Abstract
Hematopoiesis refers to the continuous production and release of blood cells into the circulation. As blood cells become old or injured, self-renewing hematopoietic stem cells (HSC) proliferate and differentiate to replenish multiple hematopoietic lineages. This process produces nearly 200 billion red blood cells, 10 billion white blood cells, and 400 billion platelets every day. In addition to the requirement for high cell production, the concentration of individual blood cell lineages is precisely regulated in the peripheral blood and tissues. The production and use of circulating blood cells increase during periods of altered homeostasis such as defense against infection or replenishment of circulating red cells after hemorrhage. When the tightly regulated production of blood cells fails, the host may encounter life-threatening anemia or other cytopenias or suffer from excessive neoplastic growth of blood cells manifesting as leukemia.
Hematopoiesis refers to the continuous production and release of blood cells into the circulation. As blood cells become old or injured, self-renewing hematopoietic stem cells (HSC) proliferate and differentiate to replenish multiple hematopoietic lineages. This process produces nearly 200 billion red blood cells, 10 billion white blood cells, and 400 billion platelets every day. In addition to the requirement for high cell production, the concentration of individual blood cell lineages is precisely regulated in the peripheral blood and tissues. The production and use of circulating blood cells increase during periods of altered homeostasis such as defense against infection or replenishment of circulating red cells after hemorrhage. When the tightly regulated production of blood cells fails, the host may encounter life-threatening anemia or other cytopenias or suffer from excessive neoplastic growth of blood cells manifesting as leukemia.
During embryogenesis, hematopoiesis occurs in tightly regulated, tissue specific sites, including the extraembryonic yolk sac, para-aortic mesonephric area, fetal liver, and the preterm marrow. A harmonious relationship between hematopoietic stem cells, neighboring tissue stromal components, and hematopoietic growth factors promotes the evolution of red cell production throughout human development. This chapter will briefly review the cellular model of hematopoiesis starting in the human embryo. Although human hematopoiesis is the focus of this chapter, certain concepts gathered from murine studies will be presented to demonstrate some basic hematopoietic principles [1].
Overview of Hematopoiesis
Early Blood Cell Investigators
With the invention of the microscope came the identification of the first blood cell in the late 1600s: erythrocytes. Dutch scientist Antonie van Leeukwenhoek described red blood cells as “sanguinous globules” that were “flexible and pliant” in order to pass through small vessels [2]. Colorless white blood cells were not identified for nearly 200 more years, when a medical student named Paul Ehrlich devised a triacid stain that allowed for the identification of different white blood cell lineages based on size, nuclear shape, and cytoplasmic elements. Platelets were first described in the mid 1800s as small globules derived from plasma [3], and later established as the third element of blood, distinct from white and red cells, by Giulio Bizzozero [4]. With additional improvements in microscopes and triacid stains, the field of hematology began to expand, and changes in blood cell number, morphology, and function were correlated with specific human diseases. Further progress in studying hematopoiesis was largely limited to conjecture and opinion derived from morphologic studies of blood cell-containing tissues until the middle of the twentieth century, when hematologists began to examine the hematopoietic consequences of animal exposure to ionizing radiation [2].
Methods of Identifying Hematopoietic Stem Cells and Progenitor Cells
Assays of hematopoietic stem and progenitor cell (HSPC) potential were first developed in the 1950s–1960s. After discovering that whole-body irradiation of animals led to significant pancytopenia (i.e. reduction in blood cell counts), scientist Lo Jacobson discovered that mice were protected from hematopoietic effects of radiation if their spleens were shielded or if irradiated mice were subsequently infused with blood cells from a healthy mouse [5]. Confirmation that hematopoietic cells, not plasma, conferred radiation protection was reported by Ford and colleagues in 1956 [6]. In 1961, Till and McCulloch provided evidence that single, multipotent hematopoietic precursors (i.e. cells giving rise to more than one lineage of blood cells) could be identified in vivo by injecting the donor marrow cells into the spleen of a lethally irradiated recipient animal and examining the recipient spleen for hematopoietic colonies 8 to 12 days later ([7]; Fig. 2.1). Each colony of hematopoietic cells in the spleen arose from a single precursor cell, the colony-forming unit in the spleen cell (CFU-S) [7]. Although this method advanced the study of hematopoietic stem cell biology, little was known in the early 1960s of the mechanisms that caused or permitted stem cells to differentiate into mature cells.
Fig. 2.1 Image of the various erythroid colonies. Examples of colonies derive from murine definitive and primitive erythroid progenitors. Immature BFU-E derived colonies, counted at day 7 of culture, typically consist of one or more large colonies surrounded by smaller colonies, which constitute a “burst.” Late-stage BFU-E derived colonies, counted at day 3 of culture, consist of smaller single colonies. CFU-E derived colonies, counted at day 2 of culture, consist of a single small colony containing 16–64 hemoglobinizing cells. Primitive erythroid progenitors (EryP-CFC) derived from the mouse embryo or from differentiating mouse embryonic stem cells generate single compact grape-like colonies of large primitive erythroblasts at 5 days of in vitro culture. Abbreviations: d, day.
