of disseminated intravascular coagulation (DIC), dilutional coagulopathy, liver failure, or vitamin K deficiency from malabsorption, biliary disease, warfarin effect, or maternal anticonvulsant therapy. Plasma should be used for specific factor replacement in congenital factor deficiencies only when specific viral-inactivated plasma-derived or recombinant factor concentrates are unavailable [2]. FDA-approved commercial factor concentrates are now available for FVIIa, FVIII, FIX, FXIII, von Willebrand factor (vWF); prothrombin complex, protein C, antithrombin, and fibrinogen. Plasma is not indicated for volume expansion, enhancement of wound healing, or first-line treatment for congenital factor deficiencies when commercial factor concentrates are available. Although earlier studies demonstrated that the incidence of IVH was reduced by prophylactic plasma transfusion, recent evidence has disproved any effect of prophylactic transfusion on rates of death or disability in preterm infants [146]. The use of plasma as a source of immunoglobulins for the treatment of neonatal sepsis, or for the treatment of immunodeficiency states is also unwarranted [147]. There is also no evidence that prophylactic plasma transfusion in neonates undergoing CPB either prevents excessive bleeding or decreases transfusion requirements despite the universal reduction in components of the coagulation and fibrinolytic systems [148]. Bleeding in this situation is often related to platelet dysfunction with or without thrombocytopenia and responds better to platelet administration.
The typical dose of plasma administered is 10–15 mL/kg as this will replace approximately 10%–30% of most factors immediately following transfusion [149]. Further doses are determined by the clinical situation, underlying disease process, and the half-life of the factor(s) being replaced. Furthermore, both the prothrombin time (PT) and activated partial thromboplastin time (aPTT) are prolonged in the neonate as a result of depressed vitamin K dependent factors in neonates, requiring correlation of lab values to clinical status of the infant when assessing plasma component needs in the sick neonate. It is essential to obtain appropriately collected, non-heparinized specimens for evaluation of coagulation factor deficiencies prior to plasma transfusion.
Cryoprecipitate is the protein fraction derived from FFP thawed at 1–6 °C, which is then resuspended in approximately 10–15 mL of plasma and refrozen to −18 °C for storage up to 1 year. Each unit of cryoprecipitate is derived from a single WB donation, and contains a minimum of 80 units of factor VIII activity and 150 mg of fibrinogen [1]. Although there are no standards for the quantity of the other factors, cryoprecipitate also contains vWF, factor XIII, and fibronectin [27]. Cryoprecipitate is the treatment of choice for severe acquired hypofibrinogenemia (<150 mg/dL) associated with bleeding. In general, an infant should receive 1 unit of cryoprecipitate per 5 kg, which increases the total fibrinogen by about 100 mg/dL [143]. Bleeding associated with von Willebrand disease, hemophilia A, and congenital fibrinogen deficiency (including afibrinogenemia and hypofibrinogenemia) should be treated with FDA-licensed recombinant factor concentrates and/or viral inactivated pooled plasma-derived factor concentrates whenever possible.
Granulocyte Transfusions
Despite effective antimicrobial therapy, sepsis-related mortality in preterm infants remains high (up to 15%), as a result of the underlying incompetence of the preterm infant’s immune system. A small neutrophil storage pool and sepsis-related defects in neutrophil deformability, chemotaxis, phagocytosis, and oxidative killing, lead to significant neutropenia and qualitative neutrophil deficiencies in the presence of bacterial infection. Furthermore, placental transfer of IgG is low prior to 32 weeks’ gestation, resulting in hypogammaglobulinemia [150, 151].
The Resolving Infection in Neutropenia with Granulocytes (RING) randomized clinical trial [152] compared standard antimicrobial therapy alone against daily granulocyte transfusion from donors stimulated with granulocyte colony-stimulating factor (G-CSF) and dexamethasone in addition to standard therapy and found no difference in survival or microbial response in the granulocyte arm, although secondary analyses indicated that higher doses of granulocytes achieved through G-CSF stimulation were associated with better outcomes than lower doses [153]. Although children (defined as <18 years of age) were included in this study, they comprised only a minority of the total number of subjects (6/49 in control arm, 4/48 in granulocyte arm) [152]. Studies of the efficacy of granulocyte transfusions for the treatment of life-threatening infections in neonatal patients are difficult to evaluate because of small numbers of patients with varying degrees of illness and supportive care regimens, differing methods of harvesting neutrophils, and different transfusion protocols. Meta-analysis of the safety and efficacy of granulocyte infusion adjunctive to antimicrobial therapy in the treatment of septic neutropenic neonates failed to show that granulocyte infusions reduce mortality or morbidity, although a reduction in the latter approached statistical significance [154]. In spite of the conflicting evidence, granulocyte transfusion may rarely be considered in neonates with qualitative neutrophil defects with severe (or progressive) bacterial or fungal infection who have not responded to appropriate aggressive antimicrobial treatment [135].
