Fig. 1
Effects of GCs on body composition and metabolism . GCs promote whole-body insulin resistance via visceral adipogenesis, mobilization, and release of free fatty acids into the circulation and development of hepatic steatosis. In addition, hyperglycemia results from β-cell dysfunction, decreased insulin secretion, and increased gluconeogenesis. In skeletal muscle, GCs cause type II fiber atrophy and decreased glucose uptake. Bone loss occurs due to increased bone resorption followed by decreased formation from reduced osteoblast function and number. DAG, Diacylglycerol; PLC, phospholipase C; G6P, glucose-6 phosphatase; PPAR, peroxisome proliferator-activated receptor
GC Regulation of Adipose Tissue
Globally, the prevalence of obesity has reached epidemic proportions with over one billion adults being overweight, and of these, roughly 300 million being obese [7]. The rapid rise in obesity and its associated comorbidities pose a major public health concern and have made the study of obesity and its adverse metabolic profile increasingly important. Research in the past 20 years has led to an understanding that adipose tissue is a complex and highly active endocrine organ which contributes to the regulation of insulin action and with functions that are altered by obesity [8]. In addition, individuals who are obese have a higher all-cause mortality [9]. Those with GC overexposure have a mortality rate four times higher than the general population, primarily due to cardiovascular disease which is in part due to GC-induced obesity and insulin resistance [10]. In an effort to better understand the effects of GCs on adipose tissue, we will first discuss adipose tissue types with a focus on distribution and mass. This will be followed by a review of the common phenotypical changes seen in adipose tissue as a result of GC excess and the subsequent effects on glucose metabolism.
Adipose Tissue, Mass and Distribution
Adipose tissue is a complex, multicellular organ that influences the functions of other organ systems and includes numerous discrete anatomical depots with variable masses, ranging from 5 to 60 % of total body weight [11]. Subcutaneous adipose tissue is responsible for storing over 80 % of total body fat, with the most described depots being abdominal, gluteal, and femoral [12]. Visceral adipose tissue refers to adipose tissue surrounding the digestive organs and can be further divided into intra-abdominal or retroperitoneal depots. In men, visceral adipose tissue typically accounts for 10–20 % of total body fat, whereas in women it is about 5–10 % [12]. Smaller depots include epicardial and inter-muscular, which may have specialized functions related to their neighboring cells. Adipose tissue is composed of adipocytes as well as stromal vascular cells , which include preadipocytes, endothelial cells, pericytes, and immune cells (macrophages, T-cells, neutrophils, and lymphocytes) [12–14].
Further distinction within adipose tissue depends on cell structure, location, vascularization, and function [15]. Two types of adipose tissue, present in all mammals, have been identified, white and brown adipose tissue (WAT and BAT). Broadly, WAT and BAT are involved in opposing functions: energy storage in WAT and energy dissipation in BAT. BAT is present primarily in newborns and its functions include regulation of thermogenesis. However, recent studies have reported the presence of BAT in adults, in cervical-supraclavicular, perirenal, and paravertebral regions, but its role in body weight and metabolic function has not yet been elucidated and is not the focus of this review [12]. A discussion of the effects of GCs on adipose tissue distribution and function will be presented here. Of note, any reference to adipose tissue refers to white adipose tissue.
GC Effects on Adipose Tissue Distribution
Chronic, excessive GC exposure has been shown to increase body fat mass; these changes are clearly evident in patients with CS who experience profound increases in total and visceral adipose tissue [5, 16]. While some studies have reported that peripheral fat stores may be reduced [17, 18], using whole-body MRI, the gold standard for assessing body composition, Cushing’s disease (CD) patients had more trunk subcutaneous and visceral adipose tissue, but similar masses of total and limb subcutaneous adipose tissue, compared to healthy controls [5]. CD patients also had an increased ratio of visceral to total fat compared to healthy controls [3]. Normalization of cortisol concentrations in patients with CD resulted in a significant reduction in trunk, subcutaneous, and visceral adipose tissue [6]. Furthermore, the distribution of adipose tissue changed: visceral/total fat and visceral fat/skeletal muscle ratios decreased, further demonstrating the effects of GCs on adipose tissue distribution [6].
Mechanisms Underlying GC-Mediated Adiposity
The lipolytic effects of GCs have been well-established, yet excess GCs are associated with increased adiposity [19]. While most obese individuals do not show evidence of elevated morning serum GC levels, considerable evidence suggests that tissue GC levels may not adequately reflect plasma levels [20]. Although it is difficult to measure tissue-specific GCs, adipose tissue is thought to have levels 10–15 times that of circulating levels [21], possibly due to 11 beta hydroxysteroid deyhdrogenase type 1 (11BHSD1) activity , which converts inactive cortisone to active cortisol, and thus enhances GC action [22]. Not only has visceral fat accumulation been associated with upregulation of 11BHSD1 [22] and a higher density of glucocorticoid receptors (GR) [23, 24], but both 11BHSD1 and GR levels are higher in the visceral compared to subcutaneous adipose tissue depots, suggesting a greater susceptibility to GCs [23] and providing a plausible explanation for site-specific adiposity [25]. One implication of enhanced GC action may include increased lipoprotein lipase (LPL) activity in adipose tissue [26]. GCs are thought to increase LPL activity via transcriptional or posttranslational modifications [26–28]. Fried et al. demonstrated increased LPL activity in omental adipose tissue of obese men and women cultured in dexamethasone [28]. The increase in activity was largely explained by the ability of dexamethasone to increase LPL expression and allows for more fatty acids (FA) being available for storage in this depot [19].
