At this point. it is worth noting that amphibians have historically been underrepresented in the ecotoxicology literature [20]. While development of test methods for detecting endocrine disruption in fishes and birds has benefited from a considerable knowledge base developed through their routine use ecotoxicological risk assessment, the foundations for amphibian test methods could be said to be more limited and more disjointed—for example, in terms of test species, exposure systems, and assessment endpoints. This is particularly true with respect to urodeles (newts and salamanders), and it has been suggested that this is cause for concern and action. Urodeles exhibit some significant differences in endocrine regulation and reproductive physiology from anurans, and this could lead to uncertainties in extrapolating information from anuran test models to wild populations of newts or salamanders. This review is therefore restricted to anuran amphibians.
8.3 EMBRYONIC DEVELOPMENT
With the exception of a limited number of anuran species that exhibit ovoviviparity or other specialized forms of parental care [21], embryonic development in amphibians occurs protected only by egg jelly, and egg masses are in contact with water in surface water bodies in which adults breed. There is therefore a potential for amphibians in early life stages—that is, from egg deposition—to be exposed to environmental contaminants in water bodies used for breeding. Consequently, a variety of known or potential environmental contaminants have been tested for adverse effects on embryonic development in various anuran species [22–24] as part of ecological risk assessment in relation to various industries or activities.
Perhaps owing to the greater ease with which adult African clawed frogs (Xenopus laevis) can be maintained in captivity as broodstock, many of these studies assessing the effects of chemical exposure on embryonic development have used Xenopus embryos [25–31]. This convenience of Xenopus as a laboratory amphibian model and the extensive knowledge of its early development as an embryological model led to the development of the Frog Embryo Teratogenesis Assay—Xenopus (FETAX). This assay was developed as a potential alternative to mammalian models for detection of developmental toxicants in chemical safety assessment. The test comprises a short exposure (96 h) of midblastula/early gastrula embryos to test chemicals in a defined reconstituted water matrix in a semistatic exposure system. Test medium is replaced every 24 hours, at which point incidence of embryo mortality is scored. At the end of the test (at 96 h), the surviving larvae are assessed macroscopically for developmental abnormalities (malformations) and may be measured (whole body length) to give an indication of growth [32]. The test therefore generates three main end points: LC50, ED50(malformation), and minimum inhibitory concentration for growth (MICG).
Given the early exposure window of FETAX, between Nieuwkoop and Faber (N&F) [33] stages 8 to 11 (midblastula to early gastrula) and 46 (early hind-limb bud), it is unlikely that the assay would be able to provide any sensitive and specific means of detecting ED activity in test chemicals. Endpoints suitable for detection of estrogenic or thyroid activity, as will be discussed in Section 8.4 and Section 8.5, cannot be incorporated into FETAX or any other embryological exposure model with Xenopus or any other amphibian, as the target systems are not sufficiently developed within such early developmental windows. Moreover, there is little if any evidence in the peer-reviewed literature that mechanistically specific effects of hormones or EDCs can be detected in early life stage exposures of amphibian embryos.
With respect to estrogens (i.e., agonists/antagonists of the ER), several studies have assessed the impacts of exposure to natural (estradiol-17β, E2), synthetic (diethylstilbestrol, DES; 17α-ethynylestradiol, EE2) or xeno-estrogens (octylphenol, OP; nonylphenol, NP; bisphenol-A, BPA) on gross morphology and organogenesis. Within the limitations of the early embryonic exposure window used (comparable to FETAX), these endpoints are affected by E2 only at concentrations in the micromolar range (i.e., significantly higher than the natural physiological range of activity in later life stages) [34–36].
In more recent studies, effects of several xeno-estrogens on gross developmental, histological, and molecular end points in early Xenopus embryos have been assessed. Bevan et al. [37] exposed Xenopus embryos from stage 10.5 through to 37 (around 48 h of exposure) to E2, 17α-methyltestosterone (MT), p,p′-DDE, and methoxychlor (MXC) at concentrations up to 10 μM without significant increases in mortality, although above 5 μM of E2 most embryos appeared moribund. In contrast, OP and NP resulted in significant mortality at micromolar concentrations. Gross malformations including dorsal flexure and abnormal cement glands were observed in embryos exposed to E2, MXC, OP, and NP at 5 μM, broadly consistent with observations by Nishimura et al. [35]. Effects on neural crest–derived melanocytes and TUNEL staining (indicating apoptosis) of neural crest–derived cells, particularly on the cranium, were observed in embryos exposed to lower concentrations (high nM range) of E2, MXC, and NP. Interestingly, the effects of NP on both dorsal flexure and melanocyte differentiation could be partially blocked by the ER antagonist ICI 182,780, indicating an ER-mediated mechanism, while the effects of MXC exposure (on melanocytes) were unaffected by the ER antagonist [37]. Oka et al. [38] reported abnormalities at neural tube closure, tail-bud stage, and gross deformities (small head, swollen abdomen, crooked vertebrae) in Xenopus embryos exposed from stage 6 (early blastula) to the weak xeno-estrogen BPA at 20 μM, with mortality at gastrulation in embryos exposed to 40 to 100 μM. Embryos exposed to 20 μM BPA exhibited typical apoptotic features in central nervous system cells, which were not observed in embryos exposed to E2 at 10 μM; the latter exhibited head and abdominal malformations only by stage 33/34 (early tadpole). Embryos exposed to E2 at 1 μM appeared normal—apparently the effects of BPA on CNS were not strictly estrogenic in nature.