Granulocyte concentrates (GCs) for neonatal use are prepared by automated leukapheresis of healthy stimulated donors, and should contain 1.0–2.0 × 109 PMNs/kg in 10–15 mL/kg volume. As discussed above, there is evidence that granulocyte yield may be increased by co-stimulating donors with dexamethasone and G-CSF [153]. Once initiated, daily granulocyte transfusions are recommended until there is clinical improvement and evidence of neutrophil count recovery (ANC + bands >3 × 109/L in the first week of life; >1.5 × 109/L thereafter). The component must be ABO- and Rh-compatible and cross-matched with the recipient, since the product contains a large number of RBCs (Hct: 15%–20%). All GCs should be gamma irradiated but NOT leukodepleted and infused as soon as possible after collection using standard 170 μm filters. Cytomegalovirus-negative donors may be preferentially selected when the recipient is known to be CMV negative because of the risk of CMV transmission, although it may be difficult to consistently provide granulocytes from seronegative donors in areas with high CMV prevalence. It is essential that the medical team and the infant’s parents be aware and consent to the fact that FDA-mandated infectious disease testing will not be completed before the product is released for transfusion because of the product’s 24-hour outdate. This ensures that the risks and benefits of transfusing an untested blood product are considered by all parties.
Intravenous immunoglobulin and growth factors (G-CSF and GM-CSF) have also been attempted to augment traditional antimicrobial therapy to support septic neonates, but the efficacy of such agents requires further investigation. In meta-analysis, the addition of G-CSF or GM-CSF to antibiotic therapy in preterm infants with suspected systemic infection did not reduce immediate mortality, although a significant reduction in mortality was seen in septic neonates with neutropenia [155]. Prophylactic administration of IVIg has not been shown to confer any significant reduction in mortality in infants with suspected infections [156]. Furthermore, IVIg was shown to be inferior in terms of overall survival to granulocyte transfusions when used in conjunction with antimicrobials in neonates with presumed bacterial infection and neutropenia [157].
Granulocyte transfusions have unique risks, and include varying degrees of pulmonary reactions which range from mild transient respiratory distress in 25%–50%, to severe pulmonary edema, hypoxia, and ARDS in about 1% of all transfusions in adults. They are also associated with a high incidence of febrile reactions. Pulmonary complications have been reported in 4% of transfused infants [158], and severe pulmonary reactions resembling TRALI have been reported [159, 160]. Amphotericin B administered within 6 hours of GCs has been suspected to potentiate severe pulmonary reactions, but has not been confirmed in RCTs [161].
Extracorporeal Membrane Oxygenation
Extracorporeal membrane oxygenation (ECMO) is the use of prolonged extracorporeal circulation and gas exchange through a membrane oxygenator to provide temporary life support in patients with profound cardiorespiratory failure who fail to respond to conventional therapy. The transfusion service plays a vital role in supporting the needs of infants on ECMO by providing blood products for the initiation of ECMO as well as ongoing transfusion support throughout the course of ECMO, which may be quite extensive. Under ideal circumstances, fresh, ABO, and Rh compatible RBCs along with type-specific plasma are used to prime the ECMO circuit. However, in cases where ECMO deployment occurs emergently, there may be insufficient time for preparation of blood products meeting all of the above criteria. Provisions for urgent use (i.e., ECMO circuit disruption) often require an inventory of group O-negative RBCs. Blood products used for ECMO should be fresh (<5–10 days), CMV risk-reduced, gamma irradiated, and sickle-negative. Although there is mounting anecdotal evidence that RBCs preserved in extended storage media are safe for ECMO, many ECMO centers use CPDA-stored RBC products or reduce the amount of additive solution prior to use [2].
