The Maturation of Thyroid Function in the Perinatal Period and During Childhood
Delbert A. Fisher
Rosalind S. Brown
Introduction
The maturation of thyroid function in the human fetus is a complex process that involves the growth and development not only of the thyroid gland itself but also of the intricate network of regulatory systems required for mature thyroid function as well. These include the development of the hypothalamus and pituitary glands, and, in addition, the coordinated maturation of mechanisms required for thyroid hormone transport, metabolism, and action. During fetal life circulating levels of thyroxine (T4), and its active metabolite triiodothyronine (T3), are low whereas the inactive metabolites reverse T3 (rT3) and T3 sulfate are high. This pattern of metabolites is a consequence of both immaturity of the hypothalamic–pituitary–thyroid axis and coordinated adjustments in the deiodinase system that determine whether T4 will be activated or inactivated. Despite the low serum T3 concentration, the level of T3 in vital organs such as brain is preserved, reflecting the importance of local conversion of T4 to T3. Maternal thyroid hormone, regulated by placental transport and metabolism, contributes to the early fetal thyroid hormone supply, particularly in the first half of gestation. The physiologic rationale for the maintenance of reduced circulating T3 levels throughout fetal life is still unknown, but it has been suggested that its function may be to avoid tissue thermogenesis and potentiate the anabolic state of the rapidly growing fetus while at the same time permitting highly regulated, tissue-specific maturation in an orderly temporal sequence.
Postnatally, changes in thyroid hormone secretion and metabolism occur that permit the neonate to adapt to the changing demands of extrauterine life. Unique to the childhood years, thyroid hormone plays a critical role in the maturation of numerous target organs, including bone, brown adipose tissue (BAT), cochlea, retina, liver, lungs, and heart as well as brain. These maturational programs are precisely controlled and occur in developmentally regulated, tissue-specific windows of time. In this chapter, the intricate regulation of the development of the thyroid system will be reviewed and recent important insights into our understanding discussed.
Thyroid system ontogenesis can be divided into four phases: Embryogenesis, maturation of the hypothalamic–pituitary–thyroid system, maturation of thyroid hormone metabolism, and maturation of thyroid hormone transport and action. Postnatal thyroid function in infancy, childhood, and adolescence will also be described.
Embryogenesis
Thyroid
Embryogenesis of the thyroid, pituitary, and hypothalamus is reviewed in detail in Chapters 2 and 3. In brief, the thyroid gland is derived from the fusion of a medial outpouching from the floor of the primitive pharynx, the precursor of the T4-producing follicular cells, and bilateral evaginations of the fourth pharyngeal pouch, which give rise to the parafollicular, or calcitonin (C) secreting cells. Commitment toward a thyroid-specific phenotype as well as the growth and descent of the thyroid anlage into the neck results from the coordinate action of a complicated, sequential cascade of transcription factors and signaling molecules that includes thyroid transcription factor 1 (TTF1, now called NKX2.1), TTF2 (now called FOXE1), and PAX8 (1). These transcription factors are expressed in a limited number of other cell types, and so it appears to be the specific combination of transcription factors and possibly non—DNA-binding cofactors acting coordinately that determine the phenotype of the cell. HOXA3 and HHEX also appear to play a role in early thyroid gland organogenesis (2), but the initial inductive signal is unknown.
During caudal migration the pharyngeal region of the thyroid anlage contracts to form a narrow stalk, known as the thyroglossal duct, which subsequently atrophies. Usually no lumen is left in the tract of its descent but, occasionally, an ectopic thyroid and/or persistent thyroglossal duct or cyst forms if thyroid descent is abnormal. In addition to a direct action of FOXE1, caudal translocation of the thyroid anlage arises indirectly, as a result of the growth and expansion of adjacent tissues, including the major blood vessels (3). In late organogenesis, the sonic hedgehog (SHH) gene and its downstream target TBX1 appear to play an important role in the symmetric bilobation of the thyroid (4); SHH also suppresses the ectopic expression of thyroid follicular cells.