In the mid 1960s, several groups demonstrated that murine hematopoietic cells could be cultured in vitro [8, 9]. Additionally, these single precursor cells, called colony-forming unit in culture (CFU-C), could differentiate into myeloid colonies when grown in the presence of soluble fluid from murine organs (i.e. urine or pregnant uterine extracts), which became known as colony-stimulating activity (CSA). Later work revealed that these colonies were composed of granulocytes, macrophages, or both (CFU-GM), and that the specific cell type formed was based on the type of CSA added. Later, using anemic mouse serum as a growth factor, Axelrad and coworkers demonstrated that red blood cell colonies were clonally derived in vitro, and they called the most primitive red cell precursors the erythroid burst-forming unit (BFU-E) and the most committed erythroid precursors colony-forming unit-erythroid (CFU-E) cells ([10]; see Fig. 2.1). Multipotent progenitor cells were also identified and called colony-forming unit-mix (CFU-Mix). These assays, developed for studying murine hematopoiesis, were rapidly adapted for human studies and continue to be sensitive assays for detecting human hematopoietic progenitor cells [11].
Because the frequency of human and murine hematopoietic stem cells is estimated to be 1 of 104 to 105 marrow cells [12, 13], the primary obstacle in studying human hematopoietic stem cell biology is that few methods can identify these rare cells. As such, results of studies of murine hematopoietic stem cells were essential to advancing our understanding of human hematopoiesis. In fact, it was murine bone marrow transplantation experiments that provided the evidence to reliably identify hematopoietic stem cells, which were defined as cells that self-renew in vivo, proliferate, and differentiate into all lineages of circulating peripheral blood cells for more than 4 months after transplantation into recipient animals [14]. Because of ethical constraints, similar in vivo reconstitution assays in human patients are not possible, and several alternative in vitro and in vivo estimates of hematopoietic precursors have been developed.
In vitro studies have identified two types of hematopoietic progenitor cells. Lineage-committed hematopoietic progenitor cells form colonies in vitro in response to one or two recombinant growth factors. In contrast, more primitive multipotent hematopoietic cells form in vitro colonies only when cultured with multiple recombinant growth factors. These results have been interpreted as evidence that the more primitive hematopoietic stem and progenitor cells (HSPC) require complex growth factor combinations to fuel or support lineage commitment and progenitor cell differentiation. Plating hematopoietic stem cells in special double-layer agar cultures with multiple recombinant cytokines permits identification of blood progenitors that are highly proliferative (i.e., high proliferative potential colony-forming cells (HPP-CFC)). High proliferative potential colony-forming cells produce colonies containing more than 50,000 cells that are visible without magnification (most CFU-Cs are less than 50,000 and microscopic) and are considered to be the most primitive progenitor cell grown in semi-solid medium without added stromal cells.
Additionally, complex in vitro cultures of hematopoietic stem cells with a monolayer of bone marrow or fetal liver stromal cells (includes macrophages, endothelial cells, fibroblasts, and preadipocytes) results in sustained hematopoiesis for several months [15]. The most primitive hematopoietic cells (i.e. those giving rise to hematopoietic progenitors) reside beneath the stromal monolayers, whereas the mature blood cells produced are released into the tissue culture medium as nonadherent cells [16]. Using limiting dilution analysis, the number of such murine or human long-term culture-initiating cells (LTC-IC) that give rise to multipotent and committed progenitors for 3 to 4 weeks in vitro can be calculated [17]. As such, LTC-IC are considered to be the most primitive hematopoietic precursor detectable by means of in vitro assays.
In attempts to develop an in vivo system for detection of human hematopoietic stem cells, several groups developed xenotransplantation models. Enriched populations of human hematopoietic stem cells from human bone marrow, cord blood, or fetal liver have been observed to engraft in the pre-immune sheep fetus (<60 days of gestation) with long-term evidence of multilineage peripheral human blood cell chimerism in the transplanted animals [18]. Nonobese diabetic (NOD) mice bred with severe combined immunodeficient (SCID) mice produce NOD/SCID mice that accept human hematopoietic grafts [19]. This NOD/SCID model permits calculation of the frequency of human repopulating cells in a donor sample, but it remains unclear whether this assay is robust and can accurately identify the same hematopoietic stem cells that repopulate the blood system of a larger animal such as a nonhuman primate [20]. More recent immunodeficient murine models apparently permit higher levels of human cell engraftment and even full lymphoid reconstitution under certain circumstances, but these models have not yet been compared to the nonhuman primate models for detection of engrafting long-term repopulating human stem cells [21–24].
Stem Cell Model of Hematopoiesis
The stem cell theory of hematopoiesis posits that the hematopoietic system comprises a continuum of functionally distinct hematopoietic cell compartments. Stem cells are rare, self-renewing, relatively quiescent cells (Fig. 2.2). In the mouse, hematopoietic stem cells have a half-life (i.e., time to 50% of stem cells cycled) of approximately 19 days [25], though slower rates may be identified in a subset [26]. Stem cells can divide to give rise to a daughter cell that retains all of the pluripotentiality of the parent (i.e., self-renewal) or can proliferate into daughter cells that have lost some multipotentiality and have become committed to producing progenitor cells [27]. After the commitment decision is made, the progenitor cell gives rise to progressively more lineage-committed hematopoietic progenitor cells and eventually to mature blood cells (see Fig. 2.2). It remains unclear how hematopoietic stem cells become committed to follow a certain lineage, but thought to be regulated by multiple environmental factors including tissue specific growth factors, cytokines, metabolic products, oxygen levels, extracellular matrix molecules, or cell-cell interaction and feedback loops [28–32].
