Puberty is the stage of development during which secondary sexual characteristics appear and there is a transition from the sexually immature to the sexually mature stage. Adolescence is widely used as a generally synonymous term for puberty, but it is often used to convey an added cultural connotation as a psychosocial coming of age.
By the mid-1960s a general concept of the major factors involved in the initiation of puberty was established ( Fig. 16.1 ). A decrease in sensitivity of the brain “gonadostat” to sex hormone negative feedback was thought to be the primary event. This signaled the hypothalamus to discharge neurohumors (then unidentified), which in turn stimulated the pituitary to release gonadotropins. The resultant rise in secretion of the gonadotropins, luteinizing hormone (LH) and follicle-stimulating hormone (FSH), was thought to account directly for increased estrogen production by the ovary. A mature relationship was thought to develop in which the blood levels of estrogen and gonadotropins were regulated reciprocally via the gonadostat, much as a furnace is regulated by a thermostat. The pineal was identified as having gonadal suppressive properties. The increased adrenocortical secretion of 17-ketosteroids (17-KS), which becomes apparent at about the time of puberty (“adrenarche”), was thought to be caused by a pituitary factor stimulating adrenal androgens in synergism with adrenocorticotropic hormone (ACTH).
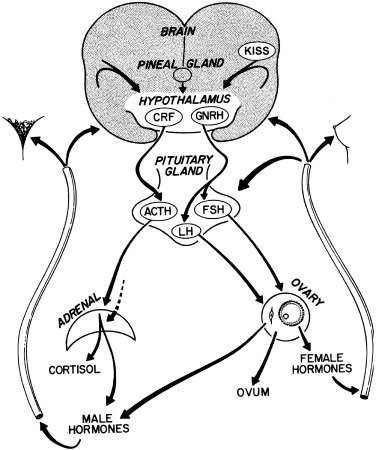
The rapid scientific advances since 1965 have permitted this concept to be tested in increasingly sophisticated ways. In the subsequent decade, radioimmunoassay (RIA), originally developed by Yalow and Berson, was applied to the measurement of gonadotropins and sex steroids; the gonadotropin-releasing hormone (GnRH) for both LH and FSH was isolated, identified, and synthesized by Guillemin’s and Schally’s groups. Cyclic adenosine-3′,5′-monophosphate (cAMP), postulated by Sutherland to mediate the action of peptide hormones, was found to mediate gonadotropin effects on the ovarian follicle. The initial steps in the mechanism of action of steroid hormones were defined by Jensen, Gorski, and their groups. The landmark nature of many of these discoveries was recognized by the awarding of Nobel Prizes in Medicine to Sutherland in 1971 and to Yalow, Schally, and Guillemin in 1977.
Our present view of the mechanisms controlling puberty is more refined and complex than it once was, although the earlier schema is correct in a general sense. The gonadostat is a patently oversimplistic concept for a complex system that regulates the activity of the hypothalamic GnRH pulse generator, a functionally interconnected and synchronized network of GnRH neurons. The gonadostat setting seems to change throughout childhood in a biphasic manner. This concept is illustrated in Fig. 16.2 . During most of fetal and perinatal life, the gonadostat is insensitive to negative feedback by sex steroid hormones; at this time the nascent neuroendocrine-gonadal axis functions at a pubertal level. The gonadostat becomes increasingly sensitive to negative feedback during infancy but does not become highly sensitive until midchildhood, at which time GnRH pulse generator activity is minimal. During late prepuberty, the gonadostat begins to relinquish its inhibition. This permits the onset of puberty. The changing set-point initially permits increasing, episodic secretion of GnRH. Increasing sensitivity of the pituitary gonadotropic cells to GnRH follows. The change in LH and FSH secretion is first detectable during sleep. Gradually, the gonads become increasingly sensitized to gonadotropin stimulation, grow at an increased rate, and bring about sustained rises in plasma sex steroid hormone levels. Some of these phenomena synergize with others, so that autoamplification occurs and the pace of change accelerates. Eventually, the set-point for gonadotropin release comes to vary sufficiently to encompass a positive feedback mechanism.
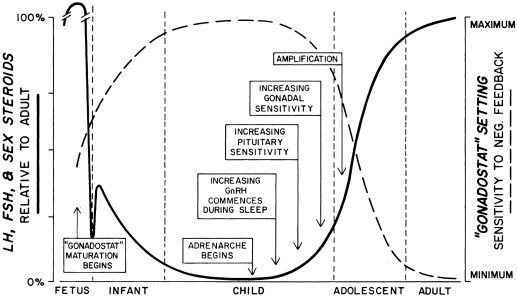
The data on which this model is based are presented later. The most recent data on the hormonal milieu and accompanying physical stages of normal puberty are then presented. Abnormal puberty is subsequently discussed: the causes, differential diagnosis, and management.
Development of the female reproductive system
Maturation of the Neuroendocrine-Ovarian Axis
Fetus
Neuroendocrine Unit
The anterior lobe of the pituitary gland, of stromal ectodermal origin, and the posterior lobe, of neural origin, differentiate by 11 weeks’ gestational age. By this time, GnRH neurons have migrated from the olfactory placode into place in the medial basal hypothalamus. Hypothalamic GnRH subsequently rises in parallel with fetal pituitary and serum LH and FSH. All peak at about 20 to 24 weeks, as the connections of the pituitary portal system become complete, to levels not again seen until menopause.
Serum LH and FSH levels are higher in human female than male fetuses. In rats, GnRH-containing neurons develop earlier in females than in males, and there are sexual dimorphisms in the degree of synapsing of specific tracts with dendritic spines in the preoptic nucleus, one of the major GnRH-containing areas of the hypothalamus. These differences may be determined by gonadal sex steroid hormone output. In all species studied, fetal secretion of LH, particularly LH pulse frequency, is permanently desensitized to estradiol-progesterone negative feedback by fetal virilization. In the rat, this has been demonstrated to be mediated by permanent impairment of estradiol-induced progesterone receptor (PR) gene expression.