Hemostatic complications during ECMO are multifactorial and include the following: systemic heparinization to prevent clot formation in the circuit, qualitative and quantitative platelet deficiencies resulting from activation and consumption within the circuit, activation of the coagulation cascade and simultaneous fibrinolysis from continuous contact of plasma proteins with the prosthetic surfaces, and underlying predisposing factors to coagulopathy (i.e., acidosis, DIC, hypoxia). For these reasons, bleeding is common in ECMO patients. Despite aggressive blood component support, the Extracorporeal Life Support Organization (ELSO) registry reports a 15% incidence of ICH and 7% incidence of other major bleeding throughout an ECMO course [162, 163].
Ongoing blood product support throughout ECMO varies based on transfusion triggers and the indication for ECMO [164]. Platelet counts decrease by a mean of 26% from baseline counts within 15 minutes of initiating ECMO and continue to decline further thereafter, while platelet function is reduced considerably. Sepsis while on ECMO increases platelet requirements. Consequently, platelet counts generally are maintained between 100 and 200 × 109/L, depending on the assessed risk of hemorrhage in the individual infant [165]. Maintaining a platelet count ≥150 × 109/L is recommended for high risk infants, and ≥200 × 109/L is often recommended postoperative in diaphragmatic hernia repair cases and/or for active bleeding. Cryoprecipitate is administered to maintain fibrinogen ≥100 mg/dL, and Hct is maintained at 40%–45%, to optimize oxygen delivery [166].
In the past, blood usage for neonates on ECMO resulted in a mean of 30 donor exposures per ECMO course; however, this been reduced to 7–10 donor exposures in many centers by employing donor exposure-limiting strategies (i.e., use of single-donor split products) [167].
Management of ECMO Patients Using Factor Concentrates such as Antithrombin III
Although on-demand dosing of antithrombin concentrates (Thrombate, ATryn) for pediatric patients on ECMO increases antithrombin levels and decreases heparin requirements for at least 12 hours after dosing, no statistical differences in the number of circuit changes, in vivo clots or hemorrhages, transfusion requirements, hospital or ICU length of stay, or in-hospital mortality were identified compared to controls [168, 169]. Similarly, studies of antithrombin supplementation in children on cardiopulmonary bypass have demonstrated decreased heparin doses without significant impact on clinical outcomes [170].
Adverse Reactions to Blood Transfusion
The physiological immaturity of various organ systems in the newborn gives rise to significant differences in the incidence and types of adverse reactions when compared with older children and adults. Whenever a patient is transfused, he/she should be closely monitored for signs of a reaction. Often, a potential reaction is noted by a change in vital signs, respiratory status, or skin manifestations (e.g., rash, hives). Typically, these changes present early in the transfusion, but they may occur at any point. The Joint Commission recommends monitoring vital signs pre-transfusion, within 15 minutes of initiation, and within 1 hour of transfusion end [171]. Hospital policies are based on these guidelines but may differ with respect to each other.
Febrile Nonhemolytic Transfusion Reactions
Febrile nonhemolytic transfusion reactions (FNHTRs) are characterized by fever, chills, and diaphoresis. These reactions are believed to result from the release of pyrogenic cytokines by leukocytes within the plasma during storage. The incidence of FNHTRs has decreased dramatically since the implementation of prestorage leukoreduction of RBCs and platelet products in 1999. Whereas FNHTRs occurred in approximately 10% of transfusions in the past, the incidence for all products since the introduction of leukoreduction is now 0.1% to 3% (approximately 0.2% for prestorage leukoreduction) [22, 23]. When FNHTR is suspected, the transfusion should be stopped. While FNHTR is typically self-limited, it is important to investigate the possibility of more serious reactions. A sample of blood from the patient may be sent for DAT, plasma hemoglobin quantification, serum lactate dehydrogenase, bilirubin level and/or urine blood to ensure that the patient is not experiencing a hemolytic transfusion reaction. The transfusion service may repeat the crossmatch between the RBC unit and a post-transfusion patient sample to ensure there is no new unexpected incompatibility. Bacterial contamination should be assessed via cultures of the transfused product and the patient’s blood; empiric antibiotic therapy may be warranted. Most FNHTRs respond to antipyretics, and meperidine may be used for rigors [172, 173].