Fig. 2.2 Timeline of hierarchical models of hematopoiesis. (a) Visualization based on cutting-edge research around the year 2000: HSC are represented as a homogeneous population, downstream of which the first lineage bifurcation separates the myeloid and lymphoid branches via the common myeloid progenitor (CMP) and common lymphoid progenitor (CLP) populations. (b) During the years 2005–2015, this visualization incorporates new findings: The HSC pool is now accepted to be more heterogeneous both in terms of self-renewal (vertical axis) and differentiation properties (horizontal axis), the myeloid and lymphoid branches remain associated further down in the hierarchy via the lymphoid-primed multipotential progenitor (LMPP) population, the GMP compartment is shown to be fairly heterogeneous. (c) From 2016 onwards, single-cell transcriptomic snapshots indicate a continuum of differentiation. Each red dot represents a single cell and its localization along a differentiation trajectory. Abbreviations: DCs, dendritic cells; EoBP, eosinophil–basophil progenitor; GMP, granulocyte–monocyte progenitors; LT, long-term; ILCs, innate lymphoid cells; MEP, megakaryocyte–erythrocyte progenitors; NK, natural killer cells; ST, short-term. (From Laurenti E, Göttgens B. From haematopoietic stem cells to complex differentiation landscapes. Nature 2018; 553(7689):418–26.)
With the development of flow cytometry, monoclonal antibodies to cell-surface antigens, and in vivo and in vitro assays for primitive hematopoietic cells, investigators succeeded in identifying enriched populations of human hematopoietic stem cells and provided evidence that a single murine hematopoietic stem cell is sufficient to restore multilineage hematopoiesis in an irradiated host [33, 34]. Purification of hematopoietic stem cells permitted investigators to pursue basic investigations of stem cell biology that translated into new methods for human hematopoietic transplantations and improved methods of somatic gene transfer. Further studies to examine hematopoietic stem cell function in patients with bone marrow failure syndromes and hematologic malignancies will also probably translate into clinical therapies.
A significant challenge to the in vitro study of hematopoietic stem cells is the realization that many of the growth factors required to stimulate the in vitro proliferation and differentiation of hematopoietic progenitor cells are associated with measurable declines in the concentrations of functional hematopoietic stem cells. Although certain stromal cell lines and primary human or murine stromal cells can maintain hematopoietic stem cell function in vitro, only in the last several decades have tissue culture conditions been developed that permit expansion of murine hematopoietic cell numbers with retention of individual hematopoietic stem cell self-renewal and reconstituting ability [35–37]. The conditions to support in vitro expansion of adult human and murine bone marrow hematopoietic progenitor cells indicate that the proliferative potential and growth factor requirements for survival in vitro differ among hematopoietic cells during ontogeny [36, 38].
Embryonic Hematopoiesis
Studies of Murine Embryonic Hematopoiesis
The murine visceral yolk sac is composed of visceral endoderm and mesoderm cells that proliferate, differentiate, migrate, and co-localize during gastrulation. The endoderm cells face the yolk sac cavity and are highly active in the absorption of material from the maternal circulation and in the biosynthesis and secretion of numerous nutrients [39]. In the mouse, yolk sac endoderm cells are also the primary route for transporting maternal immunoglobulins into the embryo. The mesoderm cells of the yolk sac give rise to blood islands containing blood cells and endothelial cells [40–42]. The simultaneous appearance of endothelial and hematopoietic cells in the blood islands, the apparent intimate cell-to-cell contact of the blood island endothelial and hematopoietic cells, and the shared requirements for certain growth factors and growth factor receptors support the idea that blood island endothelial cells and hematopoietic cells must be derived from a common precursor, called the hemangioblast [41–43]. Study of the hemangioblast remains elusive, although some reports have suggested that isolating the cell is feasible in the murine or chick embryo [43–46].
The precise temporal and spatial origins of the first hematopoietic stem cells in the murine embryo remain unknown. Studies using chicks and frogs indicate that the mesoderm cells that become committed to the formation of blood cells migrate from the posterior primitive streak and into the embryo and the extraembryonic yolk sac [40]. The mesoderm cells contributing to the formation of murine blood cells are also restricted to the posterior primitive streak at 6.75 days postconception (DPC). Some mesoderm cells fated to become blood cells migrate along the extraembryonic endoderm cells and participate in forming the extraembryonic yolk, sac blood islands [40]. Presumably, certain mesoderm cells are acted on by local factors to undergo differentiation into endothelial and hematopoietic stem and progenitor cells. Although little is known about the factors that induce murine mesodermal cell commitment to blood and endothelial differentiation, some evidence suggests that certain transcription factors and growth factor receptors are required for this process [28, 47]. Because blood cells arise from different sites at different times during murine embryogenesis, the site of origin of hematopoietic stem cells remains a vital question in experimental hematology. Hematopoietic stem cells may arise de novo in each hematopoietic site during ontogeny [48, 49]. Alternatively, all hematopoietic stem cells may arise in the embryonic yolk sac and/or the para-aortic splanchnopleura (PS) and migrate to all other sites during development [50–53]. Several groups of investigators have identified hematopoietic stem cell activity in the mouse that appears to originate from the ventral wall of the embryonic paired dorsal aorta and/or from the subendothelial mesenchyme of the PS [54–58]. The most recent data suggest that all murine hematopoietic stem and progenitor cells that reside in the bone marrow compartment are derived from hemogenic endothelium present in embryonic and extraembryonic vessels [57, 59, 60]. It is also apparent that biomechanical forces generated by flowing blood promote the emergence of the first blood cells [61–63].