In late gestation, fetal hypothalamic GnRH and pituitary gonadotropin secretion fall to low levels. These changes are likely explicable by the negative feedback effect of the high sex steroids produced by the fetoplacental unit. Meanwhile, maturation of the central nervous system (CNS) tracts that inhibit hypothalamic GnRH secretion and mediate gonadal negative feedback signals appears to progress throughout gestation.
The production of gonadotropins by the fetal pituitary seems to facilitate normal ovarian development. Hypophysectomy of rhesus fetuses has been reported to reduce the number of germ cells and oocytes, as well as the integrity of the rete ovarii. Therefore it seems that survival of gametes depends upon the secretions of the fetal pituitary.
Ovary
The ovaries differentiate in the urogenital ridge adjacent to the anlage of the adrenal cortex and the kidney. The granulosa cells are the homologues of the Sertoli cells of the testes. The theca, interstitial, and hilus cells are the homologues of the Leydig cells; hilus cells may even contain crystalloids like Leydig cells. Adrenocortical rests occasionally have been found in the hilus of the ovary. Conversely, ovarian rests have been identified in the adrenal glands.
The primitive germ cells migrate into the ovary from the yolk sac endoderm during the first month of gestation. The testes become histologically discernable by 8 weeks’ gestation. The ovaries develop in the absence of testicular development being switched on by the signaling cascade initiated by the SRY gene on the Y chromosome. Activation of the β-catenin signaling pathway by Wnt-4 and R-spondin1 permit forkhead ( Fox ) L2 transcription factor expression by germ cells to activate ovarian differentiation by sustaining oocyte and granulosa cell development and suppressing Sertoli and Leydig cell differentiation; they also support later aspects of follicle development. Steroidogenic factor-1 ( SF-1 ) WT-1 , LIM-1 , and possibly DAX-1 genes play roles in the formation of the ovaries. Germ cell bone morphogenetic proteins (BMPs) are necessary for primordial germ cell proliferation.
Primitive germ cells undergo mitotic division to become oogonia, a process that is maximum at 8 to 12 weeks. Oogonia then undergo oogenesis, entering the prophase of meiosis to become primary oocytes beginning at 12 to 16 weeks. The number of oocytes reaches a peak at 20 weeks when there are 6.8 million germ cells, of which 80% appear to be viable ( Fig. 16.3 ). When oocytes enter the diplotene stage of meiotic prophase they must be furnished with granulosa cells to form a primordial follicle, or else they undergo atresia.
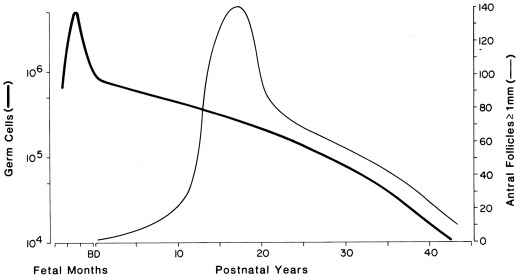
The ovary remains histologically undifferentiated until primordial follicles appear at about 16 weeks, when the epithelium of the secondary sex cords provides granulosa cells to the oocytes. However, the fetal ovary has the capacity for androgen and estrogen formation and signaling, although at a far lower level than the testes, by 12 weeks. Primordial follicles become primary follicles when the encircling granulosa cell layer becomes cuboidal. Primordial and small primary follicles ( Fig. 16.4 ) are resting follicles, which are the major repository of germ cells. This stock of germ cells is depleted only very slowly during childhood (see Fig. 16.3 ). Residence of primordial follicles in the ovarian cortex restrains their progression partly because of cortex mechanical rigidity. Mechanical effects are mediated by the growth-restrictive Hippopotamus signaling pathway and by vascular permeability via the vascular endothelial growth factor signaling pathway.
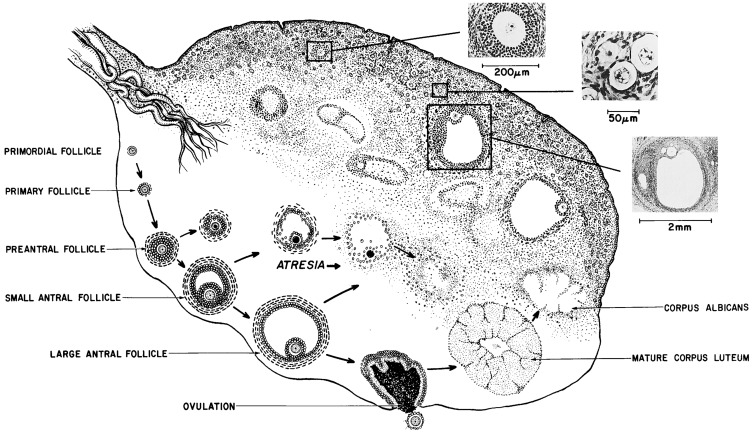
Secondary follicles and preantral follicles, characterized, respectively, by organization of a distinct theca cell layer and proliferation of granulosa cell layers, then appear successively. Preantral follicles develop at 24 to 26 weeks. Antral (graafian) follicles appear near term, and those granulosa cells enveloping the oocyte to become the cumulus. Ovarian estrogen production appears to be virtually unresponsive to gonadotropins until early antral follicles develop at near term gestational age. One or two antral follicles of 1 to 2 mm in diameter are present in the ovary at term. At this time, ovarian follicle development is complete, and the complement of ova is greater than at any other time during postnatal life (see Fig. 16.3 ), totaling 2 million, of which half appear atretic.
Both X-chromosomes are active in oocytes, and the oocytes secrete factors, such as growth differentiation factor-9 (GDF9), necessary for the induction of the granulosa cell layer that is necessary for oocyte survival. Oocyte-specific chemokines and transcription factors then coordinately direct the formation of primordial follicles and their subsequent development to primary follicles. GDF9 interaction with growth factors, such as BMP 9 and transforming growth factor beta (TGF-β), is then critical for primary follicle granulosa cell proliferation. Then preantral follicles develop when GDF9, in coordination with other growth factors, induces the theca cell layer from fibroblast-like stem cells. A host of local factors then regulate further follicle growth and development; for example, the forkhead transcription factor FOXL2, expressed specifically in granulosa cells, restrains GDF9 from prematurely activating follicle growth.