The gross and molecular end points used in these studies were assessed after short-term early life stage exposure and overall indicated that only high concentrations of E2 (micromolar range, much higher than the concentrations at which estrogens typically exert their effects) exhibited significant effects on the endpoints measured within the time scale of the studies. Grow-out of larvae into postembryonic development, completion of metamorphosis or beyond, may have enabled detection of other organizational effects of embryonic estrogen exposure at lower concentrations. However, these studies indicate that within an acute exposure window during embryonic development, Xenopus embryos as an amphibian model are not particularly sensitive to estrogens.
Several fungicides and herbicides with potential thyroid-disrupting activity have also been tested in embryonic exposures with Xenopus. Birch and Prahlad [26] assessed toxic/teratogenic potential of the fungicide Nabam (ethylenebisthiocarbamate disodium salt) in relation to time in solution, observing that both lethality and effects on the notochord declined with storage time, indicating that the agent, believed to be a degradation product of Nabam, is unstable in aqueous solution. However, one such degradation product, ethylene thiourea (ETU), which is attributed with thyroid activity, had no effect on mortality or incidence of abnormality in this study at concentrations up to 10 mg/L. This likely reflects the specific mode of action of ETU (inhibition of thyroid peroxidase; see Sections 8.5.1.1 and 8.5.1.2 on amphibian metamorphosis assay) and the fact that the thyroid system is not functioning and sensitive to this type of agent at this early life stage. Gutleb et al. conducted prolonged -FETAX type studies with X. laevis, with exposure to polychlorinated biphenyls (PCBs; 7.7 pM–6.4 μM) in the embryonic development window used by FETAX, but with assessment of mortality growth and rate of development through an extended nonexposure period (80 days). PCB exposure during embryonic development did not result in significant alterations in growth or larval development (time until completion of metamorphosis), although, interestingly, tissue concentrations of TH in the lower jaw were lower in individuals exposed to PCB compared to controls [39].
The studies reviewed above indicate the relative insensitivity to endocrine disrupters of assessment endpoints that are amenable to use in early life stage tests with Xenopus (as a model amphibian embryo). The FETAX assay is therefore not a candidate test method for detecting EDCs in amphibians. Moreover, in reviewing the suitability of FETAX for wider application in ecological risk assessment, Hoke and Ankley [40] noted a number of methodological shortcomings of the FETAX method as typically conducted, including criteria for and control of water quality characteristics, biological loading rate, exposure system, analytical verification of test concentrations, and relevance and competence of exogenous metabolic activation systems. The utility of FETAX for ecological risk assessment purposes has also been called into question on grounds that divergent water quality chaaracteristics of complex environmental samples generate confounding factors, and existing acute assays in other aquatic species may in any case be more protective of amphibian fauna [40].
8.4 HATCHING
Egg hatching is clearly a key developmental milestone for amphibians and marks the division between development and postembryonic development. Duration of embryonic development and the timing of hatching obviously depends on species, temperature, and other stressors. In the model amphibian X. laevis reared in laboratory conditions (e.g., 23 ± 3°C) as prescribed in FETAX protocol, [32], hatching typically occurs one to two days after egg deposition, while it may take several weeks in temperate European species such as the common toad (Bufo bufo) in colder conditions in the wild. In cases where effects of EDCs have been assessed in fish ecotoxicology tests, such as the Fish Full Life Cycle, hatching is assessed as a standard endpoint, and there has been some interest in developing test guidelines, such as Organisation for Economic Cooperation and Development (OECD) 210 (Fish Early Life Stage Test, ELS) for endocrine disrupter testing.
Several ecotoxicological studies with amphibian species have assessed hatching success as an endpoint, although few if any of these specifically concern detection of EDCs. Greulich and Pflugmacher [41] and Mahaney [42] assessed impacts of α-cypermethrin exposure at various life stages of the northern temperate anuran species Rana arvalis and reported reduction in hatching success at nominal concentrations of 1.0 and 10 mg/L. However, reduced hatching success correlated with poor avoidance response of hatched larvae and other behavioral abnormalities, suggesting that adverse effects of α-cypermethrin at this life stage related to neurotoxicity (as would be expected given its mode of action) rather than ED activity. Although a number of publications in recent years have suggested that the herbicide atrazine is an EDC interfering with aromatase activity [43–45] that exerts a variety of adverse effects on amphibians [46,47], Allran and Karasov [48] reported no effect of atrazine on hatching in R. pipiens, R. sylvatica, or B. americanus.
8.5 LARVAL DEVELOPMENT
Due to obvious concerns over contamination of the aquatic environment and the tractability of focusing on aquatic phases of the amphibian life cycle, much work on effects of environmental contaminants on amphibians has assessed impacts on larval survival, growth, development, and in some cases also behavior. Early studies in temperate (e.g., Ranid) species or X. laevis often assessed effects of single chemicals (in some cases mixtures) in several exposure windows from embryo, through hatching, to larval development and completion of metamorphosis [23,49].
A number of studies have assessed effects on growth and development of anuran larvae at various stages in response to exposure to pesticides, including organochlorines [50–54], organophosphates [54–58], carbamates [55–61], and pyrethroids [62,63]. Few if any of these studies explicitly considered ED modes of action, and end points are typically apical in nature. However, several studies noted delay in metamorphosis in chronic larval exposures [53,54,64–68], which might have suggested interactions with the hypothalamic-pituitary-thyroid (HPT) system that regulates amphibian metamorphosis.