In humans, thyroid embryogenesis is largely complete by 10 to 12 weeks’ gestation (5). At this stage, tiny follicle precursors can be seen, iodine binding can be identified and thyroglobulin detected in follicular spaces. Thyroid hormones are detectable in fetal serum by gestational age 11 to 12 weeks, although it is likely that a fraction of the hormones detectable at this early stage is contributed by the mother through transplacental transfer.
Pituitary and Hypothalamus
Like the thyroid, much of our knowledge regarding the early development of the pituitary and the hypothalamus is derived by analogy with rodents. Invagination of the oral ectoderm to form a rudimentary Rathke’s pouch (the buccal precursor of the anterior pituitary gland) and evagination of the ventral diencephalon to form the posterior pituitary are observed by 7 weeks of gestation. Hypothalamic cell and diencephalic fiber tracts do not develop until 15 to 18 weeks and intact hypothalamic–pituitary portal vessels are not observed until 12 to 17 weeks with continued maturation up to 30 to 35 weeks (6). Thus the fetus progresses from a state of combined thyroid–pituitary–hypothalamic immaturity in the first half of gestation to predominantly hypothalamic immaturity later.
Maturation of Thyroid System Control
Thyroid hormone levels begin to rise in the human fetus concomitant with maturation of the hypothalamic–pituitary–thyroid system from midgestation through the neonatal period, with continuing adjustment of the thyroid-stimulating hormone (TSH)/free T4 setpoint during childhood and adolescence. The maturation process involves ontogenesis of the hypothalamus and TRH secretion, pituitary thyrotroph TSH secretion, thyrotroph cell TRH receptors, hypothalamic and pituitary–thyroid hormone receptors, hypothalamic and pituitary iodothyronine monodeiodinase enzyme activities, and thyroid gland TSH receptors and postreceptor response systems (5,6,7) (Table 53.1). The net effect of these ongoing maturational events is reflected in the pattern of change in the prevailing serum TSH, free T4 concentrations, and the TSH/free T4 setpoint (Table 53.2) (8,9).
Table 53.1 Approximate Timing of Hypothalamic-Pituitary Thyroid Maturation Events in the Human Fetus | ||||||||||||||||||||
---|---|---|---|---|---|---|---|---|---|---|---|---|---|---|---|---|---|---|---|---|
|
Table 53.2 Mean Serum Tsh and Free T4 Concentrations at Various Fetal and Postnatal Ages | ||||||||||||||||||||||||||||||||||||||||||||
---|---|---|---|---|---|---|---|---|---|---|---|---|---|---|---|---|---|---|---|---|---|---|---|---|---|---|---|---|---|---|---|---|---|---|---|---|---|---|---|---|---|---|---|---|
|
Figure 53.1 graphically displays maturation of hypothalamic–pituitary–thyroid function during the perinatal period as reflected by the TSH and T4 concentrations versus gestation age. T4, free T4, and TSH concentrations in the fetus increase progressively during the latter half of gestation. The increasing T4 level is due to estrogen-stimulated
hepatic synthesis of thyroxine-binding globulin (TBG). The increasing free T4, which parallels total T4, is due to increased T4 secretion and saturation of TBG-binding sites.
hepatic synthesis of thyroxine-binding globulin (TBG). The increasing free T4, which parallels total T4, is due to increased T4 secretion and saturation of TBG-binding sites.
The molecular events involved within thyroid system maturation are incompletely understood. The increasing free T4 and TSH levels in the fetus (Fig. 53.1 and Table 53.2) presumably reflect an increasing TRH effect and increasing thyroidal TSH responsiveness (10). Hypothalamic TRH synthesis and secretion from the paraventricular nucleus (PVN) is regulated by T3 with modulation by a variety of endogenous and exogenous factors including environmental temperature and metabolic rate mediated by neurotransmitters, including catecholamines, neuropeptide Y, and somatostatin (10). Type 2 iodothyronine deiodinase (D2) is not expressed in the PVN. Rather, T3 is locally generated in glial tanycytes located in the floor and lateral walls of the third ventricle (11). It is postulated that cerebrospinal fluid T4 is the substrate for the tanycyte D2–generated T3 diffusing in a paracrine fashion to the PVN and other hypothalamic nuclei including the arcuate nucleus and median eminence. Pituitary TSH secretion is modulated by TRH and by T3 generated by pituitary D2 which rapidly deiodinates T4 to T3. However, both serum T3 and free T4 appear to be required to suppress TRH mRNA in the PVN (11,12). T4 modulation of pituitary D2 activity via ubiquitination and a high rate of D2 synthesis optimize the T4 feedback mechanism for pituitary TSH secretion (11). The inactivating D3 enzyme also is a key regulator of the hypothalamic–pituitary control axis (12). D3 is expressed in neurons presumably to protect against superphysiologic levels of T3. D3 knockout mice have elevated circulating thyroid hormone levels due to impaired T3 clearance in the neonatal period and develop central hypothyroidism with defective TRH and TSH responsiveness at 2 to 3 weeks of postnatal age (12) (see Chapter 7).