Estrogen receptor (ER) expression is critical for development of the granulosa cell layer. Insulin and androgen promote the primordial-primary follicle transition. Only upon reaching the early antral follicle stage does further follicle development become strictly dependent on FSH action.
Follicle number is determined by the balance between survival and atresia of ovarian germ cells. The endowment of ovarian germ cells has been thought to be determined during fetal life since the germ cells of the ovary, unlike those of the testes, seem to be a nonrenewing population. However, female germline stem cells can replicate, which suggests that local environmental factors extrinsic to the oocyte hold it in a state of suspended animation. The endowment of follicles may also be influenced by circulating factors, such as toxins and placental insufficiency. Some clinical evidence suggests that fetal undernutrition slows the rate of atresia. Studies in mice indicate that puberty appears to be a critical developmental window for the regulation of the follicle population because a wave of primordial follicle depletion is triggered by gonadotropin action on the intrinsic apoptotic pathway.
Placenta
The fetoplacental unit becomes the major source of sex hormones in the female fetus in the latter half of pregnancy: the fetal adrenal gland provides 17-KS as substrate for the formation of potent sex steroids by the placenta. Excess androgen, from any source, in the female fetus masculinizes genital differentiation, as discussed in other chapters. This also programs for LH elevation and insulin resistance in adult life. Another factor predisposing to postnatal insulin resistance is placental insufficiency, via hypoxemia and resultant overactivation of fetal prostaglandin production and cortisol secretion.
Adolescent
The endocrinological changes of puberty actually begin in late preadolescence before secondary sex characteristics appear, as just reviewed. The underlying basic event is increasing secretion of hypothalamic GnRH. Puberty is the consequence of the hypothalamus releasing GnRH with increasing frequency and amplitude, first only at night, then gradually throughout the day.
Increased GnRH secretion in man was initially deduced when Kastin, Job, Grumbach and their collaborators demonstrated that preadolescent children had GnRH-releasable pituitary stores of LH and FSH ( Figs. 16.6 and 16.9 ). Subsequently, it was reported that in man, the output of an immunoreactive fragment of GnRH begins to rise in late childhood and increases to adult levels during puberty. Studies in the rat suggest that hypothalamic GnRH increases through puberty.
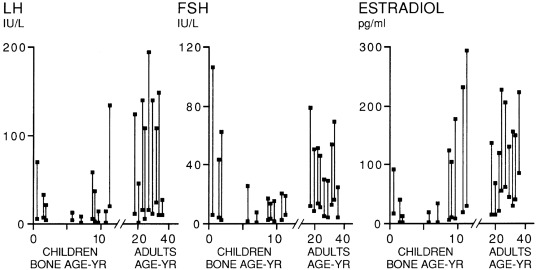
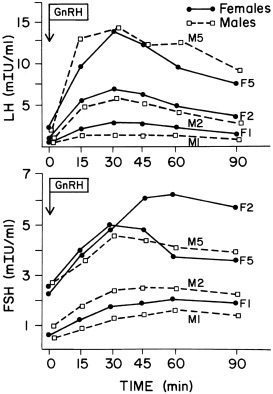
Knobil subsequently showed that puberty can be induced in the immature female rhesus monkey by administering GnRH in hourly pulses that yield blood levels of about 2000 pg/mL. Prolonged administration of GnRH according to this regimen first gradually brings about transient increases in LH and FSH. This then induces cyclic follicular development. The resultant moderate estradiol surge is of such magnitude as to result in menarche because of withdrawal menstrual bleeding in an anovulatory cycle ( Fig. 16.10 ). Continuation of the same GnRH regimen leads to development of normal monthly ovulatory menstrual periods. Physiological pulses of GnRH in man probably attain lower concentrations (200 pg/mL) and occur at slightly wider intervals than in monkeys. Consequently, LH pulses in mature women occur at intervals of approximately 1.5 hour during the follicular phases, slowing during the luteal phase.
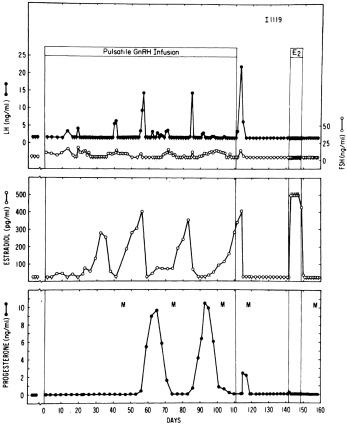
Puberty begins in response to increased GnRH secretion. Serum LH first begins to rise disproportionately to FSH; this LH-FSH disparity is particularly evident during sleep, which is reflected in responses to GnRH or GnRH agonist ( Table 16.1 ). Puberty becomes clinically apparent as thelarche when estradiol levels are sustained > 10 pg/mL. It seems likely that a rise in inhibin-B as increasing ovarian follicles develop plays a key negative-feedback role in limiting further increase in FSH levels during puberty. FSH levels become less GnRH-dependent during puberty. The mechanisms for differential regulation of FSH and LH are discussed later in this chapter.