Differentiated blood cells are first recognizable in the murine yolk sac at 7 DPC. The primary cells observed are nucleated red blood cells expressing embryonic forms of hemoglobin [64]. Hematopoiesis peaks in the murine yolk sac at 11 DPC, and the liver becomes the predominant site of hematopoiesis at 12 DPC. The murine fetal liver remains the primary site of hematopoiesis until the time of birth, when the bone marrow and to some extent the spleen provide lifelong provision of circulating blood cells (Fig. 2.3). Although red blood cell production predominates in the liver and spleen, white blood cell production predominates in the bone marrow compartment [64].
Fig. 2.3 The composition of the HSPC compartment changes in space and time. HSPCs are found in many organs in the body across a lifetime. Cells of different colors represent distinct HSPC subsets. It is unclear whether all HSPC subsets and differentiation trajectories are present in the same proportion in each of the organs. Current evidence suggests that age-related changes result from a combination of shifts in the composition of the HSPC pool, as well as phenotypic changes in particular cell types driven by intrinsic genetic or epigenetic changes and systemic alterations of the microenvironment. Abbreviation: AGM, aorta gonad mesonephros.
The pattern of hematopoietic cell differentiation also varies greatly in the yolk sac and PS. Primitive red blood cells, macrophages, and megakaryocytes are produced in the early yolk sac. The red blood cells are called primitive because they are large, nucleated cells that contain forms of hemoglobin expressed only during the embryonic phase of development. Other hematopoietic progenitors emerging in the yolk sac include BFU-E (expressing primitive and adult-type hemoglobins), CFU-GM, and CFU-Mix that vary with the age of the donor embryo [65]. Culture of hematopoietic progenitors from the PS gives rise to BFU-E and CFU-GM that are composed of cells with definitive features; red blood cells are enucleated and synthesize adult-type hemoglobins, and granulocytes predominate over macrophages in the CFU-GM. Of interest, more recent data suggest that all of the circulating definitive progenitor cells that seed the fetal liver at 10 DPC and differentiate into mature elements by 12 DPC are derived from the yolk sac and not the embryo proper [66].
Studies of Human Embryonic Hematopoiesis
The inability to use transplantation methods for identification of hematopoietic stem cells in human patients has limited the analysis of stem cell function during human embryonic hematopoiesis. However, much of the ontogeny of blood cell production in the human embryo has been described using morphologic approaches and some in vitro hematopoietic progenitor cell assays. Human hematopoiesis occurs in a sequential process throughout the embryonic and fetal period: the yolk sac, the aorta–gonad–mesonephros region (AGM), the fetal liver, and finally the bone marrow (see Fig. 2.3). This section reviews the formation and function of the human yolk sac, determination of the site and kinetics of blood cell appearance, and determination of the number and behavior of cultured human embryonic hematopoietic progenitor cells.
The human yolk sac develops in three stages: a formative period, a period of yolk sac function, and a period of yolk sac regression [67]. The first phase of yolk sac formation can be further subdivided into formation of the primary and secondary yolk sacs. The primary yolk sac is formed after implantation (7 to 8 DPC) by proliferation and differentiation of primitive endoderm cells into visceral endoderm adjacent to the developing embryonic disk and parietal endoderm projecting into the uterine lumen. This simple bilaminar structure has no identifiable function, but the endoderm cells lining the primary yolk sac give rise to mesodermal precursors (i.e. intermediate cells) that colonize the space between the yolk sac cavity and the surrounding trophoblast cells. The primary yolk sac then appears to collapse, with heterogeneous foci of re-expansion breaking up the primary yolk sac into smaller vesicles. The secondary yolk sac is formed (12 to 15 DPC) from the remnants of the primary yolk sac (i.e. parietal endoderm and mesoderm) that are contiguous with the visceral endoderm underlying the embryonic disk.
The secondary yolk sac is an active site of protein biosynthesis, nutrient transport, and hematopoiesis. A variety of proteins are synthesized including albumin, alpha–fetoprotein, prealbumin, apolipoproteins, insulin-like growth factor, transferrin, vascular endothelial growth factor, and colony-stimulating factor 1 (i.e. macrophage colony-stimulating factor) [67]. The endodermal component of the secondary yolk sac is a transporter of proteins and vitamins [68]. Another primary function of the secondary yolk sac is to produce hematopoietic cells. The first primitive red blood cells in the yolk sac adhere to surrounding endothelial cells, and these hematopoietic-endothelial masses are called blood islands [69]. Commensurate with blood island formation, the mesenchymal layer in the secondary yolk sac thickens, but the endoderm layer is not affected.
Macroscopically, the secondary yolk sac begins as a small structure (<0.4 mm) but steadily increases in size to 2 mm by day 19, to 4–5 mm by 7 weeks, and 6–6.5 mm by the end of week 10. As the yolk sac matures, an extensive network of superficial capillaries is formed as the blood island endothelial cells migrate toward one another and form vascular channels. The secondary yolk sac circulation is fed and drained by the vitelline arteries and veins, which connect the embryonic to the extraembryonic circulation. Initially, the vitelline vessels empty into the sinus venosus of the liver, establishing a preportal-type circulation. Later in gestation, the yolk sac regresses, new capillaries arising from the developing gut contribute to the vitelline circulation, and as the liver cords proliferate, the portal circulation is established [70, 71]. Although evident until week 12 of development, the yolk sac begins regressing around week 10, with progressive evidence of tissue degeneration and with loss of blood flow through the yolk sac vascular network [67, 72].