At the level of the hypothalamus, T3 binds to T3 nuclear receptors suppressing prepro-TRH mRNA and TRH synthesis and secretion (13,14). In the pituitary gland T3 binds to the T3 nuclear beta receptor (TRβ2), which, in turn, binds to a thyroid negative response element on the promotor regions of TSHβ and common α subunit genes, inhibiting gene expression and decreasing TSH secretion (15). T3 nuclear alpha receptors (TRα) may have a stimulating effect on TSH secretion; TRα receptor knockout in mice results in failure of TSH secretion in spite of decreased serum T4 concentrations (15). Nuclear receptor cofactors are involved with the T3 nuclear receptors in the mediation of thyroid hormone actions; a defective cofactor has been implicated in a kindred with thyroid hormone resistance (16). It is believed that thyroid hormones modulate TSH secretion via negative feedback while TRH maintains the free T4 setpoint for TSH regulation.
In the developing rat during the period of thyroid system ontogenesis (16 to 18 days’ gestation through 20 to 21 days postnatal), there is a progressive simultaneous maturation of hypothalamic TRH secretion, TRH degrading enzymes, hypothalamic–pituitary TRH and T3 receptors, and hypothalamic and pituitary iodothyronine monodeiodinase activities (17,18,19,20,21,22,23,24,25). At the end of the period of ontogenesis (17 to 18 days), the TSH receptor is first expressed and this is accompanied by significant thyroid growth and evidence of differentiated cell function (26). There is presumed analogous maturation of hypothalamic–pituitary functions in the human fetus during the comparable period of pituitary–thyroid axis development (the latter half of gestation and the early weeks of postnatal life) (5,7). Table 53.2 suggests major phases of thyroid axis control maturation (excluding the neonatal adaptation period): (a) The fetal phase of increasing free T4 and increasing serum TSH levels, and (b) the postnatal phase of relatively stable free T4 levels with decreasing serum TSH values (see later). Maturation of TRH secretion during the fetal phase with progressive reduction of TRH secretion during the postnatal phase would be the simplest hypothesis to explain the observed changes in serum TSH.
During transition from the fetal to the postnatal phase, there is a rapid fall in prevailing TSH concentration (Fig. 53.1, Table 53.2). Fetal serum TSH levels in the third trimester human fetus are relatively high, whereas equilibrated values after 1 to 2 weeks are significantly lower (Table 53.2). The mechanism(s) for the high fetal TSH values as well as the rapid postnatal fall in serum TSH in the absence of significant change in serum free T4 concentrations is unclear. Decreased thyroidal TSH responsiveness or reduced TSH bioactivity probably contribute to the relatively higher fetal serum TSH levels and relatively high TSH/free T4 ratio (Table 53.2) (27). Additionally, fetal serum TRH concentrations are relatively increased due to extrahypothalamic TRH production by placental and fetal gastrointestinal tissues and low serum levels of TRH degrading activity (18,19,20,21,28,29). Also fetal serum T3 concentrations are low due to relatively low feto-placental iodothyronine monodeiodinase type D1 and high monodeiodinase type D3 activities and, by analogy with the fetal sheep, most T4 in the fetus is rapidly converted to inactive sulfated analogs and rT3 (30,31,32). Finally, the lower prevailing serum TSH concentrations postnatally are associated with increased TSH glycosylation and bioactivity, loss of placental TRH production, increased serum TRH degrading activity, increased T3 production with decreased T3 degradation, and decreased production of inactive thyroid hormone analogs (19,20,21,27,28,29,30,31) (Fig. 53.2). The earliest of these changes in term infants is the increase in serum levels of active T3; other extrauterine adaptations are more delayed during the transition period and their relative contributions to the reduction in serum TSH concentrations are not clear.
