Basic Breast Ultrasound, Certification, and Accreditation for the Surgeon: Introduction
Breast ultrasound has become an indispensable tool for surgeons who wish to provide state-of-the-art care to patients with diseases of the breast. Surgeons depend daily on their interpretation of the images to make diagnoses and to guide interventional procedures. To understand the ultrasound images properly, the surgeon must have a thorough understanding of the physics involved in the generation of those images.
Sound is a form of mechanical energy that travels at varying speeds through different materials by causing temporary compression and rarefaction of the molecules of those materials. Consequently, unlike electromagnetic radiation, sound cannot travel through a vacuum. Sound travels in waves, and there is an inverse relationship between the wavelength (the distance from one point on the wave to the same point on the next wave) and the frequency of the waves. A human with perfect hearing might hear frequencies between 20 and 20,000 cycles per second or 20 Hz to 20 kHz. Sound with a higher frequency than audible sound is referred to as ultrasound. Diagnostic medical ultrasound is generally somewhere between 2 and 30 million cycles per second, or 2 to 30 MHz.
The fundamental operating principle of all ultrasound transducers is the piezoelectric effect, discovered by Pierre Curie and his less famous brother, Jacques. There are crystals that occur in nature and others that can be created that have the ability to transform electrical energy into sound and vice versa. A linear array transducer used in breast ultrasound has a series of tiny piezoelectric crystals extending from one end of the footplate to the other, each of which can be fired individually or in groups. When a voltage is applied to the piezoelectric crystal, the crystal is deformed and the electrical energy is converted into mechanical energy in the form of sound. A damping material adjacent to the crystal acts like a finger on a tuning fork to immediately stop the sound, resulting in a very short pulse. Operating according to the pulse-echo principle, the transducer is generating ultrasound less than 1% of the time. Greater than 99% of the time it is “listening” to returning echoes, which are in turn converted back into electrical energy and recorded.
When sound meets an interface between tissues with different acoustic impedances (an acoustic mismatch), part of the sound is transmitted into the second tissue, and part is reflected as an echo. The impedance of the tissue is simply the product of the density of the tissue and the rate at which sound travels in it. The greater the acoustic mismatch between adjacent tissues, the greater the echoes produced at that interface. The returning echoes are recorded on a scale in proportion to their strength or amplitude with a varying degree of brightness. Ultrasound using this scale is called B-mode scanning (the B stands for brightness). The highest strength echoes are displayed as white, the absence of echoes as black, with different shades of gray in between. Thus the other name for B-mode scanning is gray scale. The degree of echogenicity is compared with the surrounding tissues, usually the breast parenchyma, although some authors use the subcutaneous fat as the reference area.1 Tissues that generate the same degree of echogenicity as the reference area are referred to as isoechoic. Those that generate more echoes are hyperechoic, and fewer echoes are hypoechoic. The absence of echoes is referred to as anechoic. Because sound travels through most soft tissues at a rate of around 1450 to 1550 m/s, only low-level echoes are produced at most of the interfaces where there are only slight differences in acoustic impedance. Where dense tissue is adjacent to much less dense areas, there is a greater acoustic mismatch and more echoes are generated. Air is a poor conductor of sound with a speed in the range of 330 m/s, whereas bone conducts sound at speeds in excess of 4000 m/s. When sound meets an interface between soft tissue and either bone or air, there is a large acoustic mismatch, and high-level echoes are seen.
Each crystal in the transducer fires several thousand times a second and interrogates the breast tissue underneath it as the ultrasound beam travels through the tissues. Multiple echoes return from each pulse of sound as it travels deeper and deeper. After each 100 or so crystal firings, all of the echoes from all of the tissues are combined into an image, or “frame,” and displayed on the screen. With a frame rate of about 30 per second, the human eye cannot detect the individual images, and it appears that the scanning is being done in real time, like a motion picture. If the frame rate is significantly lower and the transducer is moved fairly rapidly across the patient’s breast, the individual images can be perceived, and a flickering pattern appears on the screen, much like an old-time silent movie.
Part of the sound that strikes a smooth acoustic interface perpendicular or nearly perpendicular to the path of the ultrasound beam (at right angles to the footplate of the transducer) is reflected back to the transducer and recorded. The pixels are displayed on the screen at a depth commensurate with the amount of time that has elapsed from the firing of the crystal until the return of the echoes. The longer the interval of time, the deeper (lower) the pixel is displayed on the screen, the so-called time-space conversion.