GCs increase adipose tissue mass via hypertrophy and hyperplasia [29]. Hypertrophy results from fatty acid synthesis and storage within adipocytes, whereas hyperplasia results from differentiation of preadipocytes to mature adipocytes [29]. The latter has been shown to occur in the setting of cortisol and dexamethasone exposure [30]. Also, the presence of 11BHSD1 and the resulting increase in tissue GCs promotes the differentiation of human adipose stromal cells to mature adipocytes, further confirming the adipogenic effects of GCs [29]. Interestingly, if adipogenesis were exclusively responsible for increased adiposity, individuals with GC excess would have numerous small adipocytes, which is not the case; assessment of adipose morphology in patients with CS reveals enlarged, hypertrophic adipocytes [16]. In addition, expansion of the extracellular matrix and stromal vascular cells may be involved in the accumulation of adipose tissue in response to GCs [3]. Further study of the effects of chronic GC exposure on adipose tissue morphology in humans is needed.
GC Regulation Of Glucose Metabolism and Insulin Resistance in Adipose Tissue
Adipose tissue is a major site for metabolism of GCs . The functions of adipose tissue are crucial determinants of whole-body glucose and lipid homeostasis. The importance of this is emphasized by the adverse metabolic consequences of both adipose tissue excess and deficiency [31]. For example, obesity, particularly in the visceral compartment, is associated with insulin resistance, hyperglycemia, and dyslipidemia [32]. The role of GCs in regulating adipose tissue function is complex and depends on the species, concentration, specific adipose depot [33], and chronicity of GC exposure.
Human and animal studies have shown that GCs induce pre-adipocyte differentiation and whole-body lipolysis [34–37]. Corticosterone increased pre-adipocyte differentiation in 3T3-L1 cells with increased expression of adipose triglyceride lipase and hormone-sensitive lipase (HSL) [34]. HSL contributes to the hydrolysis of triglycerides (TG) in adipocytes. Similarly, lipolytic hormones increased when dexamethasone was added acutely to rat adipocytes [35]. After rats were treated for 10 days with corticosterone, free FA and glycerol levels were elevated in both fed and fasted states [34]. Thus, acute and subacute exposure to GCs increases lipolysis in vivo . Increased lipolysis results in elevated circulating free FA levels, which in turn is associated with insulin resistance [35]. Therefore, the diabetogenic effects of GCs are not only secondary to enhanced hepatic gluconeogenesis, impaired glucose uptake in muscle, and inhibition of insulin secretion, but also to elevated circulating free FA which originate from adipose tissue lipolysis [3].
Of note, it has been shown that GCs and insulin work synergistically to activate LPL, another lipolytic hormone [26]. Elevated LPL activity and intravascular lipolysis stimulate uptake of FA and glycerol into adipose tissue, leading to expansion of adipose tissue mass, as mentioned earlier [17]. This GC-dependent increase in LPL activity is thought to be due to increased transcription of LPL mRNA or posttranslational modifications such as inhibiting the degradation of newly synthesized LPL [26–28].
Chronic GC exposure leads to adipose tissue expansion which suggests enhanced total body lipogenesis despite a possible increase in lipolysis [3]. To our knowledge, only two small studies have examined lipolysis in the setting of chronic endogenous GC exposure caused by CS [16, 38]. When examined ex vivo, glycerol release was reduced in femoral and abdominal adipose tissue from women with active CS, suggesting decreased lipolysis [16]. Conversely, glycerol concentrations were elevated in in vivo subcutaneous adipose tissue from patients with CS consistent with increased lipolysis [38]. Therefore, GCs possibly regulate factors such as hormone or neuronal signals in tissues other than adipose, which indirectly control adipose tissue functionality and may override the direct effects of GCs on adipose tissue [3].
Exposure to GCs leads to whole-body insulin resistance; however, the individual action in each tissue may vary. In fact, studies have shown that dexamethasone enhances insulin signaling and activity in human adipose tissue [39–42]. Forty-eight-hour dexamethasone pre-treatment led to a dose- and time-dependent increase in insulin-stimulated protein kinase B/akt phosphorylation and insulin receptor substrate (IRS)-1 phosphorylation in human adipocytes, but the reverse effect in skeletal muscle. These effects were mediated through induction of insulin receptor (IR), IRS-1, IRS2, and the p85 regulatory subunit of phosphoinositide-3-3-kinase, which led to augmented insulin-mediated activation of akt [40, 41]. Subsequent investigation showed that both short-term (24 h) and longer-term (7 day) exposure of differentiated human adipocytes ex vivo to dexamethasone increased insulin signaling, consistent with increased sensitization, whereas chronic high-dose GC exposure led to insulin resistance [42]. This was consistent with an in vivo study which showed that overnight administration of hydrocortisone induced systemic insulin resistance, but enhanced insulin signaling and uptake in subcutaneous adipose tissue [43]. These studies imply that the effect of GCs on insulin action may be tissue-dependent—increasing insulin sensitization in subcutaneous adipose tissue while inducing insulin resistance in muscle.