Maturation of Thyroid Hormone Metabolism
The metabolism of thyroid hormones involves a progressive series of monodeiodinations mediated via three iodothyronine deiodinase enzymes, D1, D2, and D3 (11,32,33,34) (see Chapter 7). These enzymes act to remove an iodine atom from the outer (phenolic) ring or the inner (tyrosyl) ring of the tetraiodothyronine (T4) molecule, respectively, activating or inactivating the hormone. D1 and D2 act as outer ring monodeiodinases deiodinating T4 to active T3. D1 also has inner ring deiodinase activity converting T3 to inactive T2. D3, with inner ring monodeiodinase activity, inactivates T4 to rT3 and T3 to T2. In most tissues, particularly liver and excluding brain and BAT, T3 and rT3, generated via T4 monodeiodination, diffuse rapidly from tissue to interstitial fluid to plasma. In adults under normal circumstances, T3 and rT3 are produced at approximately similar rates. Significant amounts of T3 and small amounts of rT3 also are synthesized and released by the thyroid gland. Thus, the circulating levels of T3 and rT3 reflect both secretion and deiodination of T4 in peripheral tissues. Seventy to ninety percent of
circulating T3 is derived from peripheral conversion and 10% to 25% from the thyroid gland: Respective values for rT3 are 96% to 98% and 2% to 4%. Progressive tissue monodeiodination reactions degrade T3 and rT3 to diiodo, monoiodo, and noniodinated thyronine (see Chapter 7).
circulating T3 is derived from peripheral conversion and 10% to 25% from the thyroid gland: Respective values for rT3 are 96% to 98% and 2% to 4%. Progressive tissue monodeiodination reactions degrade T3 and rT3 to diiodo, monoiodo, and noniodinated thyronine (see Chapter 7).
In contrast to the relatively high T3, low rT3 environment of the adult, rT3 levels are high and T3 levels low in the fetus until 30 to 35 weeks of gestation (Figs. 53.1 and 53.2). Human fetal hepatic D1 activity levels are low throughout gestation, averaging 24% of adult levels, increasing in the perinatal period; D3 levels are high, 10 to 15 times adult levels, decreasing in the perinatal period (34,35,36). D2 activity in human fetal cerebral cortex increases between 13 and 20 weeks of gestation; cerebellar D2 activity increases after midgestation (37). Both D1 and D2 deiodinase species are thyroid hormone responsive. Hepatic D1 activity in the fetal sheep becomes thyroid hormone responsive (i.e., activity increases in response to T4) during the final weeks of gestation; brain D2 is responsive (decreases in response to T4) throughout the final third of gestation (38). The human fetal deiodinases respond similarly to T4. In the hypothyroid human fetus D2 activity in brain tissue increases, and hepatic D1 is suppressed, shunting T4 to the central nervous system and other tissues where it is preferentially deiodinated to T3 by D2 (38). This provides a source of intracellular T3 to those tissues most dependent on T3 during fetal life including brain, pituitary, cochlea, BAT, and keratinocytes. Hepatic D1 enzyme activity (to provide increased serum T3 levels) normally increases during the final weeks of gestation and during postnatal life. The predominance of D3 activity in the fetus, particularly in placenta, liver, and kidney, account for the increased circulating levels of inactive rT3 (30,38,39,40,41). The persistence of high circulating rT3 levels for several weeks in the premature infant indicates that the D3 activities expressed in nonplacental tissues also contribute importantly to thyroid hormone inactivation, maintaining the high fetal circulating rT3 levels. The mixture of D2 and D3 activities in the placenta provides for the conversion of T4 to T3 and of T4 and T3 to rT3 and T2, respectively (30,34,35,38). D2 and D3 activities also have been identified in the uterus and D3 activity in the chorion and amnion (42).
