Cancer Metabolism
Matthew G. Vander Heiden
One of the first distinctions noted between cancer tissues and normal tissues was a difference in metabolism. In the 1920s, the biochemist Otto Warburg observed that when provided with glucose, cancer tissues generate large amounts of lactate regardless of whether oxygen is present. This finding is in contrast to most normal tissues that only ferment glucose to lactate in the absence of oxygen. This metabolic difference between cancer cells and normal cells is referred to as the Warburg effect, and along with other metabolic alterations that characterize cancer cells, it remains an incompletely understood aspect of cancer biology. The metabolic phenotype of cancer cells has been exploited for cancer diagnostics and led to the development of some of the first successful chemotherapies. However, despite the fact that altered metabolism is shared across many different cancer types, few therapies exist that exploit differences in cellular metabolism. Efforts to understand cancer metabolism are currently an active area of investigation and hold great promise as a source of novel targets for cancer treatment.
ALTERED METABOLISM IN CANCER CELLS
The regulation of metabolic pathways in tumor tissues is different from that observed in most adult tissues.1 Rapidly dividing cells must balance energy production with macromolecular synthesis, while most nonproliferating adult tissues utilize a greater fraction of nutrients for energy production and require less nutrient uptake. Cancer cells rely primarily on glycolysis for their metabolism, while the majority of normal cells in adult tissues utilize aerobic respiration to completely catabolize glucose and generate cellular energy.2,3,4,5 Most differentiated cells primarily metabolize glucose to carbon dioxide in the presence of oxygen and only produce large amounts of lactate under anaerobic conditions. Warburg observed that cancer cells produce large amounts of lactate regardless of oxygen availability (Fig. 8.1).6,7 Because cancer cells use glycolysis to make lactate from glucose in the presence of oxygen, this form of metabolism observed in cancer cells is also called aerobic glycolysis.
The primary energy source for cells in most tissues is glucose. The concentration of glucose in the blood remains relatively constant at around 4-6 mM (72-110 mg/dL). Glucose uptake into cells is controlled most proximally by the expression of glucose transport proteins on the cell surface (Fig. 8.2).8 Insulin-responsive tissues rely on the regulated delivery of Glut4 transporters to the cell surface to increase glucose uptake. Noninsulin responsive tissues, including most cancers, do not use Glut4, but instead use the homologous Glut1, Glut2, or Glut3 proteins to transport glucose into cells. All of these glucose transporters allow the diffusion of glucose across the plasma membrane. The transporters differ in their affinity for glucose and capacity for transport. Glut1 is responsible for the basal level of glucose uptake in most normal cells and is thought to be the transporter responsible for glucose uptake in most tumor cells. Expression of Glut3 and other less well characterized glucose transporters have also been described in some cancers.9,10 How these transporters differ from Glut1 and whether these differences are important for tumor biology and metabolism remain active areas of investigation.
Glucose is trapped in the cytoplasm of cells by the addition of a phosphate group to form glucose-6-phosphate (Fig. 8.2).11 This reaction is catalyzed by the enzyme hexokinase, which also has several isoforms with different normal tissue distributions and enzymatic properties. The various isoforms of hexokinase can associate with the outer surface of mitochondria, and this proximity to a source of adenosine triphosphate (ATP) has been suggested to be important for the high rate of glucose uptake observed in many cancer cells.12 The association of hexokinase with mitochondria has also been implicated in the regulation of apoptosis such that hexokinase may constitute a molecular link between glycolysis and the cell death machinery.13
Once trapped in cells, glucose can be metabolized via glycolysis to generate pyruvate in the cytosol.11 The rate of glycolysis is controlled by glucose flux into cells, cofactor availability, and the activity of glycolytic enzymes (Fig. 8.3). Both the phosphofructokinase and pyruvate kinase steps of glycolysis are highly regulated and have been implicated in the control of tumor cell metabolism
(Figs. 8.3 and 8.4).14,15,16 Another major determinant of glucose metabolism by glycolysis is the availability of oxidized nicotinamide adenine dinucleotide (NAD) to serve as the electron acceptor for the conversion of glyceraldehyde-3-phosphate to 1,3-bisphosphoglycerate. This reaction, catalyzed by glyceraldehyde-3-phosphate dehydrogenase reduces NAD to NADH (reduced nicotinamide adenine dinucleotide). Because the size of the NAD/NADH cofactor pool in cells is small relative to the flux of glucose through glycolysis, continued cycling of NADH back to NAD is critical to permit continued glycolysis (Fig. 8.3). NADH can be reoxidized to NAD through a series of reactions that shuttle reducing equivalents into mitochondria for use in oxidative phosphorylation. This process is coupled to the further metabolism of pyruvate in the mitochondrial tricarboxylic acid (TCA) cycle and can result in the generation of large amounts of ATP. ATP is used as a source of free energy for cells to enable otherwise unfavorable biochemical processes. Mitochondrial oxidative phosphorylation requires the presence of oxygen (O2) as the final acceptor of electrons from NADH and therefore is also referred to as
aerobic respiration. Aerobic glycolysis also generates ATP; however, the ATP yield per molecule of glucose is much less than for aerobic respiration. The metabolism of glucose to pyruvate without mitochondrial respiration requires the enzyme lactate dehydrogenase (LDH) to produce lactate and regenerate NAD from NADH.
