Fig. 5.1
Efficacy study of S-1 in four gastric PDX models. a and b show that GAPF155 and GAPF157 were sensitive to S-1; c and d show that GAPF161 and GAPF187 were not sensitive to S-1
PDX Models May Fail to Fully Account for Many Non-cell-Autonomous Drivers of Heterogeneity
PDXs are arguably the best models of tumor heterogeneity and therefore perhaps the most powerful tools for investigating tumor biology. Although PDX models maintain the genomic architecture, histology, and drug responsiveness of the original patients’ tumors, the clonal profiles and tumor microenvironment of PDX tumors can change during their propagation in immunodeficient mice. Analysis of genome-wide variant allele frequencies in serial passages of PDX tumors showed that clonal selection occurs more frequently in initial engraftment steps than in propagation steps, but the detailed clonal dynamics differ depending on the various tumor samples of the same tumor type [35]. The clonal dynamics in PDX tumors is probably generated by selection acting on preexisting clones rather than by generation of new clones [36]. As a result, it is probable that the more aggressive clones become dominant in PDX tumors, and, in some cases, PDX models indeed showed the genomic and transcriptomic signature of metastatic and relapsed cancers [37]. These aggressive clones could be particularly important targets in cancer therapy.
As well, stromal and immune interactions in PDXs may be altered by cellular component deficiencies and interspecies compatibility in host models. However, the tumor microenvironment has long been known to play an essential role in tumor progression, and its role in drug response is becoming apparent [38, 39]. Aside from clonal dynamics driven by intrinsic differences in a cell’s genetic or epigenetic background, intra-tumor heterogeneity can be influenced by tumor-extrinsic factors in the non-cell-autonomous compartment [40]. Cellular interactions with the extracellular matrix (ECM) can alter gene expression programs, drive differentiation, and profoundly alter cell behavior. As cancers develop, tight regulation of the ECM is lost and tissue architecture begins to degrade [38]. A study by Wang and colleagues [41] provides direct evidence that ECM-dependent signaling confers dynamic switching between transforming growth factor b receptor 3 (TGFBR3) and jun D proto-oncogene (JUND)-related expression signatures. ECM-driven oscillations between signaling pathways such as those described could have profound effects on propensity to malignancy. Furthermore, solid-state ECM interactions are necessary for cells to maintain stem cell properties, and regulated ECM helps maintain the stem cell niche [42]. In PDX models, Matrigel is often used to increase the engraftment efficiency; however, it is worth noting that this is a murine basement membrane extract, and suitable synthetic human alternatives are available. The presence of growth factors in Matrigel may favor the engraftment of one cell type over another. Finally, as ECM structure is tissue specific [42], researchers should consider the use of orthotopic transplantations where possible.
The tumor microenvironment is further characterized by an influx of stromal cells. Infiltrating cancer-associated fibroblasts (CAF) can often confer resistance to cytotoxic and targeted therapies [39]. Because of the high levels of CAF infiltrates seen in some tumor types, heterogeneity within their population would undoubtedly confer differential properties to the tumor bulk. In PDX models, human stromal cells are gradually replaced by murine equivalents upon engraftment in the mouse, suggesting that implanted human cancer cells retain the ability to recruit murine accessory cells to their niche. However, it should be noted that some differences exist between ligand repertoires of human and murine fibroblasts. Clearly, stromal architecture and activity are mimicked in the murine host; however, it is currently unclear how this reflects human stroma with regard to supporting tumor growth and development.
The immune system also plays a crucial role in tumor progression, and perhaps it is the most obvious disadvantage in PDX models, because of engraftment into severely immune-deficient host animals. Tumor cells are broadly thought to be antigenic which emerge point mutations in coding exons in a developed tumor and result in a large repertoire of neoantigens. Targeting of these neoantigens can lead to significant CD8+cytotoxic T-cell infiltration and tumor cell death. However, most tumors eventually progress and evade the immune system often through the dominant inhibitory effects of suppressive pathways (the so-called immune checkpoints such as CTLA-4/B7 and PD-1/PD-L1). This is supported by the prognostic value of the CD8+ to FOXP3+ (cytotoxic to regulatory T-cell, Treg) ratio in many solid tumors and the recently reported clinical efficacy of a variety of checkpoint inhibitors [43, 44]. The proinflammatory microenvironment established by M1-polarized tumor-associated macrophages (TAM), CD8+T-cells, NK cells, and others can lead to the recruitment of numerous immune-suppressive components. In addition, CD4+T-cell and macrophage recruitment following intensive chemotherapy in breast cancer patients is associated with significantly reduced recurrence-free survival [44].
