Blood Vessel Wall in Health and Disease
Keith R. Brunt
Sonya Hui
Jagdish Butany
Mansoor Husain
Large arteries and veins are comprised of concentric layers of smooth muscle cells (SMCs) and connective tissue, and a protective inner lining of endothelial cells (ECs). The latter normally functions as a pacifying inner lining that prevents thrombosis and secretes factors that regulate underlying SMC function and phenotype to modulate both vessel diameter and vessel wall structure. When cardiovascular risk factors harm or “activate” the endothelium and impair its normal function, pathogenic processes such as inflammation, thrombosis, SMC proliferation, and abnormal vessel contractility can be initiated. Sustained endothelial dysfunction of this nature is ultimately believed to underlie the earliest stages of atherosclerosis. Similarly, vascular dysfunction can be the incipient event of most acquired cardiovascular diseases. This chapter orients the reader to key anatomical and physiologic aspects of the vasculature, from the major conduit vessels to specific clinically relevant vascular beds, with a focus on their cellular composition and function.
BASIC VASCULAR ANATOMY AND HISTOLOGY
All vessels conform to certain common anatomical features. With the exception of capillary beds, the blood vessel wall is organized into three layers, or tunica: the intima, media, and adventitia (FIGURE 41.1).
The intima extends from the endothelium to the inner elastic lamina, which is a highly organized tensile matrix. The endothelium and its basement membrane is a dynamically regulated single-cell-thick semipermeable layer interposed between the blood and the rest of the vessel wall or, in the case of capillaries between the blood and tissue parenchyma. The endothelium is the principal site of production for many hemostatic and vasoactive substances such as nitric oxide and prostacyclin (see Chapter 42). Immediately abluminal to the endothelium is a thin layer containing fibroblasts and a fenestrated sheet of elastic fibers, termed the elastic lamina interna. This elastic layer membrane is surrounded on both sides by covalently bound loose connective tissue that provides scaffolding for endothelial adherens and integrins, which are proteins that anchor cells to the intimal matrix or to other cells in the intima. In larger vessels, the intima may also contain SMCs. While rarely found in the intima of healthy blood vessels, leukocytes from the blood such as lymphocytes, dendritic cells, or monocyte-macrophages can enter the blood vessel wall at sites of vascular injury or inflammation and contribute to pathologies such as atherosclerosis (see Chapter 89).
In larger blood vessels, the media is the thickest layer of the vessel wall and is comprised of several circumferential, spirally aligned SMC layers, each of which can be sandwiched between additional elastic laminae. The general function of the media is to maintain vascular tone (i.e., the state of contraction exerted by SMCs in response to stretch and/or neuroendocrine stimulation). The media of veins is thinner than that of arteries and contains more connective tissue, although major veins such as the inferior vena cava also possess thick medial walls with some longitudinally oriented SMC layers as well. The extracellular composition (e.g., collagen, elastin, and proteoglycan content) of the media varies depending on vessel size, compliance, and muscularity. The medial wall is innervated, and in large arteries with high metabolic requirements, the media is also supplied by small blood vessels known as vasa vasorum (see “Vasa Vasorum” below). In large- and medium-sized arteries, the border between the media and adventitia is marked by an external elastic lamina. This border can be difficult to discern as the external elastic lamina disappears in small arteries and is absent in arterioles.
The adventitia begins where the media ends and is composed of a complex mixture of mesenchymal and myeloid cells with loose extracellular matrix. In large arteries, the adventitia can be nearly as thick as the media, containing abundant collagen and even elastin fibers. The adventitia contains abundant fibroblasts, as well as SMCs, adipocytes, and leukocytes. It also houses the majority of the vasa vasorum (see “Vasa Vasorum” below). The general function of the adventitia is to provide metabolic and structural support for large vessels.