As the sound goes deeper into the tissues, it gradually weakens or attenuates for 4 different reasons.2 The most significant of these is that the tissues absorb the sound and the mechanical energy is converted into heat, albeit a negligible amount in diagnostic ultrasound.3 The second factor is that some of the energy is reflected back to the transducer as echoes. Third, when the sound strikes an irregular surface it is scattered through the tissues without returning to the transducer, further attenuating it. The final thing that attenuates the sound is the refraction (or bending) of the sound at the side of smooth surfaces such as cysts or fibroadenomas.
Overall gain refers to the amplification of the returning echoes, making the entire screen brighter. If the echoes throughout the screen were amplified to the same degree, the near field (top of image) would be very bright and the far field very dark as the sound attenuates in the deeper tissues. Built into the ultrasound equipment is a function known as time-gain compensation (TGC). The longer the time elapsed from the time the crystal fires until the echo returns to the transducer, the greater the amplification of the echoes. There are also individual slide pods that allow the sonographer to further fine-tune the amplification at each level, adjusting the image so that relative echogenicity is the same throughout the image. When TGC is set appropriately, fat in the near field has the same echogenicity as fat in the far field.
Resolution of the image refers to the ability of the equipment to distinguish 2 separate reflectors in the tissues and display them as 2 separate pixels on the screen. If the reflectors are side by side, parallel to the footplate of the transducer and perpendicular to the ultrasound beam, the resolution is referred to as lateral. If they are at different depths on the y-axis of the screen, parallel to the beam and perpendicular to the footplate, the resolution is axial. The higher the frequency of the ultrasound beam, the greater the resolution, both axial and lateral. A short pulse length is also an important contributor to good axial resolution. If one imagines 2 reflectors in an axial (vertical) plane with a short pulse of high-frequency ultrasound passing through them from the transducer, it is easy to imagine that pulse fitting between them and distinguishing them as separate. However, if either the pulse length increases or the frequency decreases (lengthening the wave length), the pulse will overlap the reflectors and not be able to distinguish them as separate. The reflectors will be merged and displayed as one reflector on the image, decreasing axial resolution (6411128).
Figure 31A-1
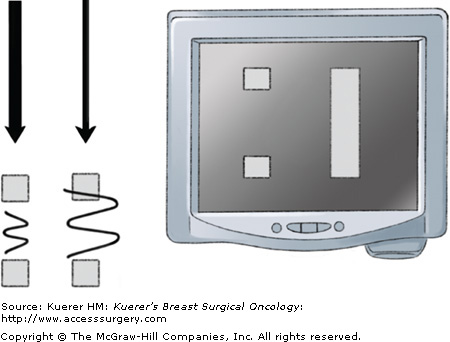
If the wavelength and pulse width are short enough to fit between 2 axial reflectors, they will be distinguished and displayed as separate pixels on the image, increasing axial resolution. If the wavelength or pulse width is long enough to overlap the reflectors, they will be merged and displayed as 1, decreasing axial resolution.
The ultrasound beam can be electronically focused (narrowed) by varying the sequencing of the groups of crystals that are fired together. Most instruments have a mechanism that allows the sonographer to move the depth of the focal zones to the area of interest or to add additional focal zones. If 2 lateral reflectors are close enough together that they can both fit within the width of the unfocused beam, they will be merged and displayed as 1. However, if the focal zone is placed at the level of the reflectors, narrowing the beam such that the 2 can no longer fit within it, they will be recognized and displayed as separate, increasing lateral resolution (6411132).
Figure 31A-2
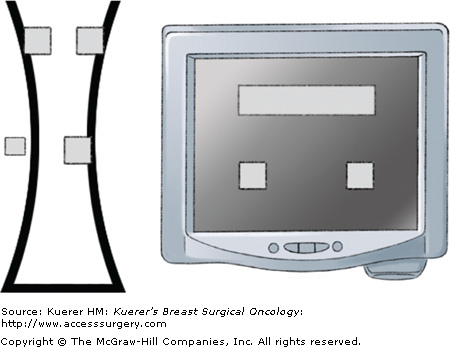
If 2 lateral reflectors are close enough together that they can both fit within the width of the unfocused beam, they will be merged and displayed as 1. However, if the focal zone is placed at the level of the reflectors, narrowing the beam such that the 2 can no longer fit within it, they will be recognized and displayed as separate, increasing lateral resolution.