(Figs. 8.3 and 8.4).14,15,16 Another major determinant of glucose metabolism by glycolysis is the availability of oxidized nicotinamide adenine dinucleotide (NAD) to serve as the electron acceptor for the conversion of glyceraldehyde-3-phosphate to 1,3-bisphosphoglycerate. This reaction, catalyzed by glyceraldehyde-3-phosphate dehydrogenase reduces NAD to NADH (reduced nicotinamide adenine dinucleotide). Because the size of the NAD/NADH cofactor pool in cells is small relative to the flux of glucose through glycolysis, continued cycling of NADH back to NAD is critical to permit continued glycolysis (Fig. 8.3). NADH can be reoxidized to NAD through a series of reactions that shuttle reducing equivalents into mitochondria for use in oxidative phosphorylation. This process is coupled to the further metabolism of pyruvate in the mitochondrial tricarboxylic acid (TCA) cycle and can result in the generation of large amounts of ATP. ATP is used as a source of free energy for cells to enable otherwise unfavorable biochemical processes. Mitochondrial oxidative phosphorylation requires the presence of oxygen (O2) as the final acceptor of electrons from NADH and therefore is also referred to as
aerobic respiration. Aerobic glycolysis also generates ATP; however, the ATP yield per molecule of glucose is much less than for aerobic respiration. The metabolism of glucose to pyruvate without mitochondrial respiration requires the enzyme lactate dehydrogenase (LDH) to produce lactate and regenerate NAD from NADH.
![]() FIGURE 8.2 Glucose uptake is controlled in mammalian cells by the presence of glucose transporters on the cell surface (Glut). These transport proteins allow the diffusion of glucose across the plasma membrane where it is phosphorylated by the enzyme hexokinase (HK) and trapped in the cell. Glucose transporter expression is controlled by insulin signaling in insulin responsive tissues. Glucose uptake is also regulated by cell growth signals. A positron emission tomography (PET) scan can be used to monitor glucose uptake in the clinic. This assay uses the positron-emitting fluorine-18 (18 F)-conjugated glucose analogue fluoro-2-deoxyglucose (FDG), which can be phosphorylated by hexokinase and trapped in the cell but cannot be metabolized further. |
Several hypotheses for why aerobic glycolysis appears to be selected for in cancer cells have been proposed. Warburg hypothesized that cancer cells develop a defect in mitochondria that leads to impaired aerobic respiration and a subsequent reliance on glycolytic metabolism.2 Although mutations in mitochondrial enzymes have been implicated in a subset of cancers (discussed in detail later in the chapter), subsequent studies demonstrated that mitochondrial function is not impaired in the majority of cancer cells.17 Nevertheless, despite his hypothesis being incorrect, Warburg’s original observation has held true with numerous reports describing that despite normally functioning mitochondria many cancer cells preferentially metabolize glucose via aerobic glycolysis.4,18
The growth of solid tumors is limited by the presence of an adequate blood supply to deliver oxygen and nutrients to support cell metabolism. Therefore, angiogenesis is an important process for tumor growth, and targeting this process has been successful for cancer therapy.19 Because many tumors are characterized by inefficient angiogenesis, cells must survive periods of relative hypoxia and nutrient deprivation during tumorigenesis.5 Therefore, it has been proposed that the relative hypoxia of tumors selects for glycolytic metabolism.3 However, aerobic glycolysis is observed at the earliest stages of tumorigenesis. It is a