LH (U/L) | FSH(U/L) | Estradiol(pg/mL) | Estrone(pg/mL) | Testosterone(ng/dL) | Androstene-dione (ng/dL) | DHEA (ng/dL) | 17PROG (ng/dL) | 17PREG (ng/dL) | DHEAS (mcg/dL) | |
---|---|---|---|---|---|---|---|---|---|---|
Baseline (8:00 AM) | ||||||||||
Preterm infants, 26–28 wk, day 4 | 0.1–175 | 2–200 | — | — | < 45 | 60–940 | 80–1485 | 100–2000 | 375–3550 | 125–880 |
Term infants, day 1 | — | — | 300–500 | 300–500 | 16–75 | 100–410 | 300–2600 | 150–850 | 110–3000 | 20–410 |
Term infants, day 3–7 | — | — | < 15 | < 20 | < 20 | 280 | 40–1300 | < 80 | 35–800 | 90–360 |
Term infants, maximum 1–6 mo | ≤ 1.1 | 1.2–19 | <7–55 | ≤ 20 | <10–45 | ≤ 40 | ≤ 950 | ≤ 110 | 40–765 | ≤ 115 |
Children, 1–5 y | < 0.15 | < 0.16–3.5 | 0.5 | 0.5 | 0.5 | 10–50 | 20–130 | 5–115 | 10–105 | 5–35 |
Children, 6–10 y | ≤ 0.3 | ≤ 2.9 | 0.9 | 0.9 | 0.9 | 10–75 | 20–345 | 5–115 | 10–200 | 10–115 |
Premenarcheal pubertal, 9–13 y | ≤ 7.2 | 1.1 – 9.0 | ≤55 | 10–35 | 10–35 | 40–175 | 40–600 | 16–220 | 35–350 | 35–130 |
Postmenarcheal, early follicular phase | 1.5–5.6 | 3.6–7.9 | 20–85 | 20–50 | 20–60 | 50–200 | 100–850 | ≤ 130 b | 55–360 | 75–255 |
Peak after ACTH 1–24 (30–60 minutes after ≥ 10 mcg/m 2 IV) | ||||||||||
Children, 1–5 y | — | — | <20 | 16–70 | 25–100 | 50–270 | 45–350 | 5–35 | ||
Children, 6–10 y old | — | — | <20 | 25–100 | 70–320 | 85–300 | 60–650 | 10–115 | ||
Premenarcheal pubertal, 9–13 y | — | — | 10–35 | 55–230 | 70–725 | 90–400 | 150–750 | 35–130 | ||
Postmenarcheal, early follicular phase | — | — | 20–60 | 60–250 | 250–1470 | 35–160 b | 150–1070 | 75–255 | ||
Peak after GnRH agonist (Leuprolide acetate 10 mcg/kg SC) | ||||||||||
Prepubertal, 6–9 y | 1.2–8.9 | 9.3–37 | ≤ 55 | — | < 20 c | 25–50 c | 25–70 c | < 25 c | — | — |
Premenarcheal pubertal, 9–13 y | 2.8–99 | 14–40 | 30–350 | — | 10–45 c | 25–165 c | 60–185 c | < 155 c | — | — |
Postmenarcheal, early follicular phase | 30–135 | 16–60 | 65–260 | — | 10–60 c | 50––180 c | 60–450 c | 30–135 c | — | — |
Conversion multipliers to SI units | 3.67 (pmol/L) | 3.70 (pmol/L) | 0.0347 (nmol/L) | 0.0349 (nmol/L) | 0.0347 (nmol/L) | 0.0303 (nmol/L) | 0.0316 (nmol/L) | 0.0271 (μmol/L) |
a 5 th to 95 th percentile for third-generation gonadotropin immunoassays and high-specificity steroid assays after preparatory chromatography, except for DHEAS. Values differ slightly among laboratories.
b 17-Hydroxyprogesterone early follicular phase baseline levels > 130 ng/dL are found in women who are heterozygous for 21-hydroxylase deficiency, and they often have responses to ACTH greater than those shown. 17PROG begins rising during the late follicular phase and peaks as high as 400 ng/dL in the luteal phase of the cycle.
c At 1600hr after dexamethasone administration (0.5 mg po at 1200hr) to blunt coincidental adrenocortical secretion.
Pubertal gonadotropin cycles seem to develop well before menarche and are capable of inducing cyclic estrogen production. Our working model of the nature of pituitary-ovarian dynamics in early puberty is illustrated in Fig. 16.11 .
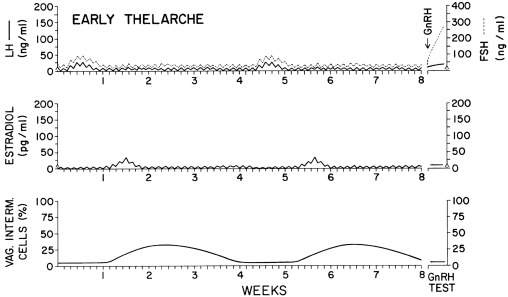
Puberty progresses as LH rises. Whereas serum FSH levels rise about 2.5-fold over the course of puberty, LH levels rise 25-fold or more. The initial change in LH secretion at the beginning of puberty is a nightly increase in LH secretion that begins within 20 minutes of the onset of sleep. Subsequently, LH increases more with the onset of sleep, stays up longer, and falls less during waking hours. As the child approaches menarche, the daytime LH levels continue to increase until the diurnal rhythm is typically lost. FSH levels follow a similar pattern, although the FSH changes are less striking. The gonadotropin diurnal rhythm during puberty seems entirely related to sleep, unlike the cortisol circadian rhythm. There is a delay of about 12 h between the peak LH level during sleep and the estradiol zenith, such that estradiol levels are maximal between late morning and early afternoon. The gonadotropin and estradiol rhythms in an early pubertal girl are shown in Fig. 16.12 .
Augmentation of the bioactivity of serum LH occurs during pubertal progression. Plasma LH bioactivity rises nearly fivefold more during the course of puberty than does LH as measured by polyclonal RIA. The change in bioactive LH is mirrored well by the “third-generation” monoclonal antibody-based immunometric (“pediatric”) assays that have very high specificity for bioactive LH epitopes. However, disparities in the ratio of bioactive to immunoreactive LH (B/I) persist with these assays, for reasons related to the molecular microheterogeneity of gonadotropins, which is discussed later. Serum FSH rises during puberty according to immunoassay more so than by bioassay.