Lastly, GC treatment was shown to inhibit 5′ adenosine monophosphate-activated protein kinase (AMPK) activity in rat visceral, but not subcutaneous adipose tissue [44]. AMPK is a key regulatory enzyme of lipid and carbohydrate metabolism as well as appetite. This observation is supported by data showing that, compared to control patients, patients with CS demonstrated a 70 % lower AMPK activity in visceral adipose tissue [45].
In conclusion, the long-term exposure to elevated GC levels results in adipose tissue accumulation and altered distribution. These body composition changes are associated with insulin resistance in part via increased lipolysis, enhanced systemic elevations in FA and TG, and impaired insulin signaling.
GC Regulation of Skeletal Muscle
GC Regulation of Skeletal Muscle Composition
After Dr. Harvey Cushing’s first description of muscle weakness in his case report of Minnie G in 1910 [46], it was not until Drs. Muller and Kugelberg’s 1959 case series of six patients with CS when GC-induced myopathy was further described [2, 47]. Since then, a few clinical studies examining muscle function, histology, and metabolism in patients with CS have provided some framework for understanding the effects of GCs on muscle [48, 49]. GC-induced myopathy typically presents as proximal weakness, with predominant involvement of the lower extremities, and is seen in 56–90 % of patients with CS [2, 50]. Patients with CD also have reduced skeletal muscle mass compared to weight-matched controls [5], and surprisingly, skeletal muscle mass may continue to decrease over time after surgical remission [6]. Effects of GCs on muscle may be related to dose, type of GC (when given exogenously), duration of exposure, and specific muscle fiber type [48, 49]. In order to better understand the role of GCs in muscle mass and function, a brief review of muscle fibers followed by mechanisms underlying GC-mediated myopathy will be discussed.
Muscle fibers are categorized into slow twitch oxidative (Type I), fast twitch oxidative (Type IIa), and fast twitch glycolytic fibers (Type IIb); additional fiber types (Ic, IIc, IIab, IIac) are based on myosin ATPase histochemical staining [51]. Type 1 fibers are characterized by high levels of slow isoform contractile proteins, mitochondria, myoglobin and capillary densities, and oxidative capacity. Type IIa fibers are defined as having a high oxidative capacity with fast contraction, whereas type IIb fibers are described by low volumes of mitochondria, high glycolytic enzyme activity, increased rate of contraction, and low fatigue resistance [2].
More than 30 years ago, investigators used myometers and strain gauge techniques to quantitatively assess proximal weakness in patients with GC-induced myopathy, in addition to needle biopsy of muscle and 24 h urinary 3-methylhistidine excretion [50]. Fiber atrophy, specifically of type II fibers , is the classic histological abnormality associated with GC-mediated myopathy; interestingly, this finding is also present in other endocrinopathies including thyrotoxicosis, myxedema, and osteomalacia [2, 52]. Patients with CS have reduced type II fiber mean area, myopathic changes (including increased polyphasic muscle potentials on EMG), and an elevated 24 h urinary 3-methlyhistidine/creatinine ratio (an assessment of myofibrillar protein breakdown) [50]. Other ultrastructural changes associated with CS myopathy include pronounced mitochondrial damage, thickening and deep invaginations of the sarcolemmal basement membrane, and thickening of the basement membrane capillaries [53]. Muscle fibers from CS patients also demonstrate marked disarray and wide interfibrillar spaces containing large vacuoles which represent degenerated mitochondria [53]. Interestingly, Khaleeli et al. noted that histological abnormalities were more pronounced in the group of patients exposed to exogenous GCs for the treatment of inflammatory conditions compared to patients with endogenous CS. This was thought to be secondary to the high cumulative exposure of GCs, but alternatively it was also suggested that induction of 11BHSD1, which is present in human skeletal muscle, might also be increased in inflammatory conditions, as has been demonstrated in adipose tissue and bone [54].
Mechanisms Underlying GC-Mediated Myopathy
GC excess is associated with a decreased rate of protein synthesis and an increased rate of whole body proteolysis, even in patients who receive GC treatment for a short duration [2]. Skeletal muscle atrophy is a well-described adverse consequence of excess GC exposure [5]. Age is thought to impact the severity and mechanism of these catabolic effects; studies in rats have shown that GCs caused more severe atrophy in older compared to younger rats [55].
The inhibitory effects of GCs on protein synthesis are multifactorial. First, GCs inhibit the transportation of amino acids into the muscle [56]. Second, GCs inhibit the stimulatory action of insulin, insulin like growth factor-I (IGF-1), and amino acids (specifically leucine) on the phosphorylation of eIF4E binding protein (4E-BP1) and the ribosomal protein S6 kinase 1 (S6K1), two factors that are instrumental in the initiation of translation of mRNA responsible for the protein synthesis machinery [57]. Finally, GCs may inhibit myogenesis by down-regulating myogenin, a transcription factor mandatory for the differentiation of satellite cells to muscle fibers [58].