All in all, heterogeneity within a tumor is governed by both cell-autonomous (e.g., genetic and epigenetic heterogeneity) and non-cell-autonomous (e.g., stromal heterogeneity) drivers. Although PDXs can largely recapitulate the genomic architecture, histology, and drug responsiveness of human tumors, they may not fully account for heterogeneity in the tumor microenvironment. However, these models have substantial utility in basic and translational research in cancer biology, but study of stromal or immune drivers of tumor progression may be limited. Similarly, PDX models offer the ability to conduct in vivo and ex vivo patient-specific drug screens, but stromal contributions to treatment responses may be underrepresented.
References
1.
Gao H, Korn JM, Ferretti S, Monahan JE, Wang Y, Singh M, et al. High-throughput screening using patient-derived tumor xenografts to predict clinical trial drug response. Nat Med. 2015;21(11):1318–25. doi:10.1038/nm.3954.CrossRefPubMed
2.
Sausville EA, Burger AM. Contributions of human tumor xenografts to anticancer drug development. Cancer Res. 2006;66(7):3351–3354. discussion 4. doi:10.1158/0008-5472.CAN-05-3627.
3.
Siolas D, Hannon GJ. Patient-derived tumor xenografts: transforming clinical samples into mouse models. Cancer Res. 2013;73(17):5315–9. doi:10.1158/0008-5472.CAN-13-1069.CrossRefPubMedPubMedCentral
4.
Guenot D, Guerin E, Aguillon-Romain S, Pencreach E, Schneider A, Neuville A, et al. Primary tumour genetic alterations and intra-tumoral heterogeneity are maintained in xenografts of human colon cancers showing chromosome instability. J Pathol. 2006;208(5):643–52. doi:10.1002/path.1936.CrossRefPubMed
5.
Dobbin ZC, Katre AA, Steg AD, Erickson BK, Shah MM, Alvarez RD, et al. Using heterogeneity of the patient-derived xenograft model to identify the chemoresistant population in ovarian cancer. Oncotarget. 2014;5(18):8750–64. doi:10.18632/oncotarget.2373.CrossRefPubMedPubMedCentral
6.
Chapuy B, Cheng H, Watahiki A, Ducar MD, Tan Y, Chen L, et al. Diffuse large B-cell lymphoma patient-derived xenograft models capture the molecular and biological heterogeneity of the disease. Blood. 2016;127(18):2203–13. doi:10.1182/blood-2015-09-672352.CrossRefPubMedPubMedCentral
7.
Seol HS, Kang HJ, Lee SI, Kim NE, Kim TI, Chun SM, et al. Development and characterization of a colon PDX model that reproduces drug responsiveness and the mutation profiles of its original tumor. Cancer Lett. 2014;345(1):56–64. doi:10.1016/j.canlet.2013.11.010.CrossRefPubMed
8.
Kim MP, Evans DB, Wang H, Abbruzzese JL, Fleming JB, Gallick GE. Generation of orthotopic and heterotopic human pancreatic cancer xenografts in immunodeficient mice. Nat Protoc. 2009;4(11):1670–80. doi:10.1038/nprot.2009.171.CrossRefPubMedPubMedCentral
9.