As mentioned previously, a high frame rate allows the sonographer to see an image in real time as though it were a motion picture. When there is 1 focal zone, the beam is focused at that depth each time the crystals fire, allowing the highest frame rate. If another focal zone is added, the beam is focused alternately at each of the 2 levels when the crystals are fired. The resolution is the same at both levels, but it takes twice as long to acquire the same amount of information. Thus the number of frames that can be acquired in a given period of time is cut in half. As additional focal zones are added, the frame rate continues to decrease proportionally, such that the human eye can better perceive the individual frames, yielding a flickering pattern as the transducer is passed across the breast. It is a matter of individual preference as to whether the sonographer prefers to accept the flickering pattern with numerous focal zones or move an individual focal zone to the depth of the lesion as different areas of the breast are examined.
The reason one cannot always use a high-frequency transducer is that the penetration of the beam decreases as frequency increases. Thus to examine structures deep in the abdomen one might have to use a 3-MHz transducer, whereas examination of most breasts can be done quite well with a 10- to 14-MHz transducer because there is less tissue to penetrate. Think of a thunderstorm. If lightning strikes nearby, there is a loud, high-pitched (high-frequency) clap of thunder. However, if the storm is 10 miles away, the high-frequency thunder has attenuated, and one hears only the low-frequency rumble that has penetrated much further. The same principle is true if one listens to a band playing down the street or a stereo from down the hall. The sound from the high-pitched flute has attenuated and is not heard. Only the low-frequency bass drum and other low-pitched instruments are audible.
When the ultrasound beam encounters a strongly attenuating structure, there will be a relative decrease in the amount of sound that continues to pass into the deeper structures. There will be fewer echoes returning from the depths of the image below the structure, and the image there will appear more hypoechoic or even anechoic. This is referred to as posterior shadowing. An extreme example would be the very high acoustic mismatch between soft tissue and rib. Almost all of the sound is reflected from the surface of the rib and appears on the screen as a bright hyperechoic rim at the anterior surface of the rib. The remainder of the rib and the deeper tissues below it are not well seen because there is essentially no sound there, resulting in an almost anechoic shadow (6411141).
Figure 31A-3
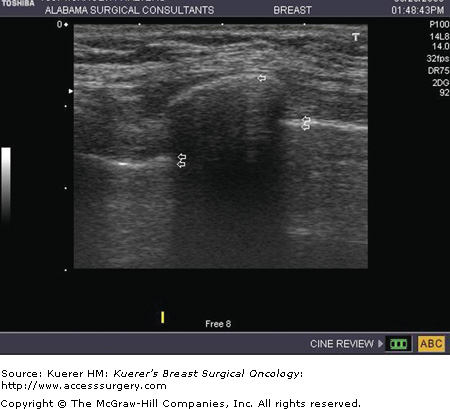
The high acoustic mismatch between the soft tissue and rib results in near-total reflection of sound, seen as the hyperechoic rim at the superficial surface of the rib (single arrow). The posterior shadowing obscures the remainder of the rib and underlying structures including the pleura (double arrows).
When the ultrasound beam encounters a weakly attenuating structure, there will be a relative increase in the amount of sound (relative to the adjacent tissue) that continues to pass into the deeper structures. There will be more echoes returning to the transducer, and this will result in a brighter (more hyperechoic) area deep to the structure, sometimes referred to as “good through transmission.” Cysts with clear fluid are classic examples of weakly attenuating structures because there are no acoustic mismatches when the sound passes through the fluid. Other relatively homogeneous structures can also be weakly attenuating and cause posterior enhancement, including medullary, colloid (mucinous), or high-grade carcinomas.1 The tissues immediately below all of these structures appear hyperechoic relative to the adjacent tissue because there has been more attenuation of the sound in the adjacent tissues by the time it reaches that depth. In addition, the area immediately beneath these weakly attenuating structures appears brighter than the tissue immediately superficial to the structure, even though the amount of sound (and echoes) are essentially the same. This phenomenon is caused by the fact that the deeper tissues are amplified more due to time-gain compensation (6411145).