The stimulatory effect of GCs on muscle proteolysis is a result of the activation of major cellular proteolytic systems , specifically the ubiquitin-proteasome system (UPS), the lysosomal system (cathespins), and the calcium-dependent system (calpains) [59]. Thus, there is enhanced degradation of myofibrilliary fibers, which is evident by increased 3-methlyhistidine excretion. GCs activate protein degradation by stimulating the expression of several components of the UPS, which are either directly involved in protein degradation by a proteasome or by conjugation of protein to ubiquitin marking it for degradation [55].
Other factors that have been implicated in the development of GC-mediated myopathy include altered production of growth factors that locally control muscle development, specifically IGF-1. GCs inhibit IGF-1 production in muscle [60]. IGF-1 stimulates muscle mass by increasing protein synthesis and myogenesis while decreasing proteolysis and apoptosis [61, 62], linking decreased IGF-1 expression to GC-induced muscle atrophy [55]. Recent studies have shown that IGF-1 down-regulates the lysosomal, proteosomal, and calpain-dependent proteolytic systems [63–65], suppresses muscle cell atrophy caused by GCs [66], and interestingly prevents GC-induced muscle atrophy as evidenced by systemic administration or local overexpression of IGF-1 in rat skeletal muscle [67].
GCs also stimulate the production of myostatin (Mstn) , a member of the transforming growth factor-beta family, and a potent inhibitor of muscle growth which down-regulates the proliferation and differentiation of satellite cells and protein synthesis [68]. In vitro data show that Mstn contributes to muscle cell atrophy by reversing the IGF-1/PI3K/Akt hypertrophy pathway; this finding was further solidified by a murine model that revealed that targeted disruption of Mstn gene expression in mice led to significant increases in skeletal muscle mass due to fiber hyperplasia and/or hypertrophy [55, 69, 70]. Interestingly, in humans, loss of function mutations of Mstn cause muscle hypertrophy, a rare condition characterized by reduced body fat and increased muscle size [71]. Furthermore, transgenic mice over-expressing Mstn in skeletal muscle have muscle atrophy [72, 73], and rats that were treated with dexamethasone in an effort to induce muscle atrophy were found to have significantly increased levels of Mstn mRNA expression and protein concentrations [74]. Further, in contrast to wild-type mice, Mstn knockout mice did not develop reduced muscle mass or fiber cross-sectional area after treatment with GCs [75]. Thus, increased muscle Mstn has been implicated as a key player in GC-induced muscle atrophy.
GC Regulation of Glucose Metabolism and Insulin Resistance in Skeletal Muscle
Skeletal muscle is the largest source of glycogen storage in the human body and accounts for 80 % of insulin-mediated, postprandial glucose uptake [76, 77]. GCs inhibit glucose uptake and utilization largely through antagonizing the actions of insulin in skeletal muscle. GCs also alter lipid and protein metabolism within skeletal muscle which leads to reduced insulin sensitivity [78–80].
One of the mechanisms by which GCs impede glucose uptake is by inhibiting insulin-stimulated translocation of the glucose transporter GLUT 4 to the plasma membrane, as demonstrated in mice treated with dexamethasone [81, 82]. GCs have also been shown to interfere with the insulin signaling cascade in skeletal muscle both in vitro and in vivo [83–86]. Insulin binds to the cell-surface IR, a tyrosine kinase that autophosphorylates and phosphorylates the IRS. Tyrosine-phosphorylated IRS associates with IR and activates downstream signaling [87]. Dexamethasone-treated mice have decreased expression and activity of tyrosine phosphorylated IR and IRS-1 [83]. The activity of phosphatidylinositol 3-kinase (PI3-K) and protein kinase B (PKB)/Akt, key signaling molecules that act downstream, is also reduced after GC exposure [83, 84, 86, 88]. Furthermore, GCs decrease glycogen synthesis and promote insulin resistance by suppressing glycogen synthase-3 phosphorylation [88]. A randomized cross-over study to determine the effect of 6 days of prednisone in 7 young healthy volunteers showed that although insulin infusion increased glucose uptake in both groups, uptake was 65 % lower in the prednisone-treated group versus the placebo group [89].
GCs also reduce insulin sensitivity, and subsequently glucose uptake, in skeletal muscle through effects on lipid metabolism . Elevated GCs stimulate adipose tissue lipolysis, which results in increased circulating levels of FA and TG [90, 91]. This enhances the accumulation of intramyocellular lipids (droplets of TG in skeletal muscle fibers) such as fatty acyl CoA and diacylglycerol (DAG), which are strongly correlated with reduced glucose uptake and insulin resistance [92, 93]. It has been shown, using magnetic resonance spectroscopy, that intramyocellular lipids decrease insulin signaling by activation of a serine/threonine kinase cascade involving protein kinase C, IKK-B and c-Jun amino-terminal kinases (JNKs). Phosphorylation of these serine sites leads to formation of proteins that are unable to activate PI3-K, which results in decreased glucose transport as discussed earlier [80].