Also in contrast to the adult, bioinactive sulfated iodothyronines are the major thyroid hormone metabolites circulating in the fetus (30,32,43,44) (Fig. 53.2). Sulfotransferase enzymes are present early in fetal life in placenta and in fetal liver, lungs, and brain. Sulfation of the phenolic hydroxyl group of the iodothyronine molecule appears to be a normal prerequisite step for monodeiodination (43). The sulfated iodothyronines are preferred substrates for D1 and concentrations are high in fetal serum in part because of low fetal D1 activity (30). Increased production of sulfated metabolites also contributes to high fetal serum levels. In the fetal sheep during the last third of gestation, the production rate of T4 approximates 40 μg/kg/d, several fold greater than in adult animals (32). The production rates of inactive rT3, and sulfated analogs T4S, rT3S, and T3S, approximate 5, 10, 12, and 2 μg/kg/d, respectively, accounting for the large majority of T4 metabolized daily in the euthyroid fetus (32). T2S, T1S, and triac sulfate also are significant fetal thyroid hormone metabolites (45,46). There is evidence that T3S may have biologic activity (suppresses TSH) in vivo, suggesting that it can be desulfated by one or more tissue sulfatase enzymes (34). There is limited information regarding production and significance of acetic acid, glucuronide conjugated or the recently characterized thyronamine derivatives of the thyroid hormones in the fetus (47,48).
Maturation of Thyroid Hormone Transport and Actions
Maturation of Thyroid Hormone Transport
Thyroid hormone actions at the cellular level require thyroid hormone access to tissue cells. It had long been assumed that thyroid hormones passively diffuse into cells. Recently, several classes of cell membrane iodothyronine transporters have been described. These transporters belong to several families of organic anion, amino acid, and monocarboxylate solute carriers, including the organic anion transporting polypeptide (OATP) family, the solute carrier family 21 (SLC21), and the system L transporters (LAT) (49,50,51,52). Mutations of the human monocarboxylate transporter 8 (MCT8), a member of the SLC21 family shown to be a specific thyroid hormone transporter present in developing brain, leads to a syndrome of combined thyroid dysfunction and psychomotor retardation
(49,50). MCT8 is expressed in many tissues including kidney, heart, skeletal muscles, brain, and thyroid; MCT10 is expressed in intestine, kidney, liver, skeletal muscle, heart, and placenta (49,50). Most OATP proteins are expressed in multiple tissues including liver, kidney, brain, lungs, heart, placenta, testis, eye, and small intestine. However, some show more restricted distribution. The OAT1 subfamily of transporters appear to be most important for thyroid hormone transport (50). MCT8, MCT10, OATP1A2, LAT1, and LAT2 have been described in placenta from early in the first trimester with varying patterns of mRNA expression throughout gestation (51,52). The ontogenesis and significance of these plasma membrane thyroid hormone transporters in fetal development remain to be further defined.
(49,50). MCT8 is expressed in many tissues including kidney, heart, skeletal muscles, brain, and thyroid; MCT10 is expressed in intestine, kidney, liver, skeletal muscle, heart, and placenta (49,50). Most OATP proteins are expressed in multiple tissues including liver, kidney, brain, lungs, heart, placenta, testis, eye, and small intestine. However, some show more restricted distribution. The OAT1 subfamily of transporters appear to be most important for thyroid hormone transport (50). MCT8, MCT10, OATP1A2, LAT1, and LAT2 have been described in placenta from early in the first trimester with varying patterns of mRNA expression throughout gestation (51,52). The ontogenesis and significance of these plasma membrane thyroid hormone transporters in fetal development remain to be further defined.