Figure 31A-4
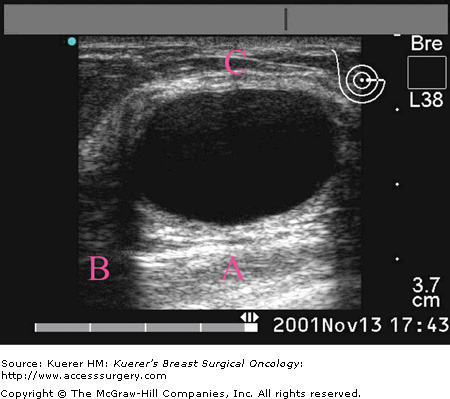
Posterior enhancement is seen deep to the cyst. The area marked B is darker than A because more of the sound has been attenuated as it passed through the superficial tissues. The area marked A is brighter than the area marked C because there is more amplification of those echoes from time-gain compensation (TGC). Note the reverberation echoes in the superficial aspect of the cyst being caused by the thickened anterior cyst wall.
Reverberation echoes are often mistakenly thought of as being generated by a cyst. In fact, the cyst has nothing to do with their creation. Reverberation echoes are occurring all of the time throughout the ultrasound image. They are lost in the myriad of other echoes, and we just don’t see them until an otherwise anechoic window is opened on the screen, such as a large cyst. To understand reverberation, look at the needle in the tissue parallel to the footplate of the transducer at a depth of 0.6 cm (6411149). The strong acoustic mismatch creates a very hyperechoic structure on the screen at 0.6 cm, placed at that level by the amount of time that elapsed before the echoes returned to the transducer. The transducer itself, however, represents a very strong acoustic mismatch with the skin, so part of the returning beam is reflected back into the tissue of the breast. When the beam that is reflected back into the tissue strikes the needle again, the echoes return a second time to the transducer, part of which are recorded and part of which make another trip into the tissue, and so on. The part that is recorded took twice as long to return as the original echoes, so the computer displays them at twice the depth, or 1.2 cm, according to the time-space conversion principle. Look closely and you will see a somewhat weaker replica of the needle at that depth. Between and below those 2 “needles” are numerous other weaker copies of the needle, regularly spaced very close to each other. These reverberations are being caused by the fact that the anterior and posterior walls of the needle also serve as reflectors, and sound is continuously bouncing back and forth between them. With each bounce, some of the sound travels back to the transducer and arrives slightly later than the previous echo and is recorded slightly deeper on the screen. The evenly spaced reverberation echoes that are seen in the superficial aspect of a cyst are being caused by some reflectors just superficial to the cyst. If one looks closely, an area of fibrous tissue, or perhaps a thick cyst wall, can usually be identified as the cause of the echoes (6411145). Whereas it is true that sound bounces between the anterior and posterior walls of the cyst, those echoes are displayed deep to the cyst and are generally lost in the tissue and not seen.
Figure 31A-5
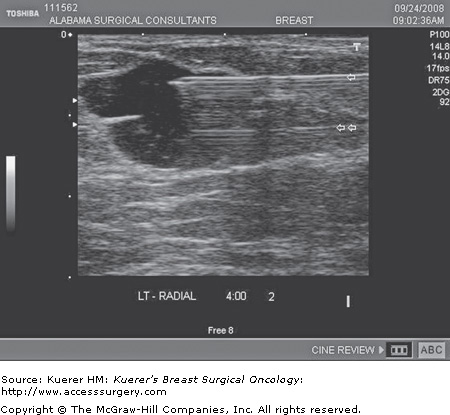
The needle in this image is adjacent to the cancer at a depth of 1 cm. The first reverberation caused by reflection from the transducer can be seen at a depth of 1.2 cm. The multiple closely spaced weaker reverberations are caused by the small delay in the echoes that return to the transducer each time the sound bounces between the acoustic mismatches at the anterior and posterior walls of the needle.