AORTA AND CONDUIT VESSELS
Anatomy of the Aorta
Adult vascular anatomy begins at the aortic root, as blood exits the left ventricle through the aortic valve. The aorta has four noteworthy anatomical segments: (a) ascending aorta (length ˜5 cm in humans), (b) aortic arch (˜4 cm), (c) descending aorta (1, 2, and 3 are collectively referred to as the thoracic aorta), and (d) abdominal aorta. The ascending aorta, just past the cusps of the aortic valve, is also the location of three sinuses: the left, right, and posterior sinuses. The left and right sinuses are the origins of the left and right coronary arteries, respectively. The three sinuses provide eddies that aid in the closure of the proximal cusps of the aortic valve during diastole. The superior curve of the aortic arch contains branches that form the brachiocephalic (becoming the left carotid and subclavian), right carotid, and left subclavian arteries before descending approximately 20 cm left of the midline. Below the diaphragm, the abdominal aorta extends another approximately 15 cm before it terminally branches into two common iliac arteries. As the aorta descends from thorax to abdomen, its diameter narrows from approximately 3.5 to 2.5 cm, as it gives rise to several intervertebral
arteries in the thorax, and major conduit arteries in the abdomen, namely the celiac, mesenteric, and renal arteries as well as smaller gonadal, lumbar and sacral branches.
arteries in the thorax, and major conduit arteries in the abdomen, namely the celiac, mesenteric, and renal arteries as well as smaller gonadal, lumbar and sacral branches.
Histology of the Aorta
The histology of the aorta is consistent with its function as a major conduit vessel. Its composition is predominately elastic > fibrous > muscular. This differs from resistance arteries, which are muscular > elastic > fibrous. The walls of the aorta and other large arteries contain large numbers of elastic fibers arranged in circumferential lamellar units.1,2 Each unit consists of two tightly packed elastic lamellae with cells and extracellular matrix contained within. A cross section of the thoracic aorta can reveal as many as 35 to 56 U, whereas the abdominal aorta typically has approximately 28 U.2,3
Physiology of the Aorta
To fulfill its functions as the central conductance vessel of the body, the aorta must have the elasticity and strength to stretch during systole, and to recoil during diastole, to correspondingly receive and transmit the entire left ventricular ejection volume and pulse pressure through to the smaller conduit arteries. The pressure pulse of blood created by systolic contraction of the ventricle is cushioned by the compliance of a highly elastic aorta. As the aortic valve closes during diastole, the potential energy stored in the aortic wall is converted into kinetic energy, propelling blood forward into the peripheral arteries with a smooth declination in flow velocity prior to the next systolic contraction.
Pathology of the Aorta
While the aortic endothelium is exposed to both high flow rate and shear stress, the curved geometry of the aorta also results in turbulent blood flow. In the lesser curvature of the aorta, turbulent flow can reduce shear stress, which predisposes the development of atherosclerosis.4,5,6,7 Additionally, during ventricular contraction the aorta bears both the circumferential wall tension (from transmural wall pressure) and tensile stress (transmural wall pressure divided by wall thickness), which is exerted perpendicular to the flow of blood. These forces also contribute to aortic remodeling and aneurysm formation that can accompany aortic inflammation and atherosclerosis. Any deficiency in the aortic wall that decreases elasticity will impair compliance and conduit function and can contribute to adverse effects such as aortic dilatation, aortic wall thickening, or even rupture. By contrast, aortic coarctation is a rare congenital anomaly that is associated with aortic constriction, typically at or near the ligamentum arteriosus, the adult remnant of the fetal ductus arteriosus (whose function was to allow oxygenated fetal blood from the placenta to bypass the nonfunctioning pulmonary circulation and enter the descending aorta of the fetus from the main pulmonary artery). Aortic compliance can also diminish with age. In this degenerative process, elastic fibers are lost, SMC apoptosis is observed, and extracellular matrix is altered, changing the architecture of the aortic wall. This pathology typically includes intimal thickening and calcification. Although less severe than the mechanical narrowing of aortic coarctation, age-associated aortic degeneration also creates an increased afterload on the heart, causing pressure overload hypertrophy with hypertension. Over time, age-related pathologic alterations to the composition of the aorta may include vessel wall breakdown and aortic ectasia, or dilatation of the aortic wall. Ectasia results in widening, elongation, and uncoiling of the aortic arch, which can so alter the geometry of the aortic root as to limit competency of the aortic valve leaflets and contribute to aortic regurgitation. Accordingly, both diminished or exaggerated aortic wall compliance may eventually affect cardiac function.