Estradiol output increases rapidly in the year approaching menarche. This seems to be the result of a variety of autoamplification phenomena that facilitate puberty, maturation of the dominant follicle, and ovulation. These are summarized in Box 16.1 . These phenomena occur at all levels of the axis. The CNS is stimulated by preovulatory levels of estradiol to increase GnRH pulse amplitude. At the pituitary level, there is the self-priming effect of GnRH, whereby a pulse of GnRH sensitizes the pituitary to have a greater LH response to a subsequent identical GnRH pulse. Critical patterns of estradiol and progesterone secretion enhance the pituitary LH and FSH responsiveness to GnRH. At the gonadal level, the cascade of events is augmented by the FSH induction of aromatase activity and progestin production in granulosa cells, phenomena in which androgens play a synergistic role. Furthermore, FSH stimulates granulosa cell mitosis and induces LH receptors, phenomena in which estradiol may play a synergistic role. Subsequently, LH is able to further enhance the aromatase and progesterone effects. Progesterone itself plays a synergistic role in stimulating granulosa cell progesterone and prostaglandin synthesis in concert with both FSH and LH. In the rat, ovarian GnRH receptor sites also diminish just before ovulation, and at about this time the ovary changes its pattern of metabolism so that the secretion of androstanediol-3β-monosulfate decreases to levels that are no longer inhibitory to LH secretion.
Central nervous system KISS1 and GnRH secretion increases via:
- •
E2-inducing progesterone receptors
- •
Progesterone synergization with E2
Pituitary LH and FSH responsiveness to GnRH increases via:
- •
GnRH self-priming
- •
Critical patterns of E2 secretion-stimulating LH/FSH responsiveness
- •
Progesterone synergization with E2
- •
LH bioactivity increases
Gonadal responsiveness to FSH and LH increases via:
- •
FSH-inducing aromatase and progesterone in granulosa cells: androgens and progesterone synergization with this effect
- •
FSH-stimulated granulosa meiosis and FSH-inducing granulosa LH receptors; IGF-1 synergization
The preovulatory gonadotropin surge occurs when all these cascading processes culminate in activation of the positive feedback mechanism, a unique feature of the female neuroendocrine system. Positive feedback refers to the neuroendocrine system acquiring the ability to secrete a midcycle surge of LH in response to the increasing estrogen secretion by a preovulatory follicle, that is, when the ovary signals via increasing estrogen secretion that it is prepared for ovulation.
Menarche does not necessarily indicate full maturation of the neuroendocrine-ovarian axis. As the studies of Knobil illustrate (see Fig. 16.10 ), menarche can be caused by estrogen-withdrawal bleeding—and it is about half of the time—but ovulatory cycles may follow in short order. General characteristics of the mature ovary are shown in Fig. 16.4 .
The morphology of the normal adolescent ovary has long been considered polycystic, and histological examination typically has shown thecal luteinization. In the perimenarcheal period, the combination of a high number of follicles and mature gonadotropin stimulation leads to a greater number of 2 to 9 mm antral follicles within a year after menarche than at any other stage (see Fig. 16.3 ). This often leads to a “multifollicular” ultrasonographic appearance that overlaps adult criteria for polycystic ovary morphology in one-third to one-half of normal adolescents (see section on polycystic ovary syndrome).
Adult
The follicular phase of each menstrual cycle recapitulates puberty in many respects. Gonadotropin and sex hormone levels are low during the premenstrual phase of the mature cycle ( Fig. 16.13 A). Gonadotropin concentrations then increase at the time of menstruation, FSH predominating in the early follicular phase, whereas nocturnal LH pulsation is slow ( Fig. 16.13 B). LH pulsation increases to a circhoral pattern around a stable baseline, and estradiol production slowly begins as antral follicles develop ( Fig. 16.13 C). Estradiol levels gradually increase and serum FSH levels fall reciprocally ( Fig. 16.13 D). Upon formation of a dominant follicle, serum estradiol concentrations increase geometrically. This selectively begins to amplify the pituitary’s LH response to GnRH as estradiol reaches about 90 pg/mL for over 3 days ( Fig. 16.13 E).
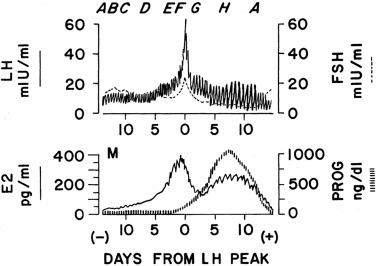
When the serum estradiol rises to over 200 to 300 pg/mL for 36 hours, the positive feedback mechanism is activated and the midcycle gonadotropin surge commences ( Fig. 16.13 F). Estradiol then appears to induce PR expression in the hypothalamus and pituitary. An increase in progesterone to 100 ng/dL facilitates the LH surge, shortens the duration of time over which estradiol is required for the surge to 24 hours, and brings about an FSH surge. The mechanism of progesterone action involves inhibition of GnRH cleavage. Androgens may also play a role in facilitating FSH and GnRH release. The LH surge is then primarily responsible for luteinizing the preovulatory ovarian follicle (see Fig. 16.13 F). At this time, LH pulses become larger in amplitude but slower in frequency and their apparent bioactivity increases. Ovulation then results.
As the follicle is disrupted by ovulation, estrogen levels fall ( Fig. 16.13 G). Meanwhile, serum progesterone increases steadily as the corpus luteum begins to form, and comes to be sustained at very high levels for several days, along with a lesser increase in 17-hydroxyprogesterone (17-OHP) and a return of estradiol to late follicular phase levels ( Fig. 16.13 H). In response to the high progesterone level, LH pulses become slow and large. In the absence of increasing human chorionic gonadotropin (hCG) from a conceptus, the corpus luteum’s life span is exhausted and its production of progesterone and estradiol wanes. Subsequently, FSH begins to rise out of proportion to LH. Shortly after the sex steroids withdraw from the scene, the endometrium sloughs, giving rise to menstrual flow. Meanwhile, the follicular growth induced earlier by FSH begins to gain momentum and the next cycle begins.