characteristic feature of leukemia and lung cancers that arises under conditions of normal to high oxygen tensions and is found in normal rapidly proliferating tissues during embryogenesis and immune responses.1 Therefore, it is likely that aerobic glycolysis provides another benefit to cancer cells that is selected for during tumorigenesis.1 Aerobic glycolysis may still facilitate tumor survival during periods of hypoxia. The same pathways that regulate angiogenesis also promote aerobic glycolysis, suggesting that important connections between these two processes exist.20,21
The selective pressure for aerobic glycolysis in cancer cells may be related to the reprogramming of metabolism to accommodate rapid cell division. Cells metabolize glucose for purposes other than generating ATP.1 Intermediates derived from the metabolism of glucose are used in other metabolic pathways in cells and ultimately provide much of
the carbon necessary to produce biomass. The production of nucleic acids, amino acids, lipids, and carbohydrates needed to duplicate all the components of the dividing cell is the major metabolic requirement that distinguishes rapidly proliferating cancer cells from most normal cells. If glucose is completely catabolized to carbon dioxide (CO2), as occurs during oxidative metabolism, there are no metabolic intermediates available for biosynthetic reactions. Thus, aerobic glycolysis may reflect how metabolism is altered to permit anabolic metabolism.1 Indeed, many micro-organisms grow by fermentation when nutrients are abundant and display a metabolic phenotype analogous to aerobic glycolysis.22
the carbon necessary to produce biomass. The production of nucleic acids, amino acids, lipids, and carbohydrates needed to duplicate all the components of the dividing cell is the major metabolic requirement that distinguishes rapidly proliferating cancer cells from most normal cells. If glucose is completely catabolized to carbon dioxide (CO2), as occurs during oxidative metabolism, there are no metabolic intermediates available for biosynthetic reactions. Thus, aerobic glycolysis may reflect how metabolism is altered to permit anabolic metabolism.1 Indeed, many micro-organisms grow by fermentation when nutrients are abundant and display a metabolic phenotype analogous to aerobic glycolysis.22
ENERGETICS OF CELL PROLIFERATION
There is evidence that the rate of aerobic glycolysis, including the accompanying increased rate of glucose utilization, is not elevated in cancer cells solely to satisfy ATP demand. It has been suggested that glycolysis in tumors cells is limited by the rate of ATP consumption.23,24 Cancer cells must balance the catabolism of nutrients to generate ATP with other metabolic needs to allow net biosynthesis and cell proliferation.1 To produce a daughter cell, a proliferating cell must replicate the genome, duplicate the ribosomes and protein synthesis machinery, generate new organelles, and synthesize de novo enough lipids to duplicate cellular membranes. This imposes a large requirement of new nucleic acids, amino acids, and lipids for cell proliferation. While ATP hydrolysis provides free energy for many biosynthetic pathways, there are additional requirements to carry out these anabolic reactions. Synthesis of lipids and nucleic acids both require specific metabolite precursors and reducing equivalents provided by nicotinamide adenine dinucleotide phosphate (NADPH) (Fig. 8.5). In fact the need for NADPH and carbon skeletons on a molar basis exceeds the requirement for ATP in many biosynthetic reactions.1 Aerobic glycolysis may allow cancer cells to balance the various metabolic requirements of proliferation.