Many twentieth century investigators using microscopic analysis of tissue sections of human embryonic tissues described the sequence of hematopoietic development that occurs in the human embryo and fetus [73–75]. During human development in utero, blood cells first arise in the yolk sac between days 16 and 19, followed sequentially by fetal liver hematopoiesis by weeks 5 to 6 and engraftment and expansion of hematopoietic cells in developing long bones by weeks 8 to 19 (e.g., most bones become hematopoietic between weeks 11 and 12) [76, 77]. The liver functions as the primary site of hematopoiesis from weeks 6 through 22 of gestation until the bone marrow becomes the lifelong predominant site of blood cell production. Distinct patterns of blood cell differentiation and proliferation occur in each specific site of hematopoiesis during ontogeny [78, 79].
The most extensive morphologic analysis of hematopoiesis in the developing human was reported in a 1979 monograph by Kelemen and colleagues [76]. These investigators studied tissue sections from 190 human embryos of between 3 and 28 weeks of gestation. Hematopoietic cells were first observed in the human embryo at day 18 in the mesenchymal layer of the secondary yolk sac. Most blood islands were composed of hematopoietic cells completely surrounded by endothelial cells, but some extravascular free aggregates of blood cells were present in the mesenchyme close to the visceral endoderm. The 3- to 4-week embryo had more defined vessels, many of which were empty of hematopoietic cells. Frequently, small clusters of undifferentiated cells called hemocytoblasts (equivalent to the hemangioblast in the mouse) associated with the endothelium protruded into the vessel lumen. Concomitantly, clusters of primitive erythroblasts (>20 μm cells with central to eccentric nucleus and orthochromatic cytoplasmic staining) began to fill capillaries and larger vessels (e.g., vitelline, umbilical, chorionic, and amniotic). Wherever the number of primitive erythroblasts predominated in vessels, few hemocytoblasts were detectable. This was a surprising finding, because the erythroblasts had previously been thought to originate from the hemocytoblasts [48]. At the sixth week of development, intraembryonic and extraembryonic blood vessels became macroscopically visible, and definitive erythroblasts (<20 μm cells with condensed nuclei and abundant hemoglobin cytoplasmic staining) and primitive erythroblasts could be identified in the yolk sac vessels. The only other mature blood cell in the yolk sac was the macrophage. In contrast to normal fetal liver and bone marrow patterns of differentiation, macrophages in the yolk sac arose without evidence of a monocytic precursor. An overall decline in hematopoiesis was observed in the human yolk sac after the eighth week of gestation.
Hematopoiesis occurred in the liver by the fifth to sixth week of gestation. The establishment of the systemic circulation (weeks 4 to 5) had just commenced before liver hematopoiesis at week 5. The predominant blood cell observed in the large vessels and in developing liver sinusoids was the primitive erythroblast. Over the next 4 weeks, there was a shift away from the primitive to the definitive erythroblast. Mature enucleated red blood cell production in the fetus was associated with a 40-fold increase in the total liver mass, with hematopoietic cells comprising 60% of the total cell number (weeks 11 to 12). Macrophages, megakaryocytes, granulocytes, and lymphocytes also were identifiable during weeks 5 through 22. Macrophages were the predominant mature blood cell type identified early in liver hematopoiesis (weeks 5 to 6), but the concentration of macrophages diminished thereafter. Megakaryocytes were present throughout the liver phase of hematopoiesis, but a notable shift from megakaryocytes with one to two nuclei to megakaryocytes with four or more nuclei occurred as liver hematopoiesis diminished (weeks 18 to 21) and bone marrow hematopoiesis began to flourish. The few granulocytes were restricted to the connective tissue of the hepatic portal area from weeks 5 through 15. A significant increase in granulocytes was observed beyond week 21 of development, coinciding with enhanced hematopoiesis in the bone marrow. Lymphocytes were sparse until week 7 of development, and even through week 13, they remained a minor population (1% of blood cells) in the liver. It was not evident whether these lymphocytes were of the B or T cell lineage, but the thymus did not have lymphocytes until week 9. The percentage of hemocytoblasts (presumed stem cells) was high (10% of blood cells) in the 5- to 6-week embryo liver but declined for the remainder of the liver phase of hematopoiesis.
The earliest bone marrow sample in which hematopoietic cells were present was taken from an embryo at week 8 of development. The predominant cell type at 8 to 9 weeks was the primitive erythroblast. Definitive erythroblast production increased greatly from weeks 11 through 14, and by 14 weeks, nearly all erythroblasts were definitive types. It was speculated that primitive erythroblasts were presented to the marrow by means of the systemic circulation and that only definitive erythroblasts were produced in the marrow. Erythrocytic cells constituted about 35% of total marrow cells by week 12 and 20% to 30% thereafter. At weeks 8 to 9 of embryonic development, granulocytes were observed in the marrow of long bones. Neutrophilic granulocytes composed 30% to 40% of total marrow cells between weeks 10 and 13. By 21 weeks, neutrophilic granulocytes were the major blood cell produced, representing nearly 60% of hematopoietic cells in the marrow. Eosinophilic granulocytes (1%) were present in the marrow of embryos at week 10 and gradually increased to nearly 5% of total marrow cells by week 21. Basophilic granulocytes were few in the 10-week embryo and remained low (0.3%) even in the 28-week embryo. Macrophages were most numerous in the embryonic marrow at weeks 10 through 16, whereas monocytes peaked between weeks 12 and 16. Lymphocytes increased from 12% of total marrow cells at week 12 to 20% to 30% in older embryos. Megakaryocytes were present in all samples examined. The percentage of immature “blast cells,” which probably represented hematopoietic stem and progenitor cells, varied from 15% at weeks 12 through 13 to 1% to 4% at weeks 21 through 23. Whereas erythropoiesis exceeded granulocytopoiesis in the 10- to 11-week-old embryo (erythropoiesis/granulopoiesis ratio of 0.8), granulopoiesis predominated by week 12 and reached a nearly adult ratio of 3 (1.8 to 3.3) by the twenty-first week of human development.