8.5.1 Anuran Metamorphosis
Postembryonic development in anuran amphibians comprises a well-characterized and dramatic transition from a legless, tailed, gill-breathing, and generally herbivorous swimming larva to a lung-breathing, predatory, tailless tetrapod and is probably the most dramatic example of metamorphosis among vertebrates. The process is obligatorily dependent on TH, and anuran larvae such as those of Xenopus start to fix iodine in the developing thyroid tissue a short time after the initiation of independent feeding [69]. This onset of TH synthesis follows earlier low-level expression of TH receptors (TRs), primarily TRα, and marks the transition from premetamorphic to prometamorphic development, in which increasing levels of TH orchestrate degeneration of larval tissues, development of adult tissues, and modifications of other common organs and systems, to adapt to adult existence. TH regulation of anuran metamorphosis is also modulated by the stress hormone corticosterone (B) and prolactin (PRL). B appears to have a bimodal effect on anuran larval development [70,71]. Premetamorphic development is inhibited by B, presumably through feedback inhibition on corticotrophin-releasing factor [72], which in anurans actually takes the role of thyrotrophin-releasing hormone [73]. In later stages of metamorphosis, however, B appears to potentiate TH-stimulated transformations independent of interactions with the HPT axis and may involve changes of gene expression (e.g., monodeiodinases and effector genes such as stromolysin) and modulation of PRL expression [74]. While PRL inhibits tail resorption, primarily through TR antagonism and prevention of TR auto–up-regulation [75,76], its action does not represent a true juvenile hormone; rather its inhibitory effects counterbalance the increasing concentrations of stimulatory THs through metamorphic climax to coordinate a sequence of tissue transformations by modifying target tissue responses [77].
The transformations regulated by TH in anuran larvae are an exquisite model of how hormones can control postembryonic development [78] and also provide a generalized vertebrate model for TH function. Principal regulatory interactions of the anuran HPT axis are shown in Figure 8.2.
FIGURE 8.2 Schematic representation of principal regulatory interactions in the amphibian hypothalamic-pituitary-thyroid axis. CNS—central nervous system, CRF—corticotrophin-releasing factor (which largely replaces the role of thyroid hormone-releasing hormone, THRH, in amphibians), MDI—monodeiodinase, T4—thyroxine, T3—triiodothyronine, T2— diiodothyronine (inactive), rT3—reverse triiodothyronine, TSH—thyroid-stimulating hormone. Note: + within an arrowhead indicates positive feedback, – within an arrowhead indicates negative feedback inhibition.
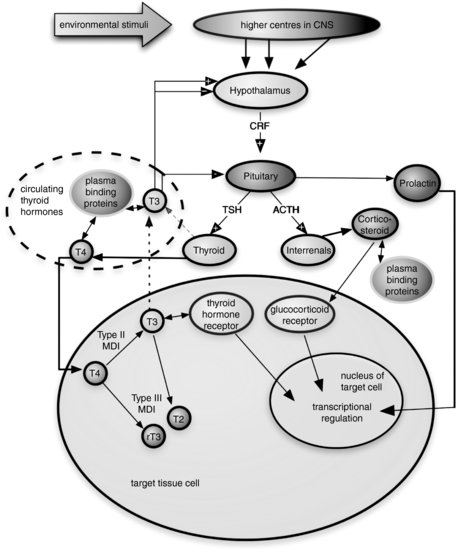
A number natural and synthetic chemicals found in the environment can impair TH homeostasis in humans and wildlife [79]. These thyroid-active chemicals, or thyroid disrupters, comprise something of a mixed bag of mechanisms of action, including:
- Competitive inhibition of iodide uptake into the thyroid gland
- Inhibition of synthesis of TH in the thyroid gland by thyroid peroxidase (TPO)
- Displacement of circulating TH from plasma carrier proteins, resulting in increased clearance
- Increased metabolism and clearance of circulating TH through induction of mixed-function oxygenases
- Alterations in peripheral TH metabolism, for example, conversion of thyroxine [T4] to the more active triiodothyronine [T3] or the inactive reverse triiodothyronine [rT3]
More recent evidence indicates the potential for some synthetic chemicals to interfere with the interaction of T3 with the TR or to disrupt of postreceptor signaling [80–84].
This variety of mechanisms by which chemicals might interfere with TH function in receptor species presents a difficulty for endocrine disrupter screening and testing frameworks, at least in the hazard identification phase, as no single in vitro screen would be competent to detect all potential modes of thyroid-disrupting activity [85]. As a result, in developing tool kits for EDC screening and testing, regulators have promoted development of in vivo methods for detecting (i.e., at screening level) thyroid-disrupting activity in order to minimize false negatives through epistemic uncertainty about mechanisms by which chemicals might disturb thyroid axis function [86,87]. In parallel with initiatives to adapt standard mammalian toxicology methods (e.g., with rats) to provide more specific information on thyroid effects, studies demonstrating the apparent sensitivity of amphibian metamorphosis to thyroid-disrupting chemicals [65,66,88] stimulated interest in development and validation of an amphibian metamorphosis assay for detecting this mode of activity.