Cancer Cells Can Metabolize Nutrients Other Than Glucose
Although tumor cell metabolism is adapted to facilitate anabolic metabolism for rapid proliferation, it is not clear why many cancer cells excrete lactate. Each lactate excreted wastes three carbons that might otherwise be recycled to fulfill some need in building a new cancer cell. It has been hypothesized that the excretion of lactate, which accompanies aerobic glycolysis, may enable faster proliferative metabolism.1 In the early stages of tumorigenesis, cancer cells are not limited for nutrients. Cells that incorporate nutrients into biomass most efficiently will proliferate faster. Lactate production may provide other advantages to a tumor as well. Acidification of the tumor microenvironment has been shown to promote invasion and metastasis.3 Lactate can also act as a nutrient for some cells in the tumor.25 As tumors grow, cells in the less-well vascularized regions of a tumor can utilize lactate from neighboring cells as a carbon source to survive periods of cell stress.26
Despite an increased reliance on aerobic glycolysis, most tumor cells continue to metabolize at least some nutrients by oxidative phosphorylation. In fact, it is likely that in some cancer cells oxidative phosphorylation continues to generate much of the ATP required for biosynthetic reactions and cellular housekeeping functions.4 Attempts to quantitate ATP production experimentally in cancer cells have estimated that 80% of the ATP generated is derived from oxidative pathways, while 20% comes from glycolysis,27 and others report that up to 50% of the ATP in cancer cells can be derived from oxidative phosphorylation.28
Glucose is not the only substrate used by cancer cells for oxidative phosphorylation.27,29 In fact, some human cancers do not demonstrate elevated glucose uptake. It has been proposed that at least some of these cancers may be dependent on the amino acid glutamine as a primary source of carbon. Glutamine can be metabolized via two transamination reactions to the TCA cycle intermediate α-ketoglutarate (Fig. 8.4), and glutamine is required for proliferation of many cancer cells.30
In addition to glucose and glutamine, other nutrients have been shown to be important in some tumors. Several clinical studies have demonstrated that some human cancers show increased uptake of acetate by positron emission tomography (PET) scan using carbon-11 (11C)-acetate as a tracer (see below).31 Acetate can be converted to acetyl-coA and serve as a precursor for lipid synthesis.32 When production of lipids from glucose is blocked by inhibiting ATP citrate lyase, an enzyme required to convert glucose to cytosolic acetyl-coA, acetate can completely rescue lipid synthesis and cell growth. Acetate is not thought to be a major nutrient available to cancer cells in patients, but may reflect an increased dependence of some cancer cells on lipid metabolism. Other enzymes involved in lipid metabolism have been linked with cancer progression,33,34 however, the exact mechanisms by which lipid metabolism promotes malignancy are not clear.
In addition to glucose and glutamine, other nutrients have been shown to be important in some tumors. Several clinical studies have demonstrated that some human cancers show increased uptake of acetate by positron emission tomography (PET) scan using carbon-11 (11C)-acetate as a tracer (see below).31 Acetate can be converted to acetyl-coA and serve as a precursor for lipid synthesis.32 When production of lipids from glucose is blocked by inhibiting ATP citrate lyase, an enzyme required to convert glucose to cytosolic acetyl-coA, acetate can completely rescue lipid synthesis and cell growth. Acetate is not thought to be a major nutrient available to cancer cells in patients, but may reflect an increased dependence of some cancer cells on lipid metabolism. Other enzymes involved in lipid metabolism have been linked with cancer progression,33,34 however, the exact mechanisms by which lipid metabolism promotes malignancy are not clear.
Nutrients such as amino acids and iron are also important for some tumors.35,36 Cachexia, or the loss of body mass that cannot be reversed by increased nutrition, is associated with the late stages of some cancers. Cachexia is poorly understood, but in cancer patients it is characterized by the loss of adipose tissue and muscle mass.37 This may reflect a derangement in whole body metabolism to supply specific nutrients to the cancer. Understanding how nutrients other than glucose contribute to cancer biology remains an active area of investigation.
IMAGING CANCER METABOLISM IN PATIENTS
The characteristic increased glucose uptake of cancer cells has been exploited to image cancer in the clinic. The glucose analogue 2-deoxyglucose is permeable to glucose transporters and trapped in cells when phosphorylated by hexokinase (Fig. 8.2). The 2-deoxyglucose-6-phosphate is unable to be further metabolized,38,38 and thus 2-deoxyglucose-6-phosphate accumulation can be used to assess the rate of glucose uptake into cells. By conjugating the positron emitting isotope fluorine-18 (18F) to 2-deoxyglucose, the uptake of glucose can be measured in patients using PET.40 18F-fluro-2-deoxyglucose (FDG)-PET is used widely in the clinic to visualize tumors. The current use of FDG-PET is primarily as a staging tool for cancers, however, it is also sometimes used to characterize lesions observed by other imaging modalities. FDG-PET is also increasingly being used as a marker of response to therapy (Fig. 8.6). At least for some treatments, there is mounting evidence that decreased uptake of FDG by PET scan following therapy is a predictor of clinical efficacy.41,42
In the clinic, FDG-PET scan can be used to classify tumors. Although many tumors are visible by FDG-PET scan, some tumors do not display elevated FDG uptake. This illustrates that different tumors can display distinct metabolic phenotypes related to their genetic background or site of origin. As discussed above, some tumors rely on nutrients other than glucose. For instance increased 11C-acetate uptake has been observed by PET in prostate tumors that do not demonstrate elevated signal on FDG-PET scan.43 However, increased uptake of 11C-acetate is also seen in some benign prostate conditions, so whether 11C-acetate uptake defines a characteristic of some prostate cancers or reflects the underlying biology of the prostate gland remains to be determined.44 New methods to image tumor metabolism by PET or magnetic resonance imaging (MRI) are being developed. If successful, such efforts will better define distinct metabolic phenotypes in patient tumors.