Splenic hematopoiesis in the human embryo has been difficult to prove. At 8 weeks of gestation, the spleen is composed primarily of a meshwork of mesenchymal cells with some primitive erythroblasts contained in the blood vessels [76]. Few macrophage and neutrophil precursors were present in the spleen at 8 weeks. The hematopoietic cells in the spleen were dispersed throughout, but most erythroblasts were confined to the vascular system. This distribution of hematopoietic cells in the spleen contrasted with the same-stage embryo liver in which the developing blood cells were confined to focal sites of hematopoiesis. Similar observations were made by other investigators [80], and these results raised the question of whether the hematopoietic cells observed in the embryonic spleen were circulating blood cells or were cells produced in the spleen [80].
Calhoun and coworkers used several methods to determine whether the human mid-gestation spleen (13 to 22 weeks’ gestation) was an active site of hematopoiesis [81]. Liver, spleen, and bone marrow samples were examined by histology, and cell suspensions from each site were prepared for enumeration of mature blood cells and their immediate precursors. Lymphocytes, macrophages, and erythroid cells predominated at 13 weeks’ gestation. Neither mature nor immature neutrophils were identified in the spleen until 16 weeks’ gestation. Throughout the study period, active foci of hematopoiesis were present in the liver and bone marrow but never in the spleen. Because the distribution of blood cells observed for the splenic suspensions was similar to the blood cell concentrations previously reported after fetal blood sampling [82], these results in total suggest that the mid-gestation human spleen is not normally an active hematopoietic organ [81].
Numerous investigators extended these morphologic observations by examining the onset of human hematopoiesis by means of functional assays of hematopoietic CFU-C formation and by using cell-surface antigen detection to identify specific hematopoietic lineages (i.e. monoclonal antibodies with immunohistochemical or FACS analysis). Although many studies have focused on human fetal liver and marrow hematopoiesis, little is known about the biology of hematopoietic stem and progenitor cells that arise in the human yolk sac. The data reviewed indicate that the transient population of yolk sac hematopoietic cells differs in many aspects from hematopoietic cells produced within the embryo proper or in the liver or bone marrow.
Migliaccio and colleagues performed a careful examination of the kinetics of yolk sac CFU-C in human embryos from 4.5 to 10 weeks’ gestation [83]. This stage encompassed the period of yolk sac hematopoietic decline and initiation of liver hematopoiesis. CFU-E and BFU-E were abundant in the yolk sac of the 4.5-week embryos. CFU-GMs were also present in high concentration. At 5 weeks, the liver contained abundant primitive erythroblasts and some macrophages in the hepatic sinusoids. The concentrations of CFU-E, BFU-E, and CFU-GM in the liver rose rapidly over the next 3 weeks and reached a plateau by 8 to 9 weeks of gestation. Although definitive erythropoiesis (i.e. CFU-E and BFU-E) predominated for the remainder of the fetal liver phase of hematopoiesis, CFU-GMs increased from week 8 through 12 and remained stable until almost 20 weeks, when the number of myeloid progenitor cells declined [84]. Concomitant with the changes in progenitor cell concentrations, the percentage of circulating and tissue-restricted mature blood cells of the myeloerythroid lineages changed as hematopoiesis shifted from the yolk sac to the liver. A gradual shift from primarily embryonic to primarily fetal and adult hemoglobin molecules also occurred in yolk sac and liver BFU-E-derived red blood cells respectively, during the fifth through eighth weeks of gestation [85].
Investigators have also attempted to isolate and enrich populations of hematopoietic stem and progenitor cells from the developing human embryo. Through the use of monoclonal antibodies and FACS, a stem cell-surface “phenotype” has emerged that is useful in isolating hematopoietic progenitor and putative hematopoietic stem cells [86]. Because numerous monoclonal antibodies may recognize the same cell-surface antigen, an international classification is assigned to each monoclonal antibody; all monoclonal antibodies reacting with a single blood cell-surface antigen are given a cluster of differentiation (CD) number designation [87].
The CD34 antigen is one that has been used extensively in the positive selection of human adult hematopoietic stem and progenitor cells [88]. CD34, a cell-surface sialomucin, is also expressed on human fetal liver hematopoietic cells; more CD34 is expressed on the fetal hematopoietic progenitor cells than on adult hematopoietic progenitor cells [89]. The cell-surface phenotype that permits the highest enrichment of committed and primitive hematopoietic progenitor cells is found in embryonic and fetal hematopoietic cells that do not express any mature blood cell lineage antigens (lin−), CD38 (a transmembrane glycoprotein), or CD71 (transferrin receptor) but express CD4, CD34, CD117 (KIT tyrosine kinase receptor), and CD90 (Thy-1 antigen) [89–91]. Other antigens that are more controversial with regard to enriching for hematopoietic stem and progenitor cell activity in the embryo and fetus include expression of CD15 (3-fucosyl-N-acetyl-lactosamine), and human major histocompatibility complex class II antigen (HAD-DR)[90].