According to Snell’s law, when sound (or light) crosses an interface between 2 media in which the speed of transmission is different, the change in velocity will cause the transmitted beam to travel at a different angle relative to the interface, thus causing the wave to bend. This phenomenon is called refraction and explains why a pole placed into a swimming pool will appear to be bent. When ultrasound passes into a relatively round structure such as a cyst or fibroadenoma, the closer the beam gets to the edge of the structure, the greater the angle of incidence. If the rounded structure has different acoustic properties from the surrounding tissues, the sound wave will be bent once as it enters the structure and again as it exits. This bending of the sound wave deflects sound away from the edge of the structure, resulting in the relative absence of sound deep to the edges of the structure. This usually causes bilateral, fairly symmetric shadows called edge shadowing or edge effect.
Improvements in ultrasound technology and image interpretation over the last decade or so have made ultrasound an invaluable tool in the evaluation and characterization of various breast problems. Indications for breast ultrasound include palpable masses, mammographic masses and densities, abnormalities detected on magnetic resonance imaging (MRI), problems with implants, nipple discharge, focal pain, and the evaluation of response of cancers to preoperative therapy. Ultrasound is also an important tool in the evaluation of the extent of disease and in excluding multicentricity and bilaterality in patients with known breast cancer.
In past decades traditional surgical teaching was that most palpable masses that could not be resolved with aspiration should be biopsied. Ultrasound has fundamentally changed that recommendation, significantly decreasing the incidence of biopsy, but it is critical for the physician to palpate the abnormality at the time of scanning to be certain the findings correlate with what is being felt. For large masses this might mean placing a finger alongside the transducer and rolling it over the mass as the transducer is painted across the lesion. Smaller masses can be trapped between the long and index fingers and the transducer placed between them. Alternatively, an opened gem clip or small straw can be placed beneath the transducer overlying the palpable mass. The reverberation artifact from the marker allows the sonographer to be sure the palpable mass is being interrogated.
Often the palpable mass can be dismissed as completely benign without further intervention. If the mass is shown to be a simple cyst there is no need to aspirate it unless the cyst is symptomatic and aspiration might alleviate pain. Frequently what the patient and referring physician feel is a ridge of normal fibroglandular tissue that comes close to the skin (6411158). The patient may have been previously told that a mammogram and ultrasound show nothing, leaving her anxious about the mass that she continues to feel. It is important for the physician to show the images to the patient, reassuring her that what is seen on the ultrasound correlates with what she is feeling and is completely normal tissue.
Figure 31A-6
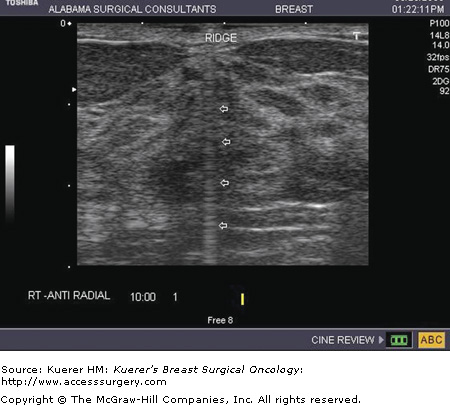
Note the ridge of normal breast tissue that comes closer to the skin than the surrounding tissue. It is firmer than the adjacent fatty tissue and gives the sensation of a mass. An opened gem clip over the mass produces reverberation echoes (arrows) that overlie the ridge, leaving no doubt that the palpable mass and the sonographic ridge are the same.