GENETIC EVENTS IMPORTANT FOR CANCER INFLUENCE METABOLISM
Human cancer occurs as a consequence of genetic events that promote the inappropriate proliferation of cells.45 These events lead to the expression of oncogenes or the loss of tumor suppressor genes that contribute to tumor formation and progression. Although alterations involving specific oncogenes or tumor suppressor genes are hallmarks of specific malignant phenotypes, many of these genetic events are found in numerous types of cancer. These genetic changes occur in diverse cellular signaling and transcriptional pathways, and it is unclear how the various mutations converge to allow inappropriate cell growth and proliferation. One common downstream consequence of these genetic changes is altered cellular metabolism.
Efforts to understand the predisposition to malignancy displayed by von Hippel-Lindau (VHL) syndrome patients identified a link between cancer genetics and the regulation of cell metabolism.46 VHL syndrome results when patients inherit one mutated copy of the VHL gene, leading to a spectrum of benign and malignant tumors including clear cell carcinoma of the kidney. In these patient’s tumors, the normal VHL allele is lost, consistent with VHL acting as a tumor suppressor gene. Subsequent work demonstrated that the VHL gene product is also commonly lost in sporadic renal clear cell carcinomas. Although the exact mechanism by which VHL loss leads to renal cell carcinoma and other tumors is not known, loss of VHL has a profound impact on metabolic gene regulation, and these effects are thought to be important in the pathogenesis of these cancers.
The VHL gene encodes the substrate recognition component of a ubiquitin E3 ligase.46 As an E3 ligase, the VHL protein-containing complex
facilitates the transfer of ubiquitin to specific target proteins to effect their degradation. Among the targets of the VHL protein is the hypoxia-inducible transcription factor-1α (HIF-1α) (Fig. 8.7). HIF-1α levels are regulated by oxygen tension.21,47 In the presence of oxygen HIF-1α is hydroxylated. The hydroxylated form of HIF-1α is recognized by VHL, leading to ubiquitination and degradation of the protein. When oxygen is absent, or when VHL is lost, HIF-1α protein accumulates, dimerizes with HIF-1α, and promotes the transcription of a number of hypoxia inducible genes. These genes include factors that promote angiogenesis to improve tissue blood supply as well as glucose transporters and most of the enzymes in glycolysis. Thus, inappropriate HIF-1α activation leads to the characteristic increased glucose uptake and glycolysis that is observed in cancer. HIF-1α accumulation is a direct consequence of VHL loss, providing a link between the loss of a tumor suppressor gene and the altered metabolic phenotype of malignant cells.
facilitates the transfer of ubiquitin to specific target proteins to effect their degradation. Among the targets of the VHL protein is the hypoxia-inducible transcription factor-1α (HIF-1α) (Fig. 8.7). HIF-1α levels are regulated by oxygen tension.21,47 In the presence of oxygen HIF-1α is hydroxylated. The hydroxylated form of HIF-1α is recognized by VHL, leading to ubiquitination and degradation of the protein. When oxygen is absent, or when VHL is lost, HIF-1α protein accumulates, dimerizes with HIF-1α, and promotes the transcription of a number of hypoxia inducible genes. These genes include factors that promote angiogenesis to improve tissue blood supply as well as glucose transporters and most of the enzymes in glycolysis. Thus, inappropriate HIF-1α activation leads to the characteristic increased glucose uptake and glycolysis that is observed in cancer. HIF-1α accumulation is a direct consequence of VHL loss, providing a link between the loss of a tumor suppressor gene and the altered metabolic phenotype of malignant cells.