As mentioned previously, the aorta is susceptible to turbulent blood flow, endothelial dysfunction, and atherosclerosis (FIGURE 41.2).8,9 Atherosclerosis of the aorta can in turn lead to aortic aneurysm formation (FIGURE 41.3). Aneurysm, often secondary to atherosclerosis and rarely related to infection, can be: (a) dissecting (FIGURE 41.4), typically caused by cellular necrosis in the aortic media with subsequent tearing of a dissection plane between the lamellar units; (b) saccular, appearing as a bulging “sac” to one side; or (c) fusiform, a circumferential dilatation, in
the thoracic or abdominal aorta (see Chapter 72). Hypertension is a major contributing factor to aortic aneurysm.10 Certain vasculitides also predispose to aortic aneurysm including Takayasu arteritis, giant cell arteritis (FIGURE 41.5), and ankylosing spondylitis (see Chapter 100). Trauma, in particular midspeed motor vehicle accidents, can also result in aortic damage, particularly those in which passengers are exposed to sudden decelerations or concussive force trauma, including seat belt injury. Finally, genetic defects of specific extracellular matrix proteins, such as in Marfan disease, are uncommon but important causes of potentially catastrophic aortic pathology.
the thoracic or abdominal aorta (see Chapter 72). Hypertension is a major contributing factor to aortic aneurysm.10 Certain vasculitides also predispose to aortic aneurysm including Takayasu arteritis, giant cell arteritis (FIGURE 41.5), and ankylosing spondylitis (see Chapter 100). Trauma, in particular midspeed motor vehicle accidents, can also result in aortic damage, particularly those in which passengers are exposed to sudden decelerations or concussive force trauma, including seat belt injury. Finally, genetic defects of specific extracellular matrix proteins, such as in Marfan disease, are uncommon but important causes of potentially catastrophic aortic pathology.
VASA VASORUM
Anatomy of the Vasa Vasorum
While the inner third of the largest conduit vessels, such as the aorta, and inferior vena cava, is avascular, the outer two-thirds11 is vascularized by a branched microvascular bed called the vasa vasorum (latin “vessel of a vessel”; FIGURE 41.6). The “vasa” can emerge from the lumen, branch up, out and through the adventitial layer (arborize), and weave into smaller vasa throughout the adventitia (and to a lesser degree, the media). However, the
vasa can also be a component of more distal arterialization from smaller arteries such as the brachiocephalic artery to ascending aorta, coronary to pulmonary artery, or mesenteric artery to abdominal aorta/inferior vena cava.12,13 The evidence for both arborized and “nonself” arterialization of vasa arose initially from plastic cast dissections and electron microscopy (EM).14 However, more recent evidence is based on microanatomical computed tomography and microparticles that assessed both vasa anatomy and blood flow. Large arteries also contain lymph vessels, which can only be distinguished from the vasa through immunocharacterization. Most blood vessels with a cross-sectional thickness of more than 30 to 50 cells possess some form of mural vascularization, invariably more identifiable in adventitia than media. Generally, vasa are limited to the adventitia of vessels with media less than approximately 300 to 500 nm thickness or arteries with <28 lamellae.15 Diffusion distance is thus a key determinant of vasa. In the adventitia, vasa form capillaries, while in media, they may also form short-distance connections or unique arteriovenous shunts that empty into an adjacent vein.16 Importantly, vasa are end arteries, or arteries that supply the only perfusion to an anatomical site. As such, reduced flow or occlusion of vasa can result in hypoxia or ischemia of cells within the blood vessel wall.17
vasa can also be a component of more distal arterialization from smaller arteries such as the brachiocephalic artery to ascending aorta, coronary to pulmonary artery, or mesenteric artery to abdominal aorta/inferior vena cava.12,13 The evidence for both arborized and “nonself” arterialization of vasa arose initially from plastic cast dissections and electron microscopy (EM).14 However, more recent evidence is based on microanatomical computed tomography and microparticles that assessed both vasa anatomy and blood flow. Large arteries also contain lymph vessels, which can only be distinguished from the vasa through immunocharacterization. Most blood vessels with a cross-sectional thickness of more than 30 to 50 cells possess some form of mural vascularization, invariably more identifiable in adventitia than media. Generally, vasa are limited to the adventitia of vessels with media less than approximately 300 to 500 nm thickness or arteries with <28 lamellae.15 Diffusion distance is thus a key determinant of vasa. In the adventitia, vasa form capillaries, while in media, they may also form short-distance connections or unique arteriovenous shunts that empty into an adjacent vein.16 Importantly, vasa are end arteries, or arteries that supply the only perfusion to an anatomical site. As such, reduced flow or occlusion of vasa can result in hypoxia or ischemia of cells within the blood vessel wall.17