Inter-muscular adipose tissue is another recently recognized ectopic adipose depot that is located beneath the muscle fascia but between the muscle groups (i.e. fat “marbling” within the muscle). It has been associated with development of insulin resistance [94], but was not found to be different in patients with CD vs. weight matched controls as measured by whole-body MRI [5].
As discussed above, enhanced protein breakdown and consequently elevated circulating amino acids (AA) have been reported after short-term high-dose GC treatment [95]. Elevated AA can impede insulin signaling by inhibiting insulin-stimulated IRS phosphorylation and activation of P13-K in cultured hepatoma cells and myocytes [96], leading to reduced glucose uptake and glycogen synthesis [78, 79]. Hence, the combined effects of reduced total muscle area and increased circulating AA lead to decreased insulin-mediated glucose uptake after prolonged GC exposure.
GC Effects on Liver
Excess GC exposure can increase glucose production and promote insulin resistance through regulation of carbohydrate and lipid metabolism in the liver. Several mechanisms have implicated GCs in the stimulation of hepatic lipogenesis and insulin resistance both directly and indirectly. Similar to skeletal muscle, excess GCs disrupt the insulin signaling cascade in hepatic tissue [84, 97, 98]. Dexamethasone-treated rats have reduced IR binding in hepatocytes [97] and down-regulation of the IR [98]. Additionally, livers of rats treated with dexamethasone exhibit decreased tyrosine phosphorylation of the IR and IRS-1 [84].
GCs also increase endogenous glucose production (EGP) by the liver [99, 100]. In the basal state, this is driven by increased gluconeogenesis via various mechanisms. First, GCs induce rate-limiting enzymes for gluconeogenesis such as phosphoenolpyruvate carboxykinase (PEPCK) and glucose-6-phosphatase (G6P) [99, 100]. PEPCK is required to generate glucose-6-phosphate, whereas G6P cleaves the phosphate allowing for glucose release into the circulation. The PEPCK gene contains GC response elements in its promoter region and plays a crucial role in GC-induced hyperglycemia. Of note, GC-mediated expression of gluconeogenic enzymes, such as PEPCK, is dependent on the cholesterol-sensing liver X receptors (LXRa and LXRb), which influence the recruitment of GR to gluconeogenic promoters. Mice lacking LXRb, but not LXRa, were resistant to dexamethasone-induced hyperglycemia, hyperinsulinemia, and hepatic steatosis [101]. Second, since GCs promote muscle wasting, lipolysis, and breakdown of protein and fat stores, the availability of substrates, such as alanine and glycerol, is increased, for gluconeogenesis in the liver [102–104]. Third, hepatic activation of the nuclear receptor peroxisome proliferator-activated receptor (PPAR-α) is associated with GC-induced hepatic insulin resistance and hyperglycemia. One study showed that PPAR-α knockout mice treated with dexamethasone did not develop hyperglycemia or hyperinsulinemia, concluding that PPAR-α expression is necessary for GC-induced increases in EGP [105]. Other mechanisms for enhanced EGP include increased metabolite transport across the mitochondrial membrane and potentiation of the effects of other gluco-regulatory hormones such as glucagon and epinephrine [103]. GCs also affect hepatic glucose metabolism by directly antagonizing the actions of insulin. For example, ceramides which are lipid-derived signaling molecules mediate GC-induced hepatic insulin resistance by blocking Akt phosphorylation and activation [106].
In addition to altering hepatic carbohydrate metabolism, GCs play an important role in hepatic lipid metabolism. Intrahepatic lipids are associated with insulin resistance and obesity and represent an important marker of cardiovascular risk, potentially even more so than visceral fat [107]. GC treatment leads to accumulation of intrahepatic lipids through various mechanisms including lipolysis of visceral adipose tissue, which leads to increased TG synthesis and delivery of free FA to the liver [108]. This leads to systemic hyperinsulinemia and hyperglycemia which drives de novo hepatic lipogenesis [109]. The critical role of GCs in hepatic lipid metabolism is demonstrated by improvement in hepatic steatosis and normalization of hepatic TG concentration in a fatty liver mouse model after liver-specific disruption of GR action [110].
GCs also enhance insulin-stimulated hepatic lipogenesis through upregulation of acetyl-CoA carboxylase and fatty acid synthase and increased very low-density lipoprotein (VLDL) production , resulting in increased TG levels, via inhibition of hepatic lipolysis [3, 111]. One small study reported enhanced VLDL secretion by the liver in patients with CD, which normalized after reduction of cortisol levels [91], and increased VLDL in healthy patients treated with prednisone [112], although these results have not been replicated.