![]() FIGURE 8.6 Early metabolic changes on 18F-fluro-2-deoxyglucose position emission tomography/computed tomography (FDG-PET/CT) are highly predictive of final treatment response. Fused coronal FDG-PET/CT images in a 44-year-old woman with stage IIA Hodgkin’s lymphoma at presentation (A) and after two cycles of ABVD (doxorubicin, bleomycin, vinblastine, dacarbazine) chemotherapy (B). The tumor seen in the mediastinum and bilateral neck shows intense avidity for the glucose analogue FDG prior to therapy consistent with increased glucose uptake in (A). Although a residual tumor mass is still seen on CT after two cycles of ABVD chemotherapy (B), it is no longer FDG-avid, and the patient has been in remission for over 2 years since completion of therapy. Normal FDG uptake and excretion is seen in the stomach, heart, and urinary tract, respectively. (Image courtesy of Tricia Locascio, Katherine Zukotynski, and Annick D. Van den Abbeele, Department of Imaging, Dana-Farber Cancer Institute.) |
Increased expression of HIF-1α-regulated genes is found in many different types of cancer and correlates with poor patient prognosis.48 Expression profiling of transformed cells demonstrates that metabolic genes are among the most strongly up-regulated groups of genes.49 Loss of expression of the HIF-1α target genes correlates with response to therapy in at least some models of cancer, suggesting that these genes are important for malignant cell proliferation and survival. Importantly, increased expression of glucose transporters and glycolytic enzymes under the control of HIF-1α are seen even in non-hypoxic tumors expressing VHL.49,50 HIF-1α also drives expression of pyruvate dehydrogenase kinase, a negative regulator of the pyruvate dehydrogenase complex that catalyzes pyruvate entry into mitochondria.51,52 Thus HIF-1α expression can promote aerobic glycolysis in cancer cells.
Transcription factors other than HIF-1α can promote the expression of metabolic enzymes. Expression of ChREBP-1, mondoA, and SREBP-1 has also been shown to be important in some cancers. ChREBP-1 is a key regulator of glycolytic enzyme expression and can promote anabolic
metabolism.53 MondoA is a key regulator of metabolic gene expression and coordinates glucose and glutamine metabolism.54 Enzymes involved in cholesterol and lipid metabolism are controlled by the SREBP transcription factor,55 and SREBP is induced as a result of oncogenic signaling.56 Finally, increased HIF-1α-mediated gene transcription is observed in many cancers in the absence of hypoxia or VHL loss.48 This has been attributed to increased production of HIF-1α that results from aberrant signaling downstream of phosphatidylinositol-3-kinase (PI3K), as discussed below.
metabolism.53 MondoA is a key regulator of metabolic gene expression and coordinates glucose and glutamine metabolism.54 Enzymes involved in cholesterol and lipid metabolism are controlled by the SREBP transcription factor,55 and SREBP is induced as a result of oncogenic signaling.56 Finally, increased HIF-1α-mediated gene transcription is observed in many cancers in the absence of hypoxia or VHL loss.48 This has been attributed to increased production of HIF-1α that results from aberrant signaling downstream of phosphatidylinositol-3-kinase (PI3K), as discussed below.