Follicular (Proliferative) Phase Ovary
The hormonal functions of the follicle have dual purposes that must be closely coordinated: to change the milieu of the ovum to prepare for ovulation and to signal the pituitary to send the signal to ovulate, that is, the LH surge. Thus the ovary is the zeitgeber for the cycle; the normal cyclic pattern of ovarian hormone secretion induces the midcycle surge of pituitary gonadotropins, even in the presence of unchanging circhoral pulses of GnRH. Ovarian hormones also augment the amplitude of the GnRH response, which is a “fail-safe” mechanism that “guarantees” a preovulatory gonadotropin surge.
Ovarian follicular development and steroid secretion in relationship to changing gonadotropin levels are illustrated in Fig. 16.14 . FSH and LH play major roles in granulosa and thecal cell differentiation, respectively, whereas a host of local factors modulate gonadotropin action. For example, follicular maturation in response to gonadotropins is enhanced by insulin-like growth factors (IGFs), TGF-β, and fibroblast growth factor, whereas it is inhibited by TGF-α.
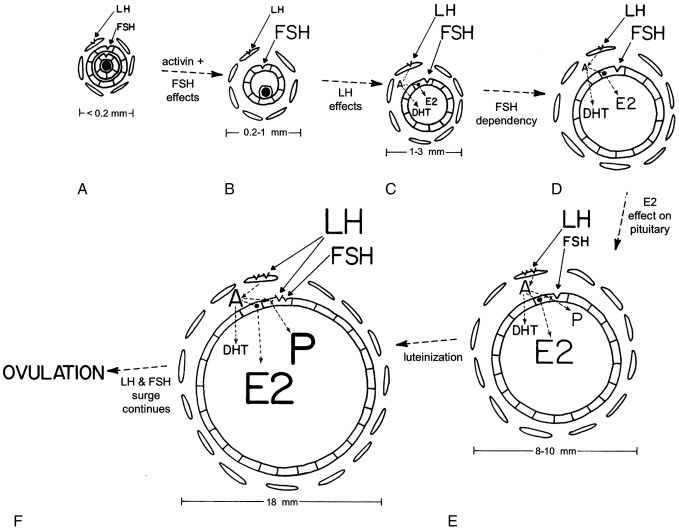
Primordial follicle growth and development is gonadotropin independent. Subsequently, granulosa cells of preantral follicles develop FSH receptors, and theca cells, which encircle granulosa cells, develop LH receptors (see Fig. 16.14 A). Activin causes FSH-independent upregulation of FSH receptors in preantral follicles, although it opposes FSH stimulation of antral follicle development. Primordial follicle growth is constitutively repressed by nuclear forkhead transcription factor Foxo3; when Foxo3 is released in response to stimulation of the PTEN-PI3K-Akt pathway, follicular growth progresses to the point where follicles become responsive to FSH.
Antrum formation requires a trace (prepubertal) amount of FSH receptor activation (see Fig. 16.14 B). FSH stimulates androgen receptor expression in primary follicles, and androgens in turn stimulate further expression of FSH receptors and the early stages of follicular growth. Androgen action is also necessary for the development of a full complement of follicles, and androgen excess stimulates excessive follicle number. LH stimulates the appearance in thecal cells of the enzymes necessary for androgen biosynthesis. Evidence that theca cells of small antral follicles form estradiol is meager.
As antral follicles grow over 2.5 mm in diameter, their granulosa cells begin to form estradiol from androgen supplied by theca cells (see Fig. 16.14 C). Androgen production at low levels may synergize with FSH to stimulate aromatase activity within the granulosa cells.
At this stage, follicles are increasingly FSH dependent and consequently uniformly FSH responsive. IGF-1 is required for follicular growth beyond the early antral stage in response to FSH. Antral follicles do not grow over 5 mm in diameter without a pubertal degree of FSH stimulation. By the midfollicular phase, the proliferation of FSH-responsive granulosa cells results in an accelerating rate of estradiol production and preferential conversion of androstenedione to estradiol rather than dihydrotestosterone (DHT) by these cells (see Fig. 16.14 D). Estradiol itself clearly stimulates proliferation of granulosa cells and oocyte survival in rodents. In humans, estradiol appears to promote antral growth independently of LH and is synergistic with FSH in bringing about the development of the dominant follicle.
A dominant follicle is selected at the beginning of the menstrual cycle from a crop of follicles that were recruited 2.5 months prior. Recruitment of a group of follicles is normally promoted by the midcycle FSH surge and regresses with increasing corpus luteum progesterone secretion. Another wave of follicle growth in the late luteal phase is promoted by the rise of FSH as luteal progesterone and estradiol secretion wanes. The selected follicle is the one that is the most sensitive to FSH (lowest “FSH threshold”). FSH is critically important during the follicular phase for optimal development of this dominant follicle. By the midfollicular phase of the cycle this follicle becomes virtually the sole source of estradiol (see Fig. 16.14 E). Typically, there is only one such follicle. Only this follicle continues to grow so as to reach a diameter of 10 mm or more. All other gonadotropin-dependent follicles undergo atresia.
At this stage, the rising estradiol level is suppressing FSH secretion and augmenting pituitary LH responsiveness to GnRH. FSH is more bioactive in the dominant follicle because it is more efficiently concentrated and because local factors increase ovarian responsiveness to FSH. The increased LH causes further proliferation of thecal cells and an increase in their LH receptor content. Androgen production is consequently increased. This synergizes with FSH to both augment aromatase activity and bring about increasing progesterone secretion by the well-estrogenized granulosa cells of these follicles. Progesterone then enhances the synthesis of both itself and estradiol. The increased thecal androstenedione production is diverted much more to estradiol than to DHT biosynthesis. Antral fluid steroid concentrations reflect these changes (see Fig. 16.15 ). Activin acts to prevent premature luteinization of granulosa cells, and activin tone seems to wane as the preovulatory phase approaches.