8.5.1.1 Metamorphosis Assays
The potential for use of anuran metamorphosis as a model for detection of thyroid-disrupting chemicals within endocrine screening and testing programs was recognized during the EDSTAC process in the United States [89] and by OECD through its Endocrine Disrupters Testing and Assessment (EDTA) task force activity, which recommended further development of an amphibian metamorphosis assay (AMA). To support this activity, an Expert Group on Endocrine Disrupters Testing in Amphibians was convened [90], with the primary remit to develop an AMA fit for the purpose of detecting thyroid-active chemicals as part of an endocrine disrupter screening and testing framework. The anticipated use for such an assay was (originally) at screening level, for detection of thyroid active chemicals rather than to represent amphibians as a group in the screening and testing framework [90]. This is not to say, of course, that an AMA such as has emerged from the OECD test guideline development could not be used in higher tiers of an ED screening and testing framework, as a partial life cycle test for hazard characterization of chemicals identified as thyroid active in screening stages.
The development and validation activity that has led to the development of an AMA in the African clawed frog (X. laevis) has benefited from research conducted in academic, industrial, and governmental laboratories, among a number of OECD member countries, and has encompassed two main approaches. Figure 8.3 provides a graphic representation of how these approaches relate to the well-characterized changes in morphology and circulating TH concentrations in Xenopus larvae.
FIGURE 8.3 Graphic representation of how candidate amphibian metamorphosis assays for detecting thyroid chemicals relate to the development of Xenopus laevis. The solid line indicates changes in tail length through larval development, while the dashed line indicates changes in circulating thyroid hormone concentrations. Pre-metamorphic development is indicated by no shading, pro-metamorphic development by light gray shading, and metamorphic climax by darker grey shading. Developmental stages on the x axis are after Nieuwkoop and Faber [33], and units on the y axis are arbitrary.
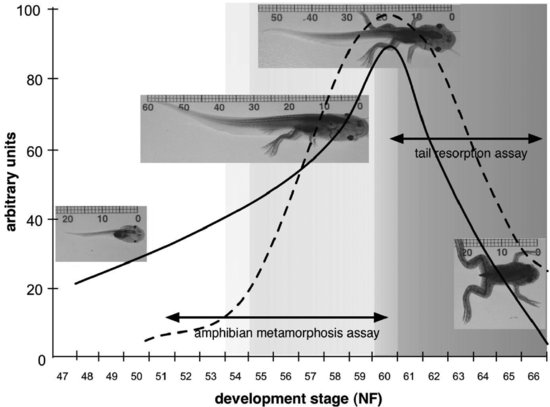
8.5.1.2 Metamorphic Climax: Tail Resorption Assay
In the EDSTAC process, it was suggested that the rate of tail resorption in Xenopus larvae exposed to test substances could be used as morphological proxy for thyroid status and thereby used to detect the action of thyroid disrupters [89,91]. This followed publication of a study by Fort et al. [91] designed specifically to address this need, with a short exposure window (14 days, from N&F stages 60–66) and using computer-aided video imaging to process the primary metamorphic end point (tail resorption).
It has since been argued that tail resorption actually provides a poor exposure window for detecting thyroid-active chemicals, as circulating concentrations of TH are so high by this stage of development that they would swamp any effect of mild agonists [92]. Interestingly, more recent studies on the tissue distribution and timing of monodeiodinase (MDI) expression have shown that several key tissue transformations are regulated by local availability of T3, the active hormone, by way of local action of MDI. Circulating T4 concentrations escalate through prometamorphosis, in the absence of a complete feedback loop because Type II MDI (5′MDI; which converts the prohormone T4 to T3) is not expressed in pituitary cells until metamorphic climax. Onset of expression of 5′MDI in the pituitary facilitates T3-mediated inhibition of thyroid-stimulating hormone secretion, and thus circulating concentrations of TH start to decline to postmetamorphic levels [93]. Expression of 5′MDI in the tail is also delayed until early metamorphic climax, and until this time the tail tissue does not respond to rising concentrations of T4 [69]. Exposure of larvae to TR agonists early in metamorphic climax (e.g., from N&F stages 58–60) could therefore be expected to result in acceleration of tail resorption, on the assumption that xenobiotic TR agonists would not require conversion by local 5′MDI and that tissue levels of the test chemical might stimulate tail resorption before it would be initiated naturally by locally generated T3. This might explain why several studies report accelerated tail resorption in this exposure window [64,68,91], although more rigorous use of known TR agonists (i.e., T4, T3) would have been informative and would have allowed better assessment of the potential sensitivity of this approach to detecting TR agonists.
Aside from the issue of detecting TR agonists/antagonists (of which we have relatively few examples among synthetic/environmental chemicals) is the issue of sensitivity to inhibitors of TH synthesis, or chemicals that reduce circulating levels of TH, of which we have far more well-characterized examples. For these agents, we may expect a tail resorption assay to be relatively insensitive, as the tail resorption process is facilitated by local conversion of high circulating concentrations of T4, so the level of upstream inhibition of T4 synthesis in the thyroid would need to be dramatic before it would be manifest in the rate of tail resorption. Several studies by the Stover Group have reported effects on tail resorption by several xenobiotics, including sufonyl meturon, nicosulfuron, pentachlorophenol (PCP), and NP, although the mechanism is not always clear [64,68,91]. PCP is reported to inhibit tail resorption in a manner that is not reversible by T4 replacement, suggesting perhaps an effect of PCP on Type II MDI activity in tail tissue. While there is some evidence of inhibition of conjugation of THs (e.g., sulfation and glucuronidation of the inactive T2 [94]), it is not clear whether this would explain the actions of PCP on tail resorption reported by Fort et al. [64]. In contrast, NP is reported to accelerate tail resorption [91], though there are few if any reports of TR agonist activity of alkylphenols. Moreover, NP is reported to inhibit thyroid peroxidase in rodents [95], while several studies have reported reduced circulating T4 concentrations and increased size of thyroid follicles (indicative of a compensatory response to reduced TH secretion) in fish exposed to NP [96,97].