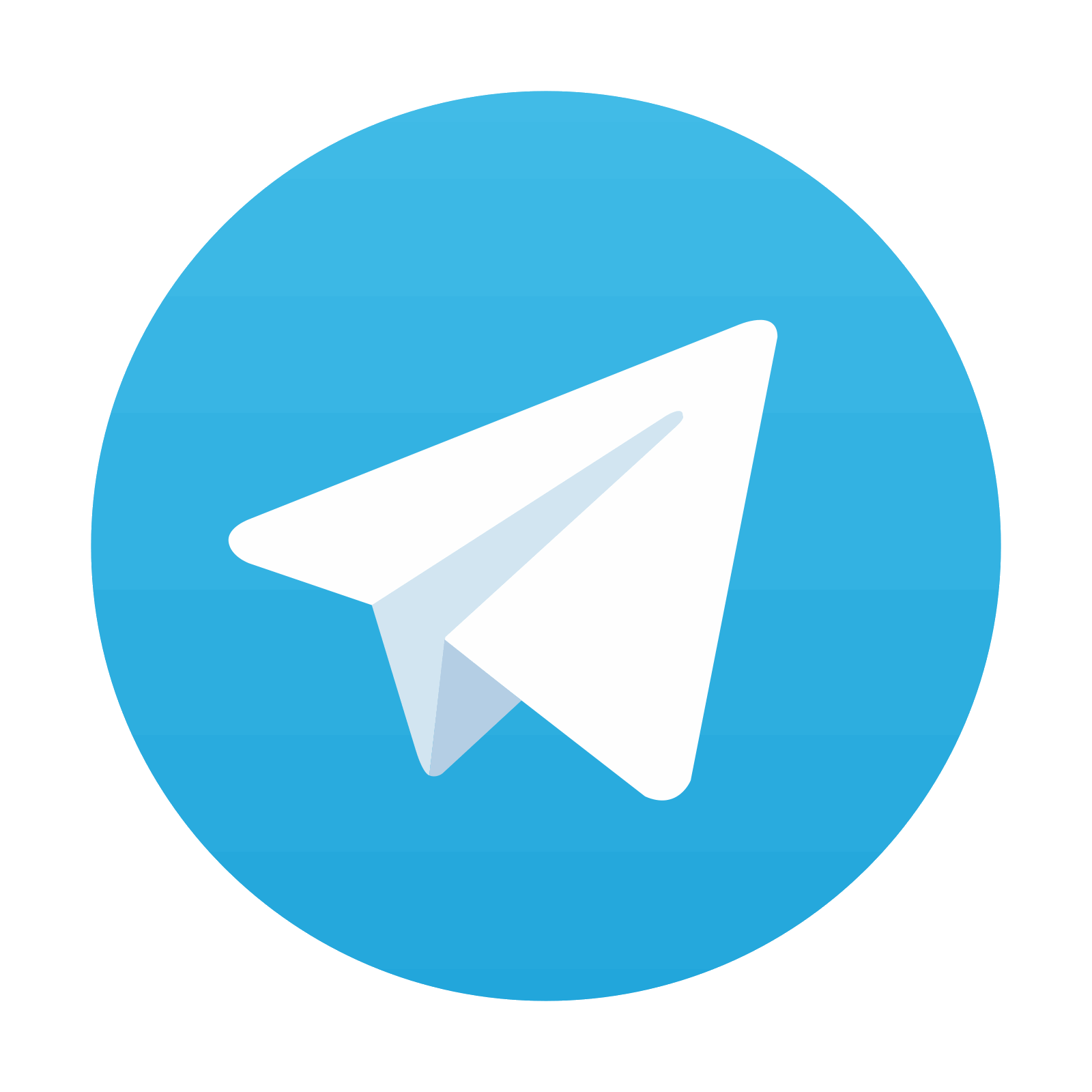
Stay updated, free articles. Join our Telegram channel
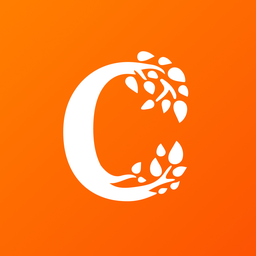
Full access? Get Clinical Tree
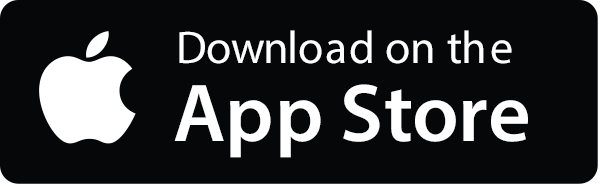
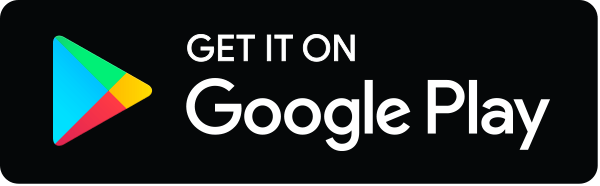
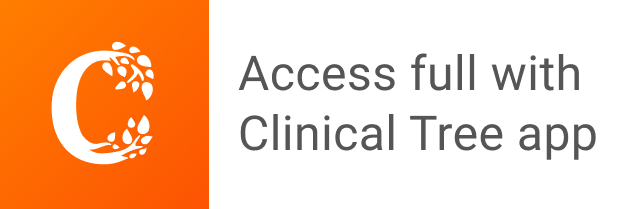