Fleming JM, Miller TC, Meyer MJ, Ginsburg E, Vonderhaar BK. Local regulation of human breast xenograft models. J Cell Physiol. 2010;224(3):795–806. doi:10.1002/jcp.22190.CrossRefPubMedPubMedCentral
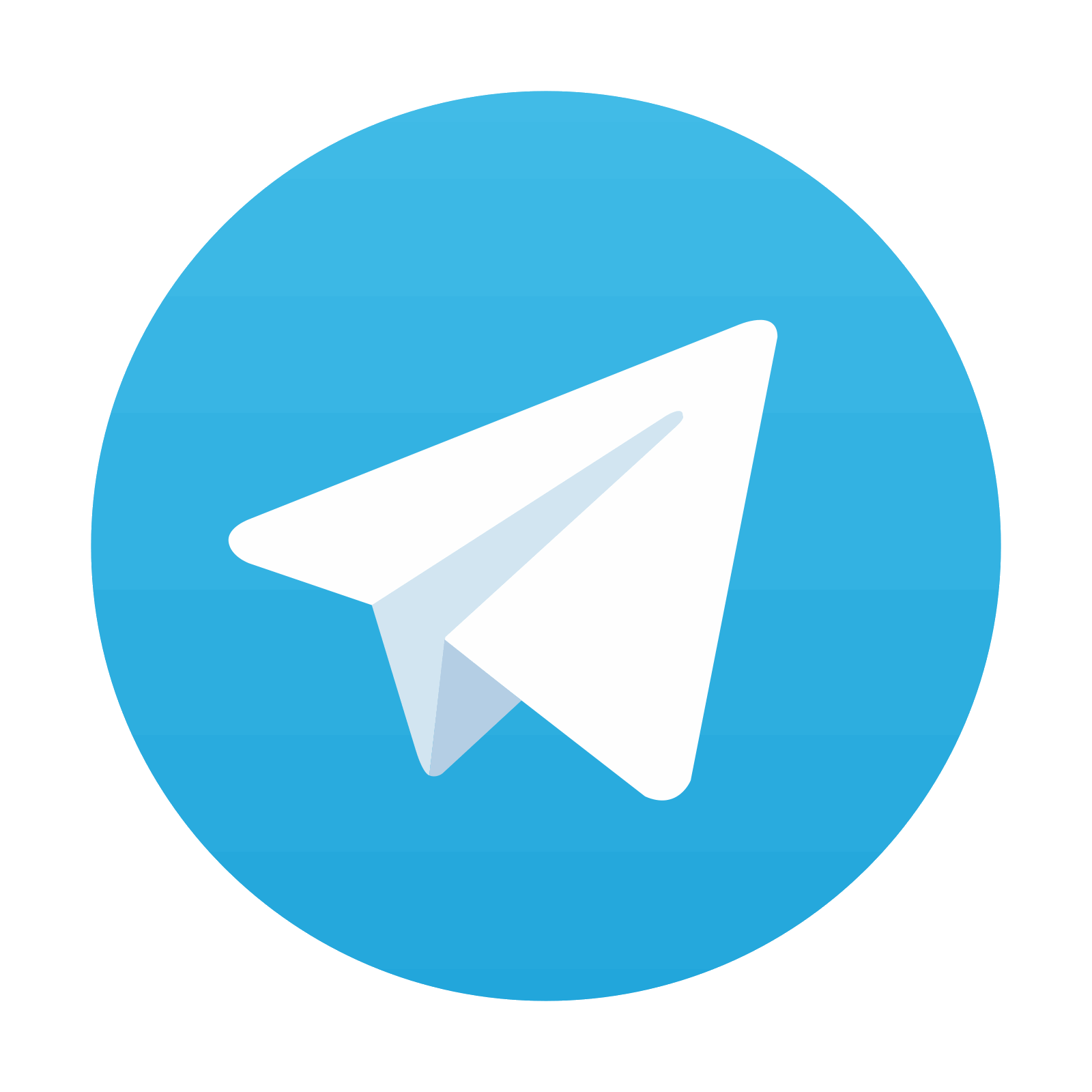
Stay updated, free articles. Join our Telegram channel
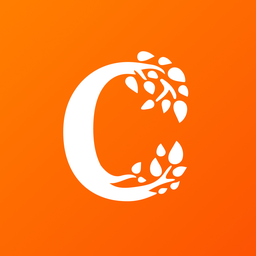
Full access? Get Clinical Tree
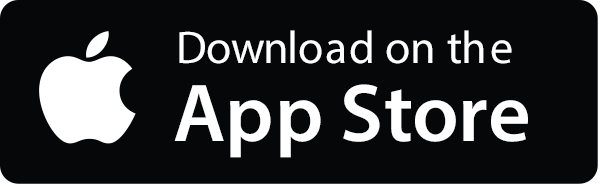
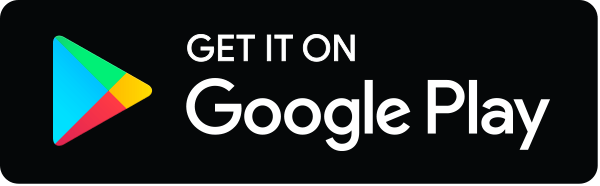