Allergic Transfusion Reactions
Allergic transfusion reactions (ATRs) are marked by urticaria and itching, but can include flushing, bronchospasm, and anaphylaxis in severe cases. For mild or localized cutaneous cases, the transfusion can be continued once symptoms have subsided; this is the only scenario in which transfusion can be reinitiated with the same unit after a reaction. Severe allergic reactions (anaphylactoid or anaphylactic reactions) may require treatment with corticosteroids and/or epinephrine. The same blood unit should never be restarted in severe cases, even after symptoms have abated. Leukoreduction does not decrease the incidence of ATRs as it has for FNHTRs [23]. Premedication with antihistamines with or without steroids is recommended for ATRs. Because these reactions are caused by an antibody response in a sensitized recipient to soluble plasma proteins within the blood product, washed RBCs and platelets may be used for severe or recurrent ATRs nonresponsive to medication. Severe ATRs leading to anaphylaxis can be caused by the development of anti-IgA antibodies in recipients who are IgA-deficient. In these instances, IgA-deficient–plasma products may be obtained, but require the use of rare donor registries [174]. In patients of Asian descent, haptoglobin deficiency may also be associated with severe ATRs [175].
The use of platelets in additive solution (PAS) resulted in decreased incidence of ATRs and FNHTRs in one adult study, presumably due to decrease in donor plasma content in the blood products [176]. However, neonates represented only 1.8% of the study population. Other retrospective reports describing PAS use have included children and neonates, but overall the effect of PAS platelets in neonates requires further study [177].
Hemolytic Reactions
Acute hemolytic transfusion reactions are rare in neonates, partly due to the absence of naturally occurring isoagglutinins before 4 months of age, but also due to the practice in some NICUs of transfusing neonates with group O blood. Although there are case reports of anti-RhE and anti-Kell formation in infants as young as 18 days of life, the majority of reports support the infrequency of RBC alloimmunization and delayed hemolytic transfusion reactions (DHTRs) in infants less than 4 months of age due to their immunologic immaturity [178, 179]. A cohort study of 1641 neonates and children up to the age of 3 years found no alloimmunization cases within the first 6 months of life. The authors concluded that after initial testing, repeat antibody screening, and cross-matching during the first 4 months of life could be safely omitted [180]. This approach also reduces iatrogenic blood loss due to repeated laboratory sample draws.
A phenomenon known as “T-activation” can cause immune-mediated hemolysis in neonates, which can range from minor to severe and fatal, if not recognized. Removal of N-acetyl neuraminic (sialic) acid residues from the O-linked oligosaccharides on glycophorins (A, B, and C) on RBC membranes by neuraminidases produced by bacteria, particularly Clostridium bacteroides and Streptococcus pneumoniae, results in activation of the normally masked Thompsen–Friedenreich (T) cryptantigen on the red-cell surface of the neonate. Transfusion of adult blood containing naturally occurring anti-T antibodies into neonates with T-activation can present with evidence of intravascular hemolysis following transfusion of blood products, or unexplained failure to achieve the expected post-transfusion hemoglobin increment. T-activation has been reported mainly in neonates with necrotizing enterocolitis, especially in those with severe disease requiring surgical intervention, but also in septic infants with other surgical problems [181]. Routine cross-matching techniques will not detect the polyagglutination due to T-activation when monoclonal ABO antiserum is used. Minor cross-matching of neonatal T-activated red cells with donor anti-T-containing serum may show agglutination, but this is not performed routinely. Infants with discrepancies in forward and reverse blood typing and evidence of hemolysis on smear should be suspected of T-antigen activation. The diagnosis is confirmed by specific agglutination tests using peanut lectin Arachis hypogea and Glycine soja, but such reagents may not be readily available. Further hemolysis may be prevented by using washed RBC and platelets, and low-titer anti-T plasma if available [181, 182]. Exchange transfusion with plasma-reduced components may be necessary for infants with severe ongoing hemolysis. The clinical significance of hemolysis mediated by anti-T and the need to avoid plasma transfusion in these patients remains a topic of discussion [183].
Numerous nonimmunologic causes of hemolysis can occur in neonates, such as exposure of RBCs to thermal and mechanical stresses (see RBC section), co-administering incompatible fluids and/or drugs, and the transfusion of abnormal donor RBCs (i.e., G6PD deficiency) [184, 185].