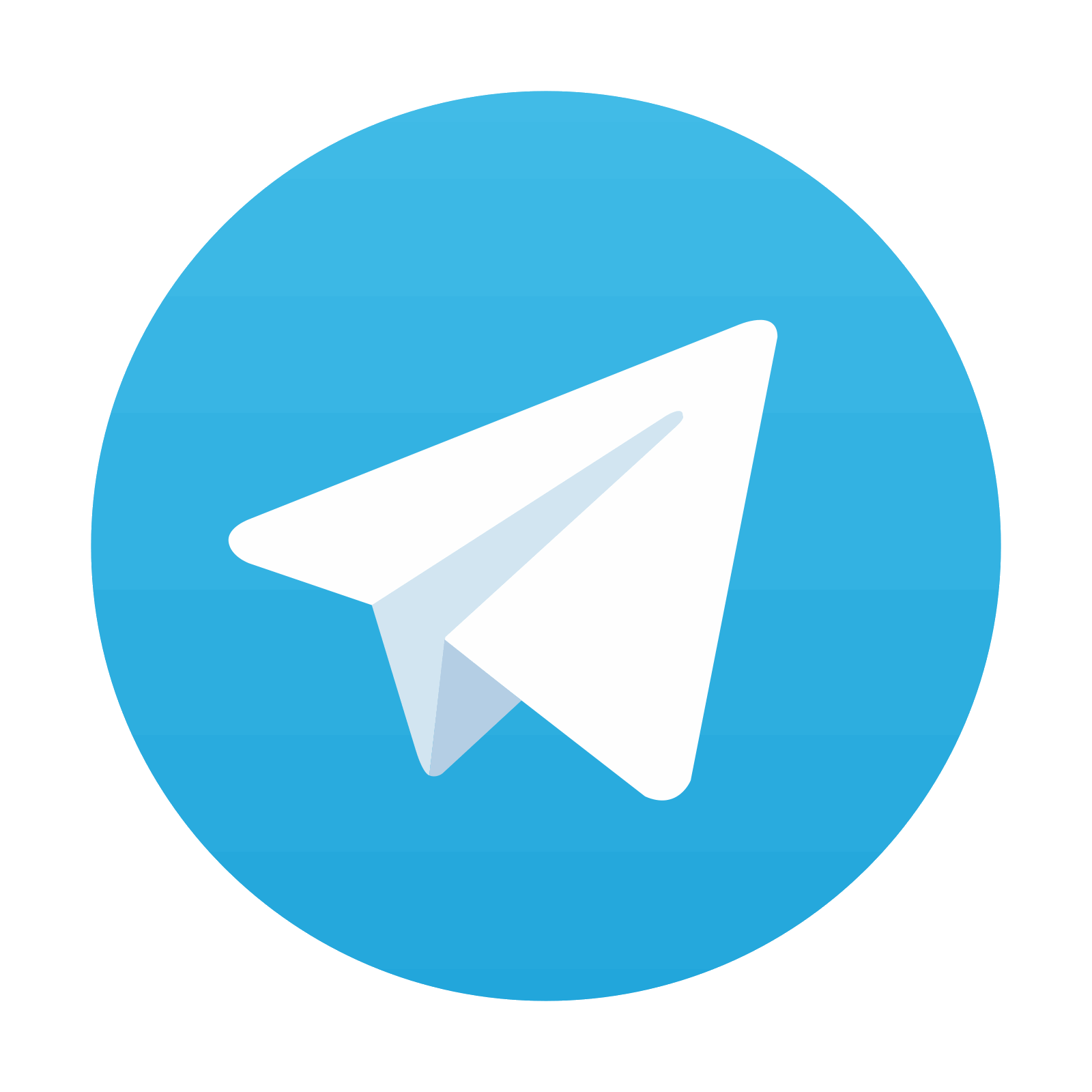
Stay updated, free articles. Join our Telegram channel
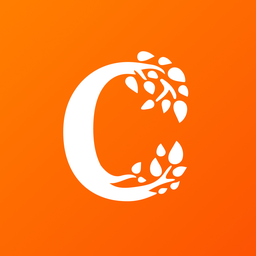
Full access? Get Clinical Tree
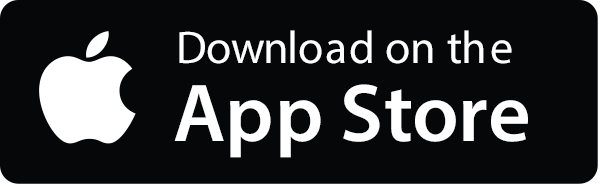
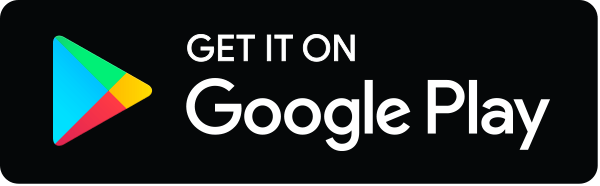