Clinical data implicating GCs in the pathogenesis of hepatic steatosis are limited. Obese patients with nonalcoholic hepatic steatosis, measured via ultrasonography, had higher post-dexamethasone-suppressed cortisol values and insulin resistance compared to patients without steatosis [113]. Additionally, altered cortisol metabolism has been reported in patients with hepatic steatosis [32, 114], which suggests a relationship between hepatic fat and altered cortisol sensitivity and regulation in the general population [3]. Although hepatic steatosis is a known sequelae of prolonged GC exposure, only one study has investigated this in CD patients and reported a prevalence of 20 % [115]. The prevalence of hepatic steatosis in the asymptomatic general population varies widely, with results ranging from 2.8 to 24 % [116–119], and as high as 33.6 % in one study [120]. A few case reports have additionally linked the effect of excess GCs to fatty liver [121, 122]. As previously noted, H-magnetic resonance spectroscopy, the gold standard for determining hepatic lipid content, has never been investigated in humans exposed to excess GCs [3]. Therefore, although data suggest a link between chronic GC exposure and development and progression of hepatic steatosis, this topic warrants further investigation in clinical studies.
GC Regulation of the Pancreas/β-Cell
The pancreas plays a vital role in glucose metabolism and is the major sensor of circulating glucose. β-cells respond to increasing plasma glucose by secreting insulin in order to maintain euglycemia. The effects of GCs on β-cell function and insulin secretion are complex and depend on the duration, dosage, and type of GC exposure.
Glucose uptake and its oxidation in β-cell mitochondria lead to a cascade of events including elevated adenosine triphosphate (ATP)/adenosine monosphosphate (ADP) ratio, influx of calcium, and activation of signaling pathways including protein kinase A (PKA) and protein kinase C (PKC) which stimulate insulin secretion [123]. GCs impair β-cell glucose metabolism by reducing the levels of GLUT2 and glucokinase (GK), therefore reducing glucose uptake and phosphorylation and downstream events [124, 125]. GCs also amplify glucose cycling by enhancing G6P activity [103, 126].
In vitro studies have shown that corticosterone inhibits the release of insulin in rodent islets following acute (within minutes) exposure [127, 128]. On the other hand, this rapid inhibitory effect is not seen in vitro with dexamethasone, a synthetic GC [129]. Only after a three-hour incubation period, isolated rat islet cells demonstrated up to 75 % reduced glucose-induced insulin secretion. These events were mediated through impaired activation of the DAG-phospholipase C (PLC)/protein kinase C signaling system. Additionally, dexamethasone reduced cyclic adenosine monophosphate (cAMP) levels, leading to reduced PKA activity and hence reduced insulin secretion [129]. GCs have also been shown to inhibit insulin secretion via upregulation of voltage gated K+ channel activity, thereby leading to decreased calcium transport [130, 131].
In humans, GCs may also inhibit insulin secretion after acute exposure. A single dose of prednisolone administered to healthy subjects resulted in reduced insulin secretion during a meal with reduced insulinogenic index (ratio between change in insulinemia and change in glycemia) [132]. Another study showed that one dose of dexamethasone administered during an oral glucose tolerance test caused impaired glucose clearance, but had no effect on insulin sensitivity [133]. It should be noted, however, that this acute inhibitory effect has not always been noted, with another study showing a rise in circulating insulin after acute administration of intravenous hydrocortisone [134].
Both in vitro and in vivo experiments suggest that this rapid inhibitory effect on insulin secretion may be due to increased sympathetic drive via activation of α-adrenergic signaling [135, 136]. For example, when hydrocortisone was administered to Swiss-Webster mice, the glucose-stimulated insulin levels were suppressed in both fed and fasted mice compared to mice not given hydrocorticone [135]. However, if the mice were given chlorisondamine or phentolamine (non-selective α-adrenergic antagonists) prior, this resulted in higher insulin levels in response to the hydrocortisone-induced hyperglycemia [135].
Longer exposure (2–15 days) to dexamethasone or prednisolone in healthy subjects can lead to hyperinsulinemia with increased C-peptide and decreased insulin sensitivity [132, 133, 137]. In these studies, healthy subjects were able to compensate for the GC-induced insulin resistance, resulting in euglycemia or only modest increases in fasting hyperglycemia. Other studies have shown that this hyperinsulinemic state after prolonged GC treatment is mediated by augmented β-cell function and mass, which counteracts the insulin resistance caused by GCs [138, 139]. However, normoglycemic subjects with reduced insulin sensitivity, first degree relatives of patient with Type 2 diabetes mellitus, obese, and other susceptible subjects may not be able to compensate [140–142]. In these settings, β-cell function does not correspond to the insulin demand and the imbalance of glucose homeostasis is more pronounced, resulting in hyperglycemia. These studies reinforce the concept that individual background is a critical factor when predicting the effects of GC exposure.
GC Regulation of Bone
GCs have a significant effect on bone physiology, and long-term exposure of the skeleton to GCs can result in osteoporosis and increased risk for fractures [2, 143]. Thus, the prevalence of osteoporosis in patients with CS is very high: approximately 55 % of women with CS have osteoporosis [144], and 19–50 % develop fractures, most commonly vertebral and rib fractures [2, 145–147]. Although bone mineral density may be decreased throughout the skeleton in CS patients, bone loss is most significant in areas rich in trabecular bone [146, 147]. First, the bone remodeling process and key cells will be briefly discussed, followed by a review of the mechanisms of bone loss secondary to GCs.