Donor Leukocyte Effects
The presence of donor leukocytes in blood products can contribute to HLA alloimmunization, which can complicate future medical interventions by increasing transplant graft failure rate or contribute to platelet refractoriness. Another described phenomenon is transfusion-related immunomodulation (TRIM), a process in which leukocytes, cytokines, stored RBCs, or other biological response mediators alter the recipient’s immune system. Both effects are improved, although not eliminated, by pre-storage leukoreduction of blood products [186, 187]. The effectiveness of other interventions to address TRIM (e.g., washing) has not been established [188]. The characteristics and impact of TRIM in critically ill pediatric patients are under investigation [189].
Transfusion-Related Acute Lung Injury
The passive transfusion of blood containing leukoagglutinins (HLA or neutrophil antibodies) directed against the recipient’s leukocytes initiates complement activation with microvascular lung injury. This condition termed transfusion-related acute lung injury (TRALI) typically manifests with non-cardiogenic pulmonary edema, severe hypoxemia, hypotension, fever, and transient leukopenia within 6 hours of transfusion. TRALI has been well documented in the pediatric population, but is reported only rarely in neonates partly because of the difficulty in distinguishing the acute respiratory distress that accompanies the syndrome from other causes of respiratory deterioration [188]. High-volume plasma products (FFP, apheresis platelets, GCs) account for the majority of severe TRALI cases, and multiparous women are the most commonly implicated donors [190]. Use of high-volume plasma products from men, women who have never been pregnant, or previously pregnant women who have tested negative for HLA antibodies has dramatically decreased the incidence of TRALI [191]. A case has been reported of a 4-month-old girl who experienced TRALI within 2 hours of completion of a RBC transfusion from her mother. HLA antibodies were identified in the mother’s serum demonstrating the possible role of HLA antibodies in the pathogenesis of TRALI in the setting of a designated blood transfusion between mother and infant [192]. TRALI has been documented as complicating granulocyte transfusions in neonates [159, 160].
Transfusion-Associated Circulatory Overload
Transfusion-associated circulatory overload (TACO) is defined as acute respiratory distress associated with signs of fluid overload, including tachycardia, hypertension, pulmonary edema, positive fluid balance, or elevated levels of brain natriuretic peptides. It contributes to deaths attributable to transfusion. Neonates are at risk because their small blood volume may not accommodate rapid or large volume transfusions. Other risk factors include pre-existing cardiac or renal dysfunction, and hospitalization in the intensive care unit [193]. The presentations of TACO and TRALI are similar, and both may be difficult to differentiate from the patient’s underlying disease. Diuretics may be helpful in supporting patients affected by TACO. Preventative options include anticipating blood needs in order to transfuse smaller aliquots at a lower rate, selecting lower-volume alternatives (e.g., volume reduced units or factor concentrates), and closely monitoring the fluid balance [194].
Transfusion-Associated Graft-versus-Host Disease
Transfusion-associated graft-versus-host disease (TAGVHD) occurs due to the proliferation and engraftment of viable donor lymphocytes in an immunosuppressed or immunodeficient transfusion recipient who does not recognize the cells as being foreign and is unable to reject them. Similarities in HLA antigens facilitate engraftment and, therefore, this phenomenon is much more likely to occur when family members serve as blood donors or when the population is relatively homogeneous. Infants at high risk of TAGVHD include those receiving transfusions from blood relatives, those receiving IUTs, those receiving postnatal transfusions who had received IUTs, those receiving large volumes transfusions (i.e., exchange transfusion), and those with primary cell-mediated immunodeficiency disease [195–198]. Although there exists no “standard of care” regarding irradiation of blood products for infants without TAGVHD risk factors, some institutions adopt universal irradiation policies, whereby all infants less than 4 months of age receive irradiated cellular blood products so as to avoid TAGVHD in patients with an undiagnosed immunodeficiency. Other transfusion centers irradiate all cellular blood products given to preterm infants with birth weights ≤1.0 to 1.2 kg [199]. The known and presumed indications for irradiation of blood components for neonates are listed in Table 20.3.
The clinical signs and symptoms of TAGVHD typically present 3 to 30 days following transfusion of a leukocyte replete cellular component, and include fever; generalized, erythematous rash with/without progression to desquamation; diarrhea; hepatitis (mild to fulminant liver failure); respiratory distress; and severe pancytopenia resulting from graft T lymphocyte-induced hematopoietic progenitor cell death. The mortality rate is approximately 90% in the pediatric population, mostly as a result of bone marrow hypoplasia. Treatment supportive, as there exists no effective curative therapy [178].