The CD34+ hematopoietic progenitor cells have been identified as early as day 23 of human gestation in the yolk sac and within the embryo proper [92]. The intraembryonic CD34+ cells were localized to the ventral aspect of the dorsal aorta around the peri-umbilical region, or the AGM. The hematopoietic clusters were in the luminal side in tight association with the endothelial cells [92]. When tissue explants of the peri-umbilical aortic region, liver, heart, limbs, blood, and umbilical cord of embryos at days 30 through 40 of gestation were co-cultured in vitro with a murine bone marrow stromal cell line, fivefold more hematopoietic progenitors were derived from the peri-umbilical aortic region than from all other sites combined [92]. Additionally, long-term hematopoietic engraftment was achieved by transplanting cells from the AGM into immunodeficient mice [93]. These results confirmed the presence of hematopoietic progenitor cells in the human embryo at sites distinct from the yolk sac and liver and more recently have defined vascular endothelium as the cells giving rise to the hematopoietic clusters [94, 95]. Huyhn and coworkers isolated yolk sac, liver, and embryonic tissues from 25 to 40-day-gestation embryos and plated the enzyme-disaggregated samples in methylcellulose cultures for detection of hematopoietic progenitor cells [96]. Although the concentration of BFU-E in the yolk sacs and embryo proper were similar, 7–12-fold higher numbers of CFU-GM were present in the embryo proper than in the yolk sac. These results were interpreted as evidence that intraembryonic hematopoietic precursors occur in high concentrations when the liver is initiating hematopoiesis.
In addition to differences in progenitor cell concentration in the 25- to 50-day yolk sac, liver, and embryo samples, distinct differences in the biology of the hematopoietic progenitors were also observed. Yolk sac BFU-Es were distinct from liver and embryo proper-derived BFU-Es by being larger and containing dispersed myeloid cells on microscopic examination. It appeared that the yolk sac BFU-Es were more primitive (i.e. containing erythroid and myeloid progenitor activity) than typical adult marrow-derived BFU-Es [12]. Dose-response curves analyzing the in vitro growth factor responsiveness of the progenitor cells did not indicate any difference in the growth factor sensitivity of progenitors from these sites [96]. The CFU-E concentrations were low in the yolk sac and embryo proper but were abundant in the liver. These CFU-E results contrast with the findings of Migliaccio and coworkers, who observed high numbers of yolk sac CFU-Es in the 4.5- to 5-week human embryonic yolk sac [83]. The discrepant results have not been resolved.
The yolk sac and embryo proper are known to be composed of hematopoietic cells demonstrating HPP-CFC activity. The CD34+ hematopoietic cells recovered from the yolk sac and embryo yielded 45 HPP-CFC and 20 HPP-CFC, respectively, with 10,000 CD34+ cells were plated with specific combinations of growth factors [96]. All CD34+ progenitors and HPP-CFC in the yolk sac and embryo proper failed to express CD38 or CD33, consistent with the pattern of expression observed for adult marrow hematopoietic stem cells.
The LTC-IC concentration is reported to be higher in fetal liver CD34+CD38− cells than in phenotypically similar adult marrow cells, and the number of secondary colonies derived from cultured LTC-IC was sevenfold higher in fetal liver compared with adult marrow CD34+CD38− cells [97]. Hematopoietic stem cells from the livers of 12- to 15-week gestation human fetuses can engraft and produce circulating blood cell progeny for more than 2 years after in utero transplantation into preimmune fetal sheep [18, 98]. Human fetal liver cells can also engraft and repopulate blood cell lineages in human patients with a variety of hematopoietic disorders [99, 100].
No published reports have characterized the biology of hematopoietic stem and progenitor cells in the human late embryonic and early fetal bone marrow. Several groups have isolated enriched populations of hematopoietic cells from the bone marrow (e.g. tibias and femurs) of human fetuses at 12 to 24 weeks’ gestation. Turner and colleagues compared in vivo repopulating ability of CD34+ cells isolated from fetal bone marrow, adult bone marrow, and mobilized peripheral blood cells (e.g. patients treated for 5 to 7 days with recombinant growth factors and blood cells collected by apheresis)[101]. The fetal marrow cells expanded to constitute 23% of the total number of marrow and spleen cells in 15% of the recipient mice, whereas adult marrow cells only engrafted in 2% of the recipients. Human peripheral blood cells engrafted in 3% of the mice but only in the T lymphocyte lineage. The investigators concluded that fetal marrow appears superior to adult marrow and mobilized peripheral blood in engrafting in this xenograft model and that human hematopoiesis can occur in this model without the administration of human cytokines to recipient mice. One factor that may have contributed to the high level of fetal marrow engraftment in the mice was that 18- and 9-fold more CD34+ cells were recovered in the fetal marrow than in the mobilized peripheral blood and adult bone marrow, respectively.
While the use of human embryonic hematopoietic stem cells has not been widely applied for therapeutic transplantation, human umbilical cord blood has emerged as an effective alternative to adult bone marrow as a source of transplantable stem cells [102–106]. Human embryonic stem (hES) cell-derived hematopoietic stem cells has been proposed as an emerging hopeful inexhaustible alternative to all current sources of stem cells, though progress in defining full multilineage engraftment from a hES cell has been elusive [107–110]. Most encouraging are the increasing amounts of literature on reprogramming human somatic cells to pluripotent stem cells [111–113]. Indeed, recent work has demonstrated that human pluripotent stem cells can be differentiated into human hematopoietic stem and progenitor cells and that adult murine endothelial cells may be directly programmed into hematopoietic stem cells [114, 115]. These exciting developments may provide novel approaches to treating human disease, but more work is needed to understand the molecular regulation of hematopoietic stem cell development, and as such, both great challenges and life-altering opportunities lie ahead.