Physiology of the Vasa Vasorum
Vasa are required to meet the metabolic needs of thicker blood vessels. As such, vasa are governed by the same metabolic cues that regulate angiogenesis in other beds,17 with vessel wall hypoxia representing a trigger for new vasa formation, increased vasa density,18,19 and even increases in blood flow.13 Consistent with this, large energy-consuming arteries are more vascularized in the media than compliant vessels of similar size such as veins.13 Commensurate with lower vasa density, blood flow through the vasa of veins tends to be lower than in the vasa of arteries.3,20 However, the more muscular the vein, the greater its metabolic demand and the greater its vasa density and blood flow.13,21 Compression forces in larger arteries also influence flow, with perfusion through the vasa of carotid arteries occurring mostly after pulse velocity flow.22 Finally, these vessels are also innervated and also respond to neuroendocrine factors that regulate tone.
Pathology of the Vasa Vasorum
While vasa vasorum appear to play a role in vascular disease, the extent to which their dysfunction is causal or correlative remains unknown. Restricted or occluded flow through the vasa can result in hypoxia or necrosis of the vessel wall.23 Experiments in dogs have demonstrated that removal or occlusion of vasa caused medial necrosis, reduced vessel compliance,23 and neointimal formation akin to restenosis.24 Obstructions of the vasa can also occur as a result of thrombosis, hypertension, or interventions, such as bypass graft surgery or angioplasty, in which vasa may be cut or compressed. Since vasa possess arterial tone, regulated by autocrine and neuroendocrine responses, they are susceptible to constriction from pressor molecules (e.g., angiotensin II or endothelin I) that are typically elevated in hypertension.16,25 Also, similar to the coronary circulation, vasa blood flow is reduced by compression forces from elevated systemic blood pressure during systolic contraction.26 Chronic hypertension is associated with impaired vasodilator capacity27 and can reduce vasa flow, which may partially underlie aortic remodeling in models of chronic hypertension.
Atherosclerotic lesions, particularly in humans, display significant vasa remodeling, with increasingly disorganized microvessel density in proportion to the extent of the lesion. This has broadly led to the hypothesis that vasa play a pathogenic role in atherosclerosis.28,29 Specifically, thrombotic occlusion of vasa may create acute necrotic events that trigger monocyte invasion and further lipid infiltration.30,31 Of considerable interest, the vasa of atherosclerotic lesions are anatomically distinct from normal vasa; their architecture resembles that of tumor vessels with increased tortuosity and instability.32 As such, the
term vasa plaquorum has been proposed for vasa supplying atherosclerotic lesions. Vascular lesions in vasa-perfused vessels are not only a source of potent thrombotic and inflammatory stimuli, they also increase arterial wall thickness, and thus reduce oxygen tension in the plaque. Indeed, vasa plaquorum angiogenesis may be initiated by mitogenic stimulation and/or hypoxic demand. However, upon encountering the hostile microenvironment of the plaque itself, vasa plaquorum fail to develop normally; they can be leaky, with fragile endothelium lacking the pericytes (see below) necessary for development of proper tone.33 Therefore, vasa plaquorum can be a source of intraplaque accumulation of albumin, fibrinogen, hemorrhage, and inflammatory cells. Counteracting these negatives, the vasa plaquorum may also support nutrient flow to the lesion, thereby preventing necrosis.29 Depending on lesion composition, the vasa may also provide the circulatory basis for plaque regression and vessel remodeling.34 As such, the role of vasa plaquorum is far from definite.