An EPA-led analysis of the tail resorption assay proposed by EDSTAC concluded that the assay was not fit for purpose [98]. This decision is largely empirically based but also recognizes a number of practical issues with this assay (e.g., obtaining sufficient numbers of larvae synchronized at an advanced stage of development). These issues notwithstanding, more recently several groups have reported inhibition tail resorption, either spontaneous or induced by induced by T3, in anuran larvae exposed to BPA or the BPA derivatives tetrabromobisphenol A (TBBPA), tetrachlorobisphenol A (TCBPA). and tetramethylbisphenol A (TMBPA) [34,83,99]. These findings, in particular the ability to inhibit tail resorption induced by exogenous T3, suggest that these compounds are able to interfere with the binding of T3 to its receptor in target tissue (i.e., the tail) or to block postreceptor signaling events. If BPA, or these derivatives, was acting at the level of TH synthesis or local conversion of T4 to T3, we would not expect them to inhibit the tail resorption response to exogenous T3. This interpretation is also supported by reports that these chemicals can inhibit T3-induced tail resorption in vitro, in tail explant cultures [34,100]. These observations lend support to the idea that the (loose) group of chemicals that may be considered as thyroid disrupters may include agonists/antagonists of the TR. As a result, any assay designed for screening chemicals for thyroid-disrupting activity should cater for these specific modes of action as opposed to focusing solely on detecting inhibitors of TH synthesis or inducers of TH metabolism, which represent the majority of known thyroid disrupters.
8.5.1.3 Pre/Prometamorphic Exposure Windows
As noted earlier, premetamorphic postembryonic development in anuran larvae (e.g., from hatching until early hind limb bud stage, N&F 54 in X. laevis) is independent of TH control, although expression of TRα at low levels precedes this. As a consequence, exposure of premetamorphic larvae to exogenous TH can stimulate precocious metamorphic changes. These changes are well orchestrated and can result in a normal transition to the adult form when T4 is used, which accommodates tissue-specific control of conversion to the active T3 by MDIs. In contrast, precocious metamorphosis stimulated by T3 typically is abnormal and accompanied by high levels of mortality, owing in large part to the fact that the normal sequence of tissue transformations regulated by local availability of T3 though MDI activity is bypassed [93]. Nevertheless, the ability to respond to TH in premetamorphic larval development provides a useful window in which exogenous TR antagonists might be detected with higher sensitivity than in prometamorphosis, when the endocrine milieu of the larvae is increasingly dominated by endogenous TH.
In two chronic larval development studies in X. laevis, Goleman et al. [65,66] reported that exposure throughout larval development to ammonium perchlorate (a competitive inhibitor of the sodium/iodide symporter that inhibits TH synthesis) resulted in inhibition of hind limb growth, indicating the potential sensitivity of hind-limb length as an endpoint. However, these studies featured an exposure duration of 70 days, and while this may in part reflect suboptimal conditions for larval development [101], the approach of exposing larvae throughout larval development would not likely be amenable for use at a screening level, where there is an emphasis on throughput and low cost in order to deal with potentially large numbers of test chemicals.
Kloas’s group developed the XEnopus Metamorphosis Assay (XEMA), which features a 28-day exposure period starting from stages 48 to 50 (in premetamorphosis, going through into prometamorphosis) with assessment of the primary end points, body length and developmental stage, at days 7, 14, 21, and test termination [88]. This assay underwent interlaboratory validation activity in an informal ring test funded by the Umweltbundesamt of Germany. The exercise tested several chemicals: amitrole, zineb, and ethylenethiourea (ETU), with propylthiourea (PTU) as a control inhibitor of TH synthesis and T4 as a control TR agonist [88]. This study indicated that developmental stage is a robust and reliable end point for use in an AMA for thyroid-disrupting chemicals. As T4 had accelerated development by day 7, the study also demonstrated the potential advantages of an AMA in which exposure was initiated in premetamorphosis. However, at 28 days, and with quite labor-intensive staging of all larvae at days 7, 14, 21, and 28, this assay is still quite demanding and time consuming for a screening-level assay, which was the primary remit of the OECD Ad Hoc Expert Committee on Amphibian Testing [90].
Further work in developing the AMA investigated the potential for shortening the assay while retaining ability to detect both TR agonists (e.g., T3, T4) and inhibitors of TH synthesis. Tietge et al. [101] compared the response of Xenopus larvae to chronic exposure to ammonium perchlorate throughout larval development (44 d), and to eight- or 14-day exposure, starting at either stage 51 (premetamorphosis) or stage 54 (beginning of prometamorphosis). While the longer exposure period was more sensitive for the developmental stage end point than either of the short exposure windows, in both experiments histological changes in the thyroid gland were more sensitive than developmental stage distribution. The higher sensitivity to TPO inhibitors of thyroid gland histological changes, compared to developmental stage distribution, was corroborated in similar pre-/prometamorphic comparison studies conducted by Degitz et al. with methimazole and PTU. Moreover, the effects of exogenous T4, as a model TR agonist, were assessed in parallel studies with 21-day exposure starting at stage 51 and 14-day exposure starting at stage 54. Advancement of developmental stage distributions were seen in both exposure models, with comparable sensitivity [102].