TAGVHD can be abrogated by pretransfusion gamma irradiation of all cellular blood components at 2,500 cGy. The shelf life of irradiated red cells is 28 days; however, no data currently exists on the safety in the neonatal population of gamma irradiated RBCs that are stored for this amount of time [200]. Because potassium and free hemoglobin increase after irradiation and storage of RBCs, it is preferable to irradiate cellular blood products close to administration time for neonates, who may not be able to tolerate high potassium loads; this is often referred to as irradiation on issue.
Transfusions and Necrotizing Enterocolitis
Another concern linked to RBC transfusions is the development of neonatal NEC; several retrospective studies have demonstrated a temporal association between the two. They report that 25%–38% of NEC cases occur within 48 hours of RBC transfusion and that the risk of transfusion-associated NEC increases with decreasing gestational age of the infant [201–204]. Blau et al. found a convergence of transfusion-associated NEC at 31 weeks’ gestation, the age of presentation of O2 toxicity and other neovascularization syndromes [202]. In another retrospective report, Singh et al. displayed a strong association of transfusion within 24 hours and NEC (OR = 7.60, p = 0.001), a significant albeit decreased association for transfusion within 48 hours (OR = 5.55, p = 0.001), and a statistically insignificant (absent) association for transfusion within 96 hours (OR = 2.13, p = 0.07) in their multivariate analysis [205]. Although attempts were made to minimize the confounding effects of multiple variables, the effect of infants’ nadir hematocrit level on the risk of developing NEC remained statistically significant, making it impossible to separate the influence of hematocrit and RBC transfusion.
However, other studies suggest that RBC transfusions may be an epiphenomenon with respect to NEC rather than a contributor to the pathogenesis of disease. Bednarek et al. reported that there was no significant difference in the incidence of NEC between high versus low hematocrit threshold transfusion practices among six NICUs [8], and the PINT trial did not show a difference in the incidence of NEC between the low versus high hematocrit transfusion threshold groups [14]. In a meta-analysis of the published literature on transfusions and NEC, increased RBC transfusions were associated with lower rates of NEC. Thus, the direction of effect of RBC transfusions on NEC in randomized trials was opposite to that seen in observational studies [206]. Keir and colleagues performed a separate systematic review and did not find an association between transfusions and NEC [18]. A meta-analysis of 17 observational studies reported similar results, with the caveat that these studies were predominantly of low-to-moderate quality [207]. One recent prospective study of 598 very low birth weight infants found that severe anemia (hemoglobin <8 g/dL), but not RBC transfusion, was associated with an increased risk of NEC. Thus, prevention of severe anemia may be more important than avoidance of RBC transfusion alone [208]. The temporal relationship observed between RBC transfusion and neonatal NEC may represent reverse causation, whereby clinical instability from evolving NEC leads to RBC transfusion prior to the formal diagnosis of NEC. A prospective, multicenter observational cohort study of infants with birth weight less than or equal to 1250 g is underway to investigate the associations between RBC transfusion, product irradiation, anemia, and intestinal oxygenation and injury that lead to NEC [209].