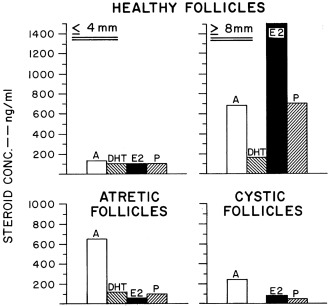
FSH next induces LH receptors in the granulosa cells, luteinizing them (see Fig. 16.14 F). Androgen and insulin synergize with FSH in this induction of LH receptors. LH then joins FSH in acting on luteinized granulosa cells to augment estradiol and progestin production.
The LH and FSH surge then occurs in response to the positive feedback action of estradiol at both the CNS and pituitary levels, an effect amplified by the rising levels of progesterone. The final steps in follicle maturation ensue rapidly: the LH surge induces granulosa cell PR and prostaglandin synthase while inhibiting cyclin gene transcription, and the FSH surge upregulates vascular endothelial growth factor. In the absence of these critical steps, ovulation and follicular rupture do not occur. Then the follicle promptly becomes desensitized to LH and FSH and ceases to grow. This is followed by an inflammatory-type response. Protease activity, prostaglandin production, and vascular permeability increase; cell junctions loosen; and cumulus cells form a mucopolysaccharide envelope around the oocyte (cumulus expansion).
Oocyte meiotic maturation resumes in response to a specific phosphodiesterase, forming the haploid gamete (secondary oocyte) and the first polar body in response to the LH surge. Ovulation of the cumulus-oocyte complex then occurs. The presence of a favorable follicular steroidal milieu is necessary both for ovulation (a premature LH surge in a subject with an unripe follicle will not result in ovulation) and subsequent developmental competence of the oocyte. Meiosis will go to completion and the second polar body will be extruded only in response to contact with a sperm.
The processes stimulating dominant follicle emergence are delicately balanced by those preventing it. It seems critical that the intraovarian concentration of androgens not become excessive. Androgen excess interferes with follicle viability beyond about the 8-mm stage and synergizes with FSH to cause premature luteinization. These interfere with the emergence of dominant follicles. Follicles arrested in their growth become atretic, and atretic follicles contain relatively high concentrations of androgens (see Fig. 16.15 ). Progesterone also suppresses further differentiation of nondominant follicles by some of the same mechanisms. High concentrations of estrogen play a critical role in inhibiting selection of the dominant follicles in primates. If there is interference with estrogenization, multiple large cystic follicles develop that are impaired in their ability to ovulate and undergo androgen-dependent atresia.
Anti-Müllerian hormone (AMH) and inhibins have emerged as other granulosa cell factors important in the regulation of follicular development. AMH is the major hormonal paracrine inhibitor of primordial follicle progression. It is produced by the granulosa cells of small growing follicles. As follicles grow, intrafollicular AMH levels rise sufficiently to inhibit recruitment of primordial follicles to the primary follicle stage; it also inhibits P450c17 activity, GnRH release, and FSH stimulation of aromatase activity. Because estradiol inhibits AMH production, there exists an intrafollicular short negative feedback loop confining AMH expression to follicles up to about 8 mm in diameter. Thus AMH appears to act as a follicular gatekeeper, ensuring that each small antral follicle produces little estradiol before selection of the dominant follicle, which allows a direct ovarian-pituitary dialogue regulating the development of the follicle selected to undergo ovulation.
Inhibin-B is the predominant form of inhibin. It arises from granulosa cells in small follicles before aromatase is expressed and is regulated by FSH in a sluggish negative feedback loop. It upregulates thecal steroidogenesis, as discussed later. Inhibin-A is a product of the preovulatory follicle (and corpus luteum) that responds to both LH and FSH.
Atresia is the fate of all except the few hundred follicles chosen for ovulation during an individual’s life span. Most follicles beyond the primordial stage become atretic. Atresia occurs by the process of programmed cell death. This apoptotic process has diverse determinants, including cell death inducer and repressor genes. FSH support becomes increasingly necessary for survival as the follicle matures, and it is normally only the follicle that has the lowest FSH threshold that escapes atresia.
Luteal (Secretory) Phase Ovary
Ovulatory rupture of the dominant follicle (see Fig. 16.13 G) is followed by invasive proliferation of capillaries and fibroblasts from the theca that breaks down the separating basement membrane. The luteinized granulosa and theca cells then intermingle and complete the luteinization process by forming the corpus luteum.
Histologically, luteinization is a process of lipid droplet accumulation that begins as the dominant follicle forms. The biochemical hallmark of the luteinized granulosa cell is the acquisition of LH receptors, with the subsequent capacity to form progesterone, 17-OHP, and estrogen in response to LH/hCG.
During its functional life span, the corpus luteum is normally the major source of the sex hormones secreted by the ovary. Corpus luteum function reaches its peak about 4 days after ovulation and begins to wane about 4 days before menstruation (see Fig. 16.13 H). Loss of sensitivity to LH and estradiol heralds luteal senescence. Regression of the corpus luteum—luteolysis—occurs if pregnancy does not provide hCG. Luteolysis is probably mediated by prostaglandins. Transformation of the corpus luteum into an avascular scar, the corpus albicans, then occurs.
Early luteal phase increases in secretion of both estradiol and progesterone cause secretory transformation and hyperplasia of the endometrium, which is necessary for implantation of the fertilized egg. Later falloff in secretion of female hormones to a level insufficient to maintain the endometrium results in menstruation (see Fig. 16.13 A). Withdrawal of progesterone is specifically responsible for constriction of spiral arteries, local prostaglandin accumulation, and subsequent ischemic necrosis of the endometrium. Normal menstrual flow then results from a complete slough of the secretory endometrium.
Documentation of ovulation can be accomplished by demonstrating collapse of the dominant follicle by ultrasonographic monitoring or by assessing the luteal transition in the estradiol/progesterone ratio, detecting the LH surge, or demonstrating a normal midluteal phase rise of serum progesterone either directly or indirectly by a rise in basal body temperature. A significant rise in basal body temperature, averaging 0.55° C, usually occurs when serum progesterone reaches 400 ng/dL or more and continues as long as that level is maintained. While the results of these methods are correlated, LH surges are sometimes inadequate to stimulate a follicle sufficiently mature to develop into a normally functioning corpus luteum, particularly during adolescence (see Luteal Phase Defects).