Bone Remodeling
Bone is dynamic tissue that is constantly undergoing catabolism (bone resorption) and anabolism (bone formation). Bone remodeling is the coupled process of bone breakdown followed by new bone formation ; it occurs in bone multicellular units (BMU) consisting of osteoclasts, osteoblasts, and surrounding tissue, and is regulated by biochemical and mechanical factors [148, 149]. Bone remodeling involves three consecutive phases: resorption, reversal, and formation. Resorption begins with the migration of mononuclear preosteoclasts to the surface of bone. Then under the influence of cytokines, hormones, physical, and chemical stimuli, preosteoclasts mature into osteoclasts, which are multinucleated cells which are able to decalcify bone by creating resorption pits [148, 149]. After osteoclastic resorption is complete, mononuclear cells appear on the bone surface in preparation for bone formation and to provide the necessary signals for osteoblast differentiation and migration. The formation phase consists of osteoblasts which cover the resorbed bone with osteoid, a compound that becomes bone once calcified. These phases vary in length of time, with resorption lasting about two weeks, reversal continuing up to 5 weeks, and a timeframe up to 4 months for the completion of formation [148]. Typically, bone formation and resorption occur in concert, but in conditions where bone resorption predominates or bone formation is compromised osteoporosis occurs [143].
Osteoblasts are specialized bone-forming cells with several important roles in bone remodeling which include expression of osteoclastogenic factors, production of bone matrix proteins, and bone mineralization [150]. Osteoclast maturation or osteoclastogenesis is regulated by various stimuli; one in particular is receptor activator of nuclear factor KB ligand (RANKL). RANKL is a transmembrane glycoprotein expressed on the surface of osteoblasts/stromal cells in the bone, and its expression leads to increased osteoclast maturation and activity, as well as suppressed apoptosis [149]. Interestingly, knockout mice lacking RANKL completely lack osteoclasts and the ability to resorb bone [151]. Additional factors stimulating osteoclastogenesis include sustained hyperparathyroidism, decreased sex steroids, and an increase in inflammatory cytokines [149]. Balancing the effects of RANKL , osteoblasts secrete a decoy receptor, osteoprotegerin (OPG), or osteoclast inhibitory factor (OCIF), which binds to RANKL preventing its interaction with RANK, subsequently leading to decreased osteoclast maturation and survival.
Mechanisms Underlying GC-Induced Osteoporosis
It is well-established that excess GCs reduce bone formation [152–164], which is the predominant mechanism of GC-induced osteoporosis, whereas studies on the effects on bone resorption have been conflicting [153–155, 157–160, 164]. One reason for these contradictory results is that many studies included patients with GC excess secondary to exogenous GC treatment for various disorders that impact bone turnover and mass independently [152, 159, 165–171]. Also, previous studies have included both eugonadal and hypogonadal patients, thus introducing another confounding factor in GC regulation of bone turnover and mass [172, 173]. Lastly, bone resorption has been studied with nonspecific markers, such as urinary hydroxyproline and serum type I cross-linked C telopeptide [155, 157, 160, 162, 163, 172, 174]. Despite these limitations, GCs do appear to increase resorption in concert with limiting formation, as evidenced by a study of 18 eugonadal female CS patients who were compared to eugonadal healthy controls . This study demonstrated decreased osteoblastic function, increased bone resorption, and reduced bone mineral density (BMD) at the forearm, femur, and spine in CS patients versus healthy controls [164].
An increase in bone resorption is likely responsible for the initial bone loss observed following GC exposure [143]. Previous studies proposed that this was caused by secondary hyperparathyroidism [152, 154–157, 159, 175–178]. GCs are known to decrease calcium absorption in the gastrointestinal system and increase urinary excretion of calcium, resulting in elevated PTH levels [156]. Chiodini et al. identified secondary hyperparathyroidism , indicated by high PTH levels in the presence of normal plasma calcium levels, in a series of eugonadal CS patients, but noted no correlation between bone resorption markers and PTH levels [164]. The specific finding of trabecular bone loss in the setting of hyperparathyroidism, an entity known to typically affect cortical bone [179], further points to direct GC effects as the central cause of bone loss in patients with CS, and not secondary hyperparathyroidism [164]. Furthermore, patients exposed to GCs develop bone disease essentially characterized by decreased bone remodeling, whereas this is increased in patients with hyperparathyroidism [143].
Another mechanism contributing to increased bone resorption in patients with CS is decreased gonadotropin production . In estrogen deficiency, T-cell tumor necrosis factor (TNF-α) increases, stimulating bone resorption [156]. However, it is unclear whether TNF-α is elevated specifically in GC-induced hypogonadism [180]. As a final point, GC-induced bone resorption may involve RANK-L and OPG [143, 181, 182]; GCs increase RANK-L, while decreasing OPG expression, resulting in enhanced osteoclastogenesis and bone resorption [143]. The abovementioned factors are thought to contribute to the initial bone loss seen in GC-induced osteoporosis. Eventually, bone remodeling will be decreased because of the inhibitory effects of GCs on osteoblastogenesis resulting in reduced osteoblasts number and function, which subsequently leads to reduced signals for osteoclastogenesis and increased osteoclast apoptosis [143, 183].