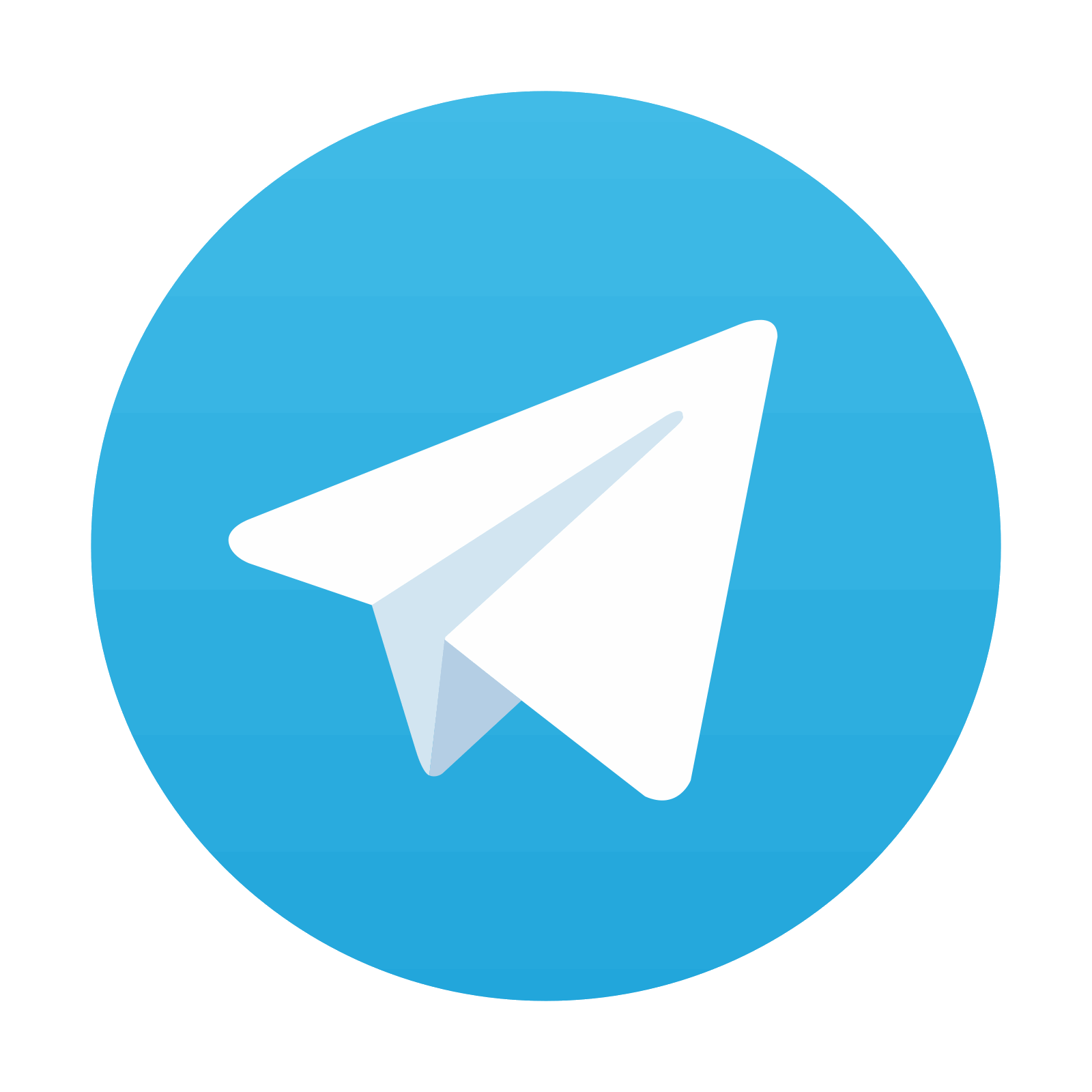
Stay updated, free articles. Join our Telegram channel
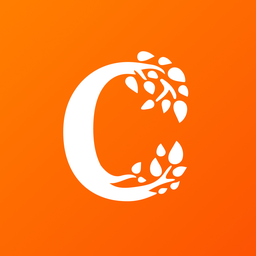
Full access? Get Clinical Tree
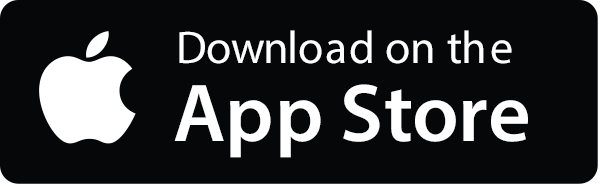
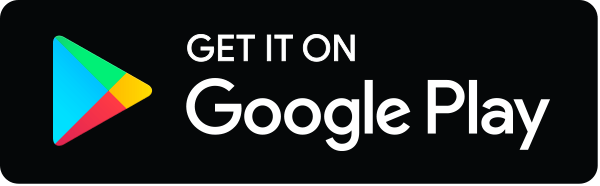
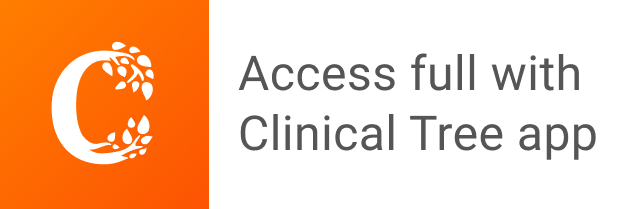