Taken together, these studies at the EPA Duluth Laboratory provided several important findings:
- A short pre-/prometamorphic exposure window was sufficient to enable detection of TH synthesis inhibition.
- Extending the exposure window does not necessarily improve sensitivity to inhibitors—more time for development can result in greater variability within replicates/treatment groups and reduce statistical power.
- Thyroid gland histology was more sensitive than assessment of developmental stage.
- There may be qualitatively different responses of the thyroid gland to TH synthesis inhibitors with slightly different modes of action (e.g., PTU has some monodeiodinase inhibition activity in addition to TPO inhibition).
- Thyroid gland size increased in a proportionate manner with respect to exposure concentration (i.e., there was a linear concentration dependence of thyroid gland area, and this could be used as a quantitative end point reflecting changes in the thyroid gland).
8.5.1.4 AMA Test Guideline Development
Taking stock of the findings reviewed above relating to XEMA (four-week assay starting at stage 51) and shorter-duration studies developed by the EPA lab (8- to 21-d studies starting at stage 51/54), the OECD Ad Hoc Expert Group on Amphibian Testing oversaw a series of three interlaboratory validation exercises, with contributions from several countries and academic, industry, and government laboratories. These exercises provided valuable data on intra- and interlaboratory variability in test parameters while addressing issues raised in discussion around the duration of exposure and stage at initiation.
In particular, the Phase I exercise addressed the question of whether three weeks of exposure from stage 51, incorporating about one week of premetamorphic development in which endogenous TH levels would be low, would lend the assay greater sensitivity to agonists. The Phase I exercise therefore compared 21-day exposure starting at stage 51 with 14-day exposure starting at stage 54, and featured measurement of hind-limb length at day 7 in addition to the primary end points of developmental stage and thyroid gland histology. Across the three participating labs, significant differences in sensitivity to T4 (as a model TR agonist) or PTU (a model TH synthesis inhibitor) between the stage 51/21d and stage 54/14d approaches were not observed. In light of this equivocal data, for subsequent optimization and validation studies, the stage 51/21d approach was preferred as it still offered potentially greater sensitivity to TR agonists in the early premetamorphic phase (assessed at 7d) and also potentially greater sensitivity to inhibitors of TH synthesis, in providing a longer exposure window for exaggeration of differences rate of metamorphosis. In support of this approach, additional studies conducted on behalf of EPA as part of the protocol demonstration and optimization studies [103] indicated that responsiveness of the assay to hydrophobic chemicals (e.g., PCBs), taking longer to reach critical tissue burdens, would be improved with a longer exposure window.
Subsequent phases of the OECD optimization and validation process for the AMA included a five-laboratory ring test with three substances (T4, perchlorate, and iopanoic acid, an MDI inhibitor), and a final phase in which three labs tested two compounds: a non–thyroid-active EDC (E2) and benzophenone-2, a compound exhibiting weak thyroid-disrupting activity in rodent assays. This final phase also further optimized and standardized the feeding regime to better control background growth and development, as variation in these control parameters is a potential source of error in assessment of both morphological and histopathological end points.
The result of this development work is the OECD Test Guideline (TG) 231 for the AMA, in which several chemicals with known thyroid-disrupting activity have been reliably shown to exhibit effects on gross morphology of the tadpole (as evident from assessment of development stage or measurement of hind-limb length) and/or on thyroid histopathology. The TG involves aqueous exposure (ideally flow-through) of X. laevis larvae from N&F stage 51 for 21 days and features these endpoints:
- Mortality (as indication of systemic toxicity, observed daily through the test)
- Growth (wet weight and snout-vent length)
- Development (hind-limb length and developmental stage)
- Thyroid histopathology
After seven days of exposure (around the transition from premetamorphic to prometamorphic development), a subsample of larvae is randomly selected for measurement of hind-limb length, snout-vent length and wet weight, and development stage, in order to maximize detection of thyroid agonist activity of test chemicals.
In addition to guidance on conduct of the AMA, test acceptability criteria, and feeding schedule to minimize potential confounding effects of suboptimal growth during the test, TG 231 also provides a decision tree for interpretation of effects on the selection of apical end points used. This decision tree is reproduced in Figure 8.4. The potential outcomes of the test/decision tree, in relation to modes of action of thyroid disruption, are discussed in the following sections.
FIGURE 8.4 Decision logic for interpretation of the outcomes of an amphibian metamorphosis assay, as set out in OECD Test Guideline 231. *Histology may be required by some regula-tory authorities despite significant differences in advanced and asynchronous development.
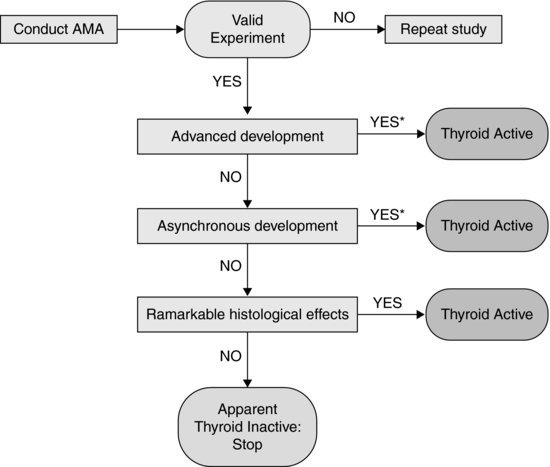
8.5.1.5 Advanced Development
Advanced development would be indicated by significant differences in hind-limb length (increased) or developmental stage (increased stage number) between test concentrations and controls. Advanced development would be indicative of some sort of thyroid activity, most likely TR agonist activity, although effects on central regulation of thyroid system could not be ruled out on evidence from apical end points used in the AMA. Theoretically, increased conversion of T4 to active T3 in target tissues could advance development, but given the variety of monodeiodinase activities and their differential expression in thyroid-responsive larval tissues (discussed earlier), it is far more likely that such an effect would be manifest in asynchronous development (see Section 8.5.1.6).