Many Genetic Drivers of Cancer Increase Nutrient Uptake
Highlighting the central role that altered cellular metabolism plays in tumor biology, one shared consequence of many genetic events that promote cancer is increased glucose uptake and metabolism. Transformation is associated with elevated glucose uptake.57
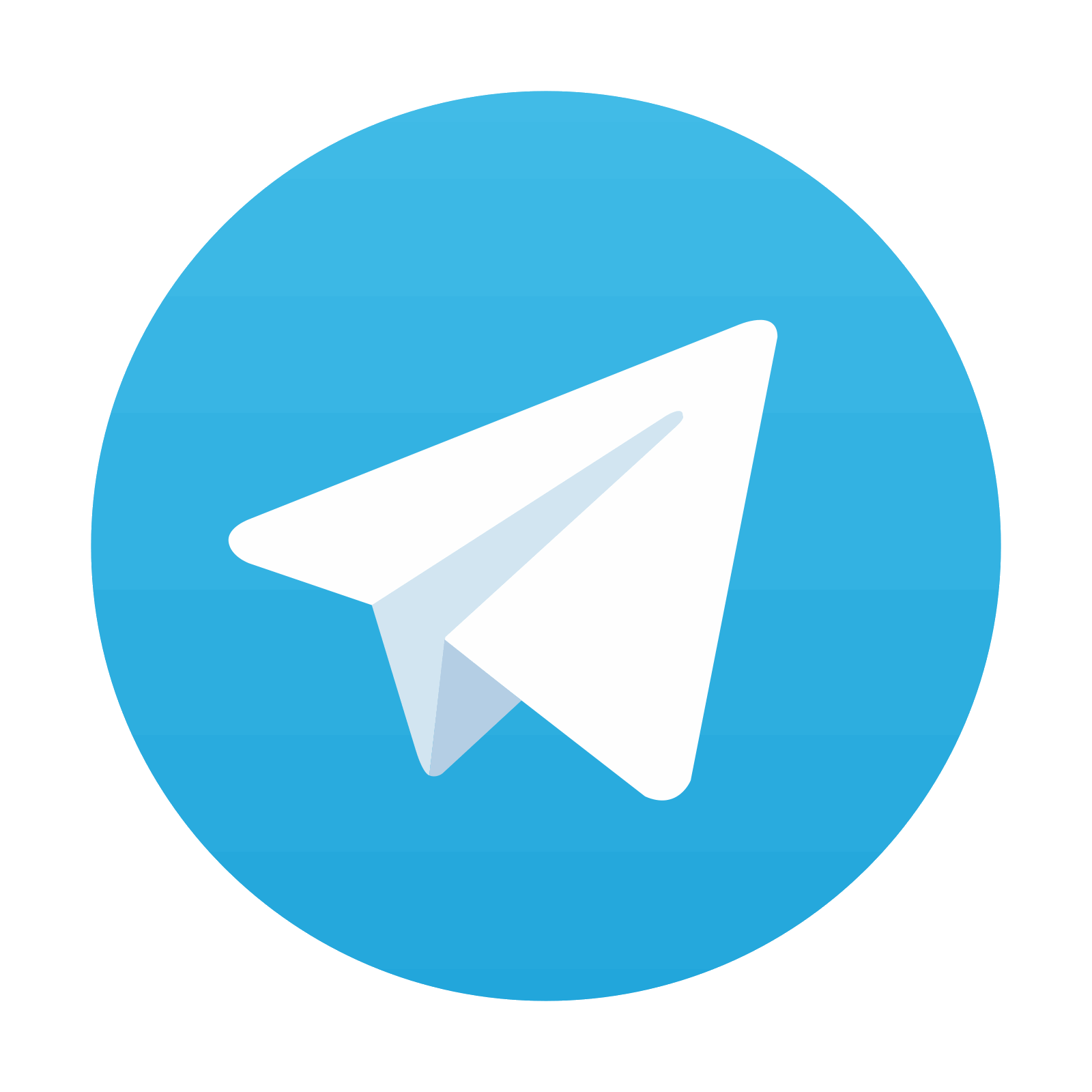
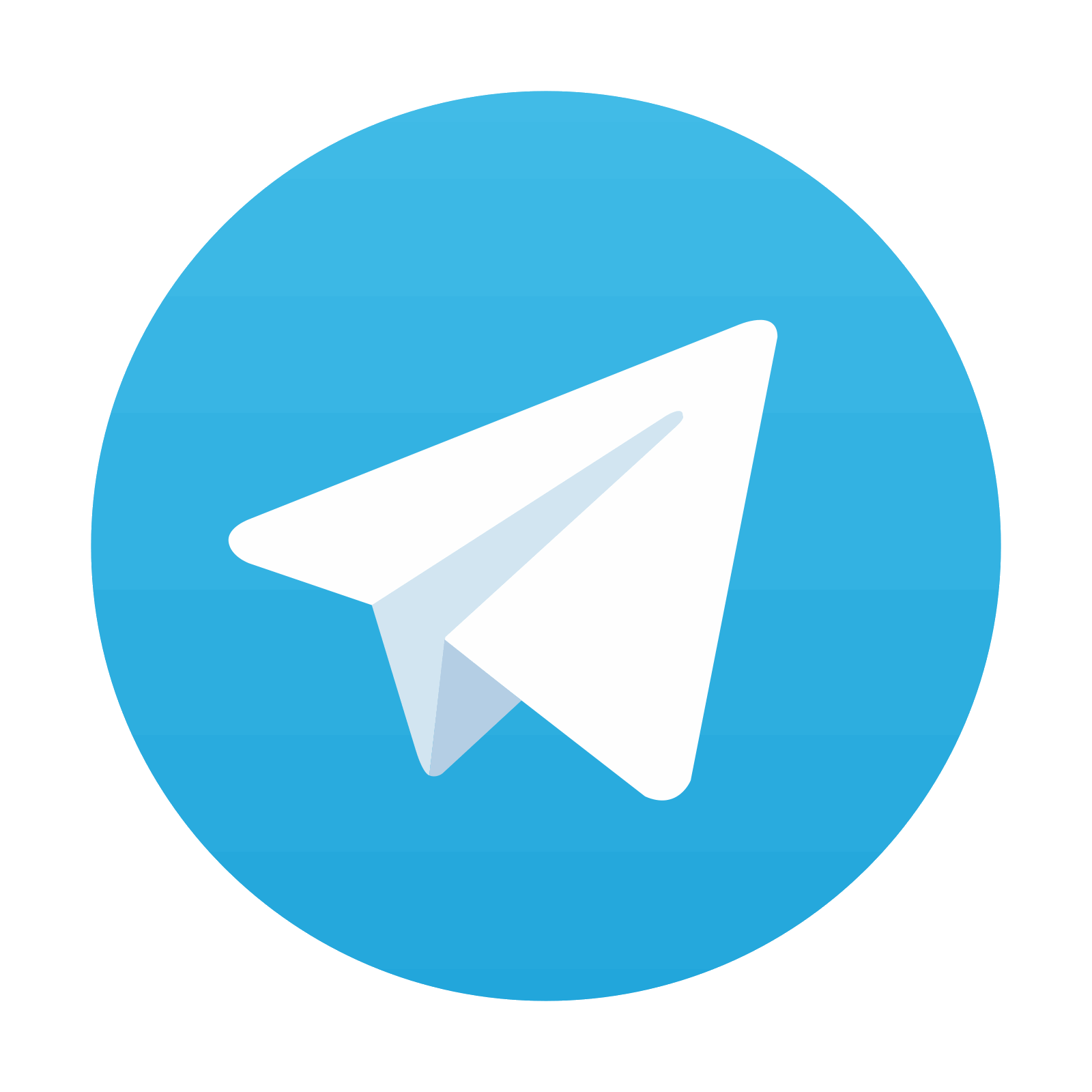
Stay updated, free articles. Join our Telegram channel
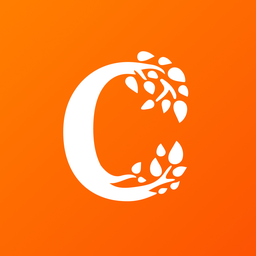
Full access? Get Clinical Tree
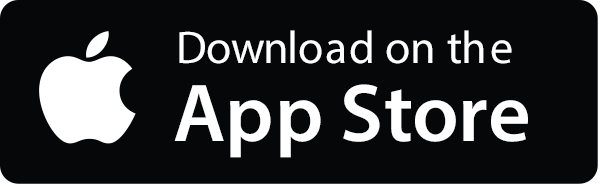
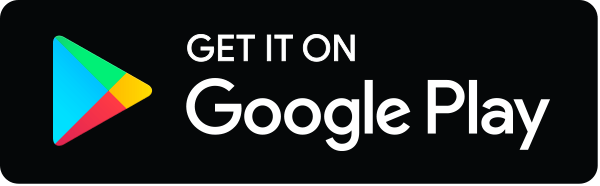
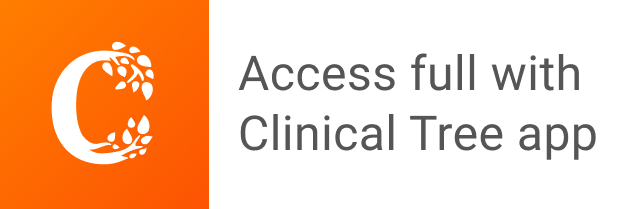