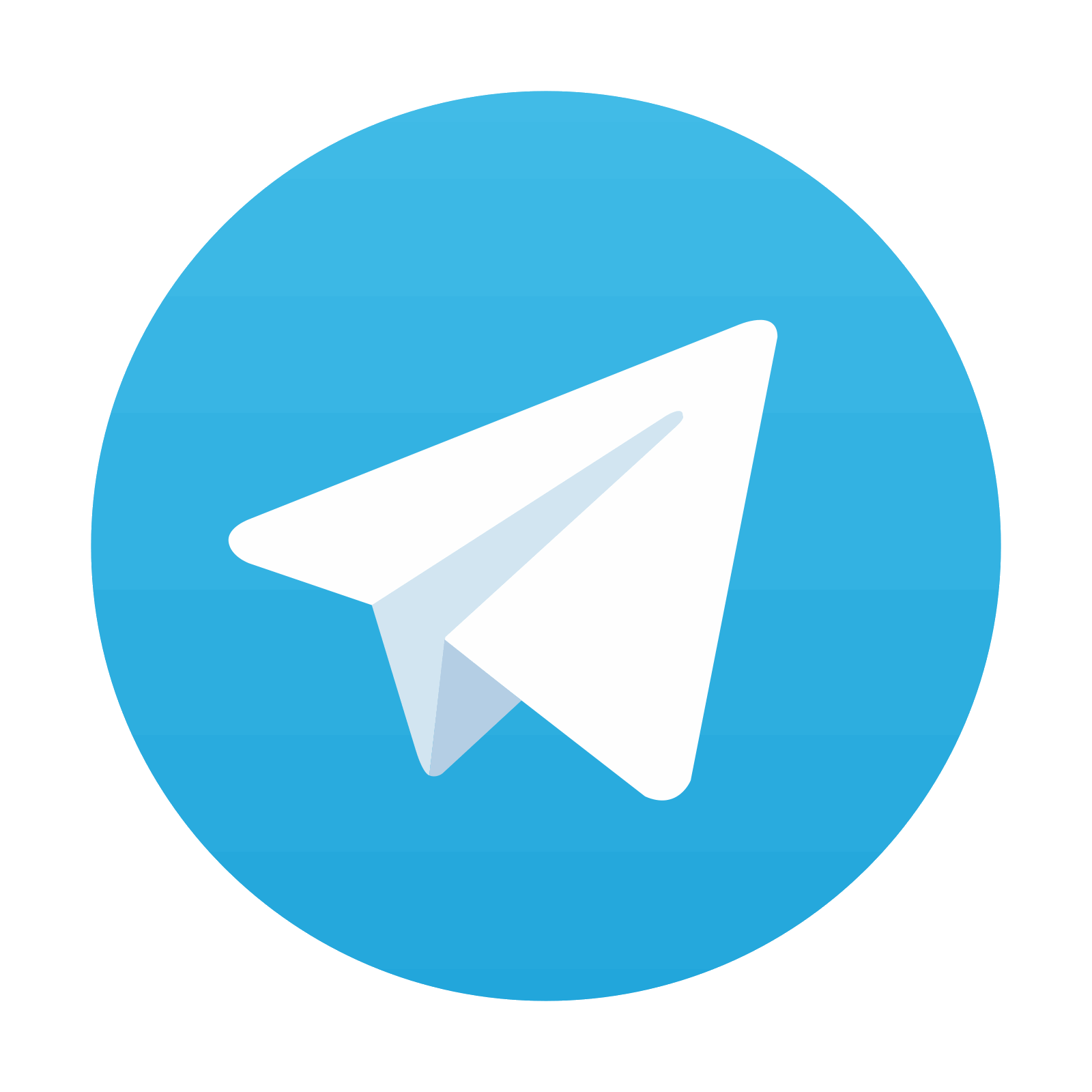
Stay updated, free articles. Join our Telegram channel
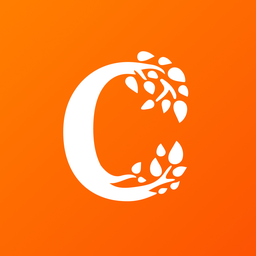
Full access? Get Clinical Tree
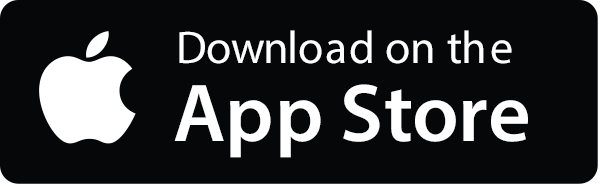
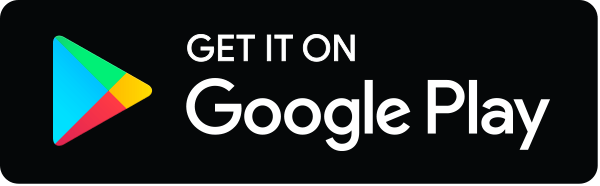
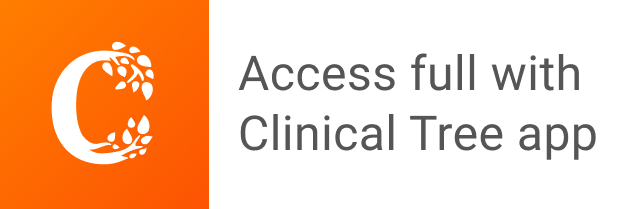