According to TG 231, some regulatory authorities may require confirmatory thyroid histopathology in addition to advanced development before concluding that a chemical was thyroid active, although it is worth noting that subsampling at day 7 is primarily to maximize detection of agonists (primarily through effects on hind-limb length), but histopathology is required only at day 21, test completion. Furthermore, care must be taken in comparing thyroid histopathology between T4-exposed larvae and controls when there are significant differences in stage of development between the two groups. Differences in thyroid gland structure in T4-exposed larvae whose development has been advanced may, of course, reflect the normal state of the gland at a more advanced developmental stage (albeit generated by exposure to the exogenous test chemical) rather than a strictly pathological response to the agent. In fact, whether such a distinction is meaningful for endogenous thyroid agonists is debatable, and there are few other known synthetic TR agonists with which to further test this issue (none of which has been tested in these assays with histopathological assessment of the thyroid gland to date).
During the OECD Phase II optimization and validation exercise, T4 was tested as a model thyroid agonist and exhibited equivocal effects on thyroid histopathology. Among the studies performed by with T4 at exposure concentrations between 0.5 and 2.0 mg/L, both thyroid gland atrophy and hypertrophy were observed, but there were no consistent concentration–response relationships with these histological end points in any of the Phase II studies with T4 [104]. While atrophy of the thyroid gland is typically indicative of excessive circulating thyroid hormones, glandular hypertrophy and changes in follicular cell morphology consistent with this (i.e., increased epithelial cell height, hyperplasia), which were generally observed at the higher exposure concentrations in the Phase II studies, run counter to what would normally be expected in responses to exposure to exogenous TH. However, this assumption is somewhat predicated by our understanding of better-chaaracterized mammalian models—the negative feedback inhibition of TH on the pituitary secretion of thyroid-stimulating hormone (TSH) is regarded as a universal feature of the vertebrate thyroid axis. When rats are exposed to TR agonists (i.e., TH), they typically exhibit morphological evidence of reduced stimulation of the thyroid gland [105], resulting from abrogation of the negative feedback inhibition on the pituitary thyrotropes—evident as reduced expression or secretion of TSH [106,107].
At this point, it is worth noting that while the basic components of the thyroid axis are evolutionarily conserved between amphibians and mammals, amphibian metamorphosis represents an exceptional model in which regulation of the thyroid axis may not be typical of feedback systems in adult vertebrates, such as rats or humans. In particular, the existence of negative feedback inhibition during pro-metamorphic development is hard to reconcile with the steady increase of circulating TH from the time the thyroid starts fixing iodide and synthesizing TH (around N&F stage 46) until it peaks in metamorphic climax. It has been suggested that the feedback effect of TH on pituitary secretion of TSH does not develop until metamorphic climax, when Type II MDI expression is up-regulated in the pituitary, enabling conversion of T4 to T3 [93]. However, as Manzon and Denver [108] point out, Huang et al. [93] did not directly test for the absence of negative feedback inhibition in prometamorphic larvae (i.e., before this up-regulation of Type II MDI).
Of course, the fact that exposure to chemicals that inhibit TH synthesis results in compensatory changes in the structure of the thyroid gland in Xenopus larvae (as demonstrated throughout the AMA development and validation process with various goitrogens) strongly suggests that there is indeed negative feedback inhibition during prometamorphic development, and this is consistent with similar findings reported in the literature [reviewed in 108]. In vitro studies with explant cultures of tadpole pituitaries indicate the potential for negative feedback inhibition by THs on pituitary thyrotropes from prometamorphic larvae, as exposure to TH at physiological concentrations results in down-regulation of expression of TSH β-subunit (TSH-β) and the α-glycoprotein subunit—the other half of TSH, which is shared with other pituitary glycoproteins [108]. Whether the simplified model of pituitary explant culture can be expected to fully represent regulatory interactions in the intact organism is uncertain. Indeed, Manzon and Denver [108] also suggest that the increase in thyroid titers through to metamorphic climax occurs in spite of a functional negative feedback mechanism at the level of the pituitary precisely because hypothalamic signals may override those signals. These overriding signals may be absent in the pituitary explant culture used by Manzon and Denver to demonstrate negative feedback mechanisms operating in the prometamorphic pituitary. Consistent with this, Opitz and Kloas [109] reported that after short-term in vivo exposure of prometamorphic Xenopus larvae to T4, pituitary expression of TSH-β was not significantly altered. They attribute this to the fact that negative feedback by T4 on the pituitary could be mediated in the first instance by inhibition of secretion of TSH by thyrotropes, which would be independent of transcription.
Given these uncertainties about the nature of feedback mechanisms in prometamorphic development in anurans and the equivocal findings regarding effects of T4 in the AMA optimization and validation studies, it is not clear whether thyroid histopathology is likely to provide as reliable and sensitive an end point for thyroid agonists as it does for TH synthesis inhibitors. In the absence of practical means by which to measure circulating (i.e., plasma or serum, not whole body) TH or TSH, which are of course readily available for the rat model, this potentially leaves only hind-limb length and developmental stage as end points for detection of thyroid agonists in this model.