term vasa plaquorum has been proposed for vasa supplying atherosclerotic lesions. Vascular lesions in vasa-perfused vessels are not only a source of potent thrombotic and inflammatory stimuli, they also increase arterial wall thickness, and thus reduce oxygen tension in the plaque. Indeed, vasa plaquorum angiogenesis may be initiated by mitogenic stimulation and/or hypoxic demand. However, upon encountering the hostile microenvironment of the plaque itself, vasa plaquorum fail to develop normally; they can be leaky, with fragile endothelium lacking the pericytes (see below) necessary for development of proper tone.33 Therefore, vasa plaquorum can be a source of intraplaque accumulation of albumin, fibrinogen, hemorrhage, and inflammatory cells. Counteracting these negatives, the vasa plaquorum may also support nutrient flow to the lesion, thereby preventing necrosis.29 Depending on lesion composition, the vasa may also provide the circulatory basis for plaque regression and vessel remodeling.34 As such, the role of vasa plaquorum is far from definite.
CORONARY ARTERIES
Anatomy of the Coronary Arteries
The coronary arteries emerge from the left and right aortic sinuses to provide the entire blood supply of the myocardium. The left main coronary artery branches to form the circumflex (Cx) and left anterior descending artery (LAD). The LAD traverses the left ventricle in the anterior interventricular groove, giving off septal perforators and typically two distal diagonal branches as it makes its way toward the apex. The Cx, which lies in the atrial-ventricular (AV) groove, also gives off two descending marginal branches. The right coronary artery (RCA) traverses the descending circumferential aspect of the right ventricle (RV). It branches toward the apex of the RV as the marginal artery, before continuing on to form a dedicated arterialization of the AV node, with the remaining vessel forming the posterior descending artery (PDA) that runs along the posterior interventricular groove toward the left ventricular apex. In approximately 15% of humans, the PDA is formed by a continuation of the Cx rather than the RCA, in which case the coronary circulation is termed “left dominant” (as opposed to the more common “right dominant”). All of the above epicardial arteries give off smaller penetrating branches that perfuse the myocardium from epicardium to endocardium.
The coronary circulation has many small connecting arterial processes termed collaterals and anastomoses that allow blood to flow between the main epicardial vessels, providing alternative routes of blood flow. The prevalence of collaterals and anastomoses in the coronary circulation undoubtedly reflects the importance of the heart as a vital organ, and the critical importance of redundant perfusion routes, particularly in the event of a coronary vessel occlusion. Having said this, the papillary muscles that tether the AV valves are often vulnerable to ischemic damage and dysfunction with the occlusion of specific coronary territories. The anterior papillary muscle of the left ventricle is perfused by the LAD, whereas the posterior papillary muscle is perfused by the PDA.35 The atria are vascularized by branches of the AV and sinoatrial (SA) nodal arteries; the SA nodal artery arising most commonly from the RCA.36,37,38
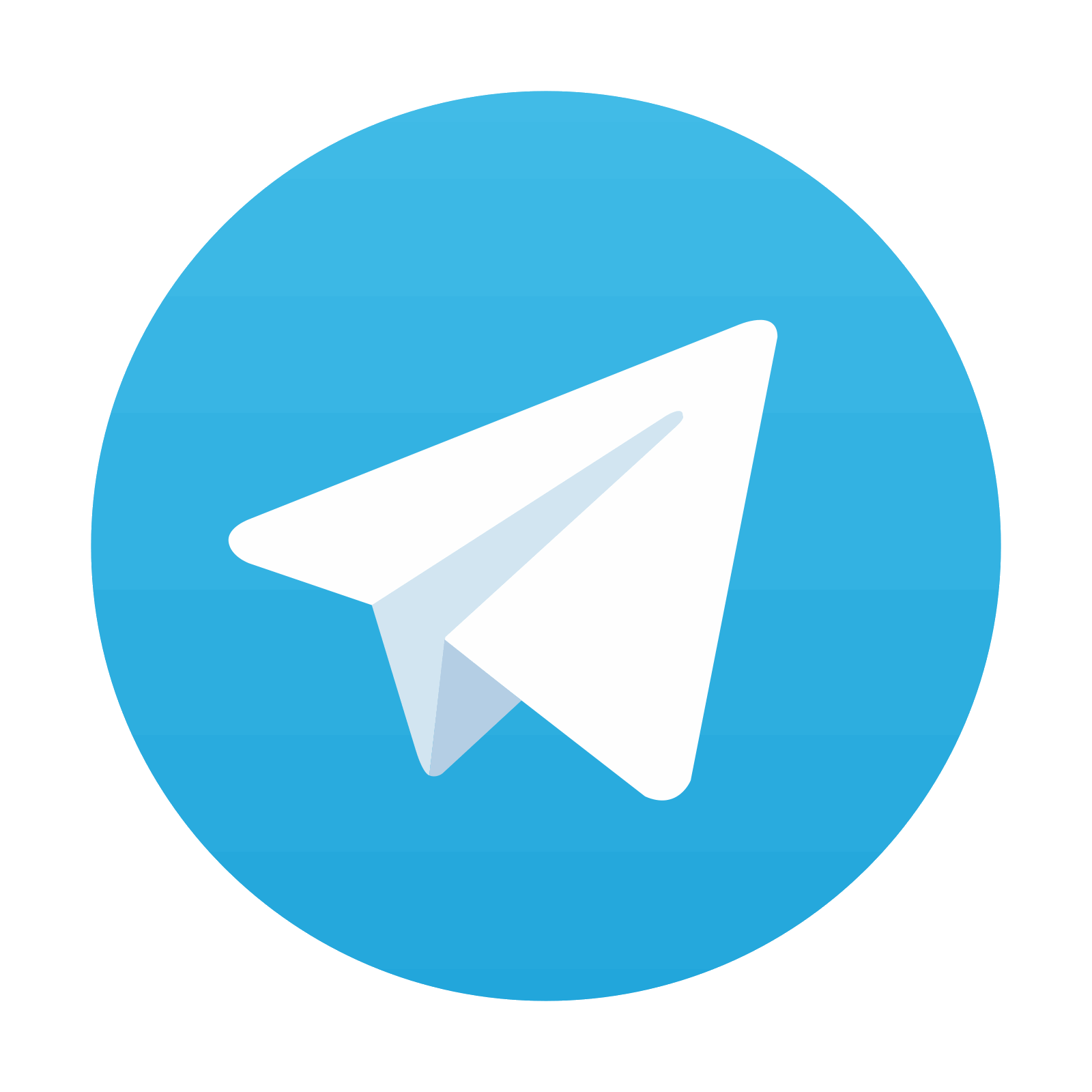
Stay updated, free articles. Join our Telegram channel
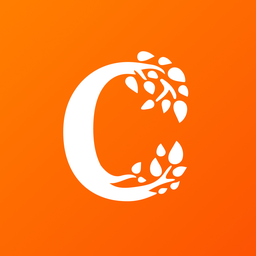
Full access? Get Clinical Tree
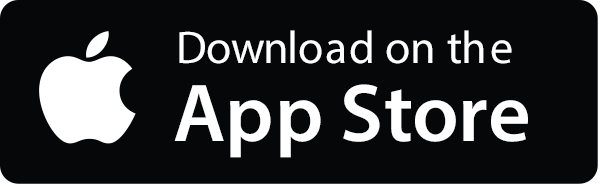
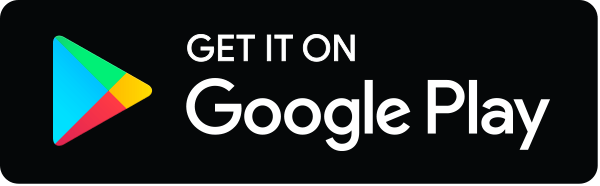