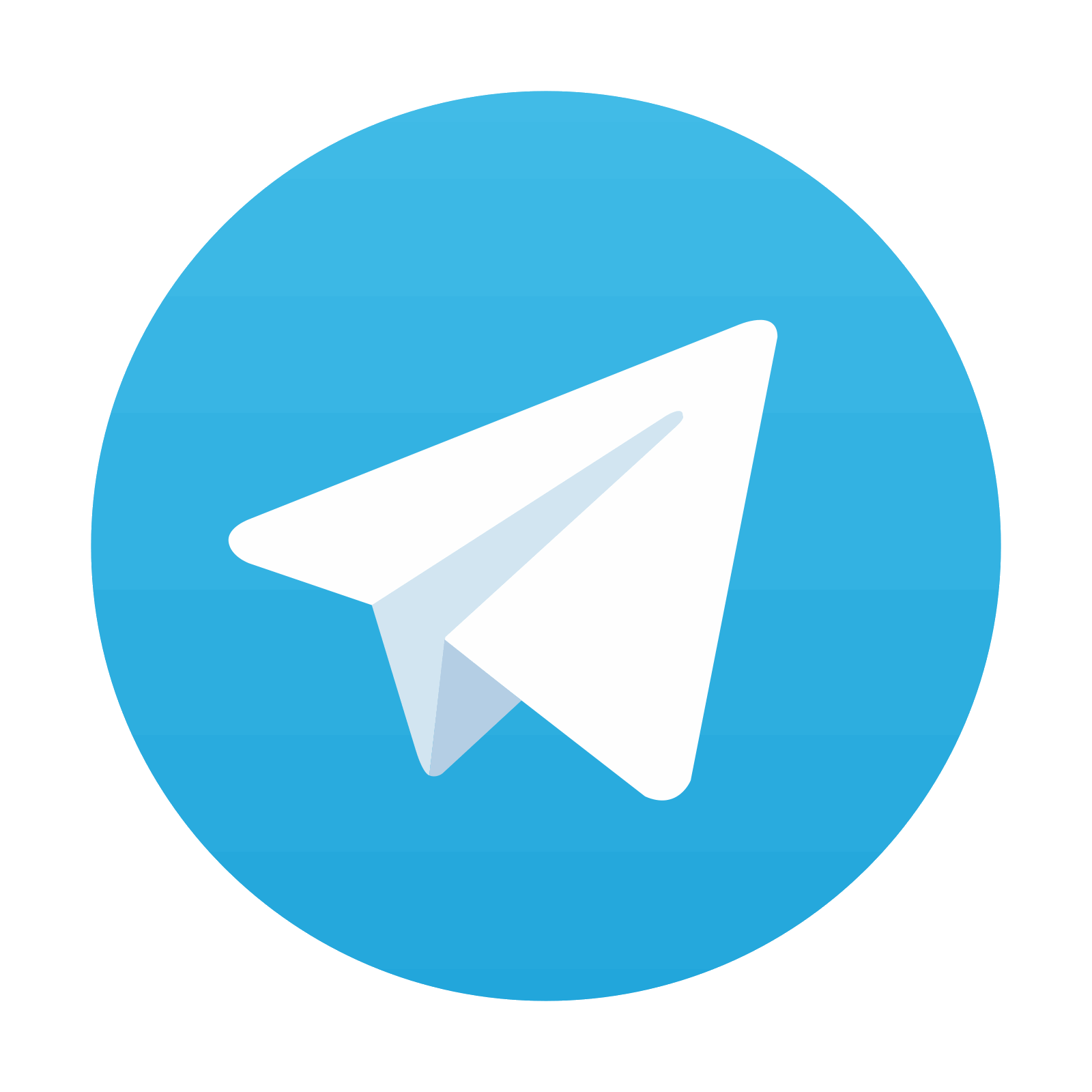
Stay updated, free articles. Join our Telegram channel
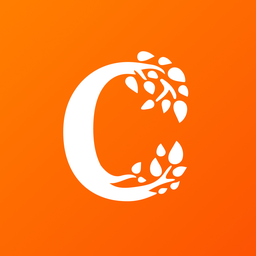
Full access? Get Clinical Tree
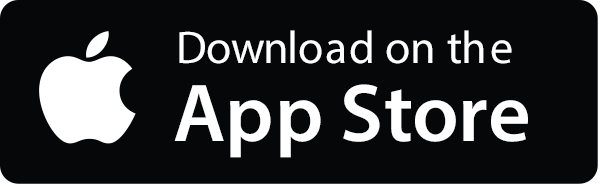
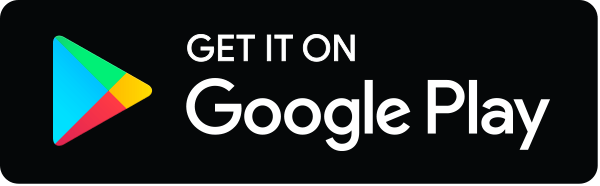