Clearly there is a need for further research on the development and regulation of feedback mechanisms in the HPT axis in amphibian larvae, in particular during the prometamorphic developmental window that the AMA encompasses. Additionally, any such studies should specifically include thyroid histopathology to better support guidance on whether this end point will be valuable in confirming TH agonist activity in the AMA.
8.5.1.6 Asynchronous Development
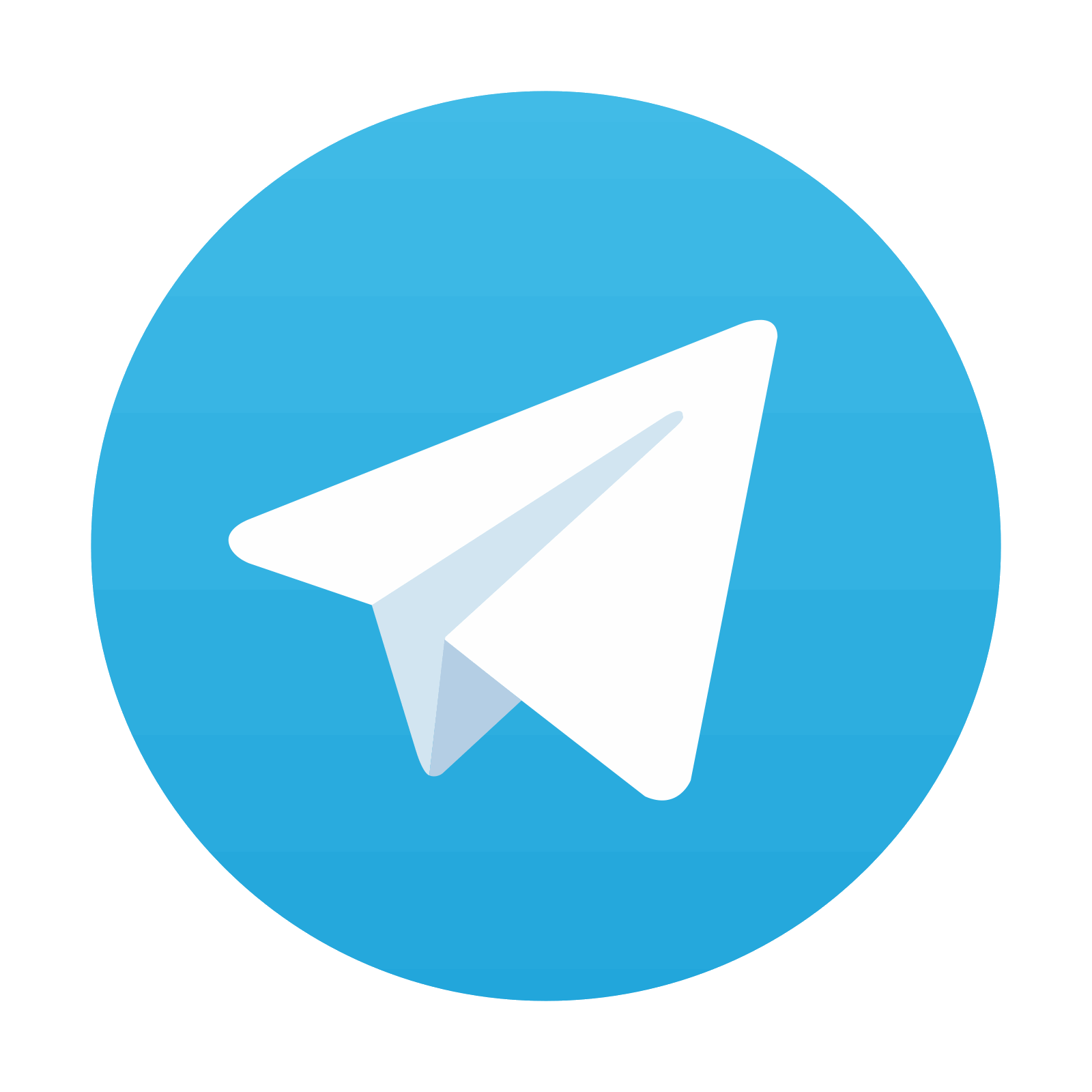
Stay updated, free articles. Join our Telegram channel
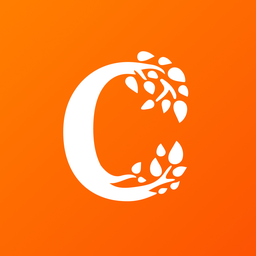
Full access? Get Clinical Tree
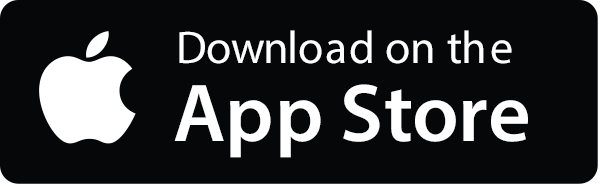
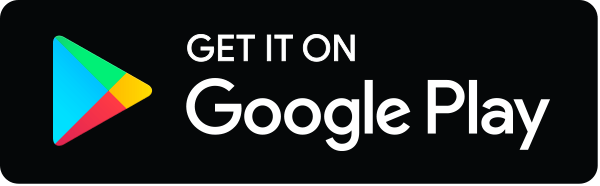