Regulation of the Neuroendocrine-Ovarian Axis
Factors Controlling the Onset of Puberty
Pubertal onset is under the control of a complex regulatory network that is able to dynamically respond to numerous endogenous and environmental signals. GnRH neurons play a critical hierarchical role in the direct and indirect integration of these central and peripheral signals. Reproductive development is coupled with metabolic cues that may disrupt the maturational process. The mechanisms by which neuroendocrine and genetic factors control pubertal development remain unknown. Epidemiological studies indicate that nutrition, ethnicity, and genetic factors, are normally important in the pubertal process. Environmental chemicals and chronic inflammatory disease can disrupt the process.
Evidence that there are genetic factors involved in the timing of puberty comes from multiple studies. It has been estimated that between 50% and 80% of the variation in the timing of puberty is genetically determined. Several large genome-wide association studies (GWAS) of age at menarche, examined pubertal timing in healthy females to identify the genes responsible. These studies demonstrated that there is significant genetic heterogeneity in pubertal timing in the general population that is likely to involve hundreds of common variants. The gene Lin-28 homolog B ( LIN28B ) was the first locus associated with age of menarche. LIN28B is the human ortholog of the Caenorhabditis elegans gene that controls developmental timing through micro ribonucleic acid (microRNA). Mutations in LIN28B have not been identified in humans with disorders of puberty. The 1000 Genomes Project studied genotype data in about 370,000 women and identified 389 independent signals ( P < 5 × 10 − 8) for age at menarche, with effect sizes perallele ranging from 1 week to 5 months. These signals explain only about 7.4% of the population variance in age at menarche. Genes implicated in GnRH signaling, pituitary development, hormonal regulation, fatty acid biosynthesis, and energy homeostasis have been implicated. Although mutations in these genes have been shown to cause physiological interruptions in development, their role in the initiation of puberty remains unknown. Specifically, single nucleotide polymorphisms (SNPs) in the GnRH and GnRH receptor genes have not been associated with variations in the timing of puberty in the general population.
The key in the initiation of puberty is the activation of the hypothalamic GnRH pulse generator. The molecular events that control the pulse generator include a complex interplay between both inhibitory and stimulatory factors. The mechanism of central activation of puberty first appears to be a consequence of a removal of a restraint mechanism, with a rise in gonadotropin secretion (initially during sleep). This restraint in the GnRH pulse generator is independent of the presence of gonads and more intense in males. A targeted gene approach in mice has confirmed that ERα (also termed ESR1) in Kiss1 neurons mediates feedback suppression of both Kiss1 expression and gonadotropin secretions during the prepubertal period.
However, the high levels of testosterone to which the male fetus was exposed during the period of sexual differentiation may be responsible for the more prolonged suppression of GnRH release in males than females. A role for decreased estrogen feedback sensitivity by the hypothalamic pulse generator near the time of puberty has also been shown.
Recent evidence points to an important role for the kisspeptin 1 receptor (KISS1R), a G-protein–coupled receptor (previously known as GPR54), and its ligand, kisspeptin, an excitatory neuropeptide, as a signal for pubertal GnRH release. Expression of both proteins has been found to increase before pubertal onset in association with the increase in GnRH pulse generator activity in the hypothalamus. Kisspeptin binding to its receptor on GnRH neurons stimulates GnRH secretion. Mice with knockout of Kiss1r were found to be infertile despite having normal GnRH neurons. Leptin and androgen synergistically upregulate this system, and estrogen antagonizes it. Mutations in KISS1R result in hypogonadotropic hypogonadism. However, mutations in KISS1R have not been found in boys with pubertal delay, nor have polymorphic sequences been associated with delay of pubertal development. Elegant studies in primates have demonstrated an increase in kisspeptin during pubertal development with a corresponding increase in KISS1R associated with an increase in LH. The maximum level of expression of kisspeptin and KISS1R in the hypothalamus in both males and females occurs at puberty. For each Tanner stage, girls tend to have higher kisspeptin levels than boys, potentially explaining their earlier onset of puberty.
Chronic administration of kisspeptin to immature female rats induces precocious activation of the central axis. In addition, chronic treatment with kisspeptin restores pubertal development in a rat model of undernutrition. Kisspeptin may thus not only influence the priming of puberty, but also the integration of nutritional and energy status. Although it is clear that kisspeptin activation of GnRH neurons occurs at puberty and that GnRH is increasingly sensitive to kisspeptin activation during development, other pathways contribute to GnRH activation since the hypogonadism associated with deficiency of KISS1 or KISS1R is not complete.
Neurokinin B (NKB) signaling seems to be critical for the initiation of puberty. Some kisspeptin neurons, KNDy neurons, coexpress NKB, dynorphin A, and their receptors (TAC3R and KOR), the primary function of which seems to be synchronizing kisspeptin neuron pulsatility. Receptors for NKB are also located on GnRH neurons, where they seem to modulate GnRH release or transport. Loss-of-function mutations in TAC3 and its receptor TACR3 in patients with normosmic GnRH deficiency and pubertal failure have identified a role for this neuropeptide in the control of GnRH secretion. Although kisspeptin directly regulates GnRH expression and secretion, NKB agonists failed to stimulate GnRH release in rodents. It appears most likely that a collaborative mechanism that includes both kisspeptin and NKB signaling to GnRH neurons is necessary for reproductive function in females. To investigate the interactions of kisspeptin and NKB in humans, the effects of the coadministration of kisspeptin-54, NKB, and an opioid receptor antagonist, naltrexone, on LH pulsatility were studied. Subjects receiving kisspeptin and naltrexone increased LH and LH pulsatility, whereas NKB alone did not affect gonadotropins. NKB and kisspeptin given together had significantly lower increases in gonadotropins compared with kisspeptin alone. These results suggest significant interactions between the KNDy neuropeptides on GnRH pulse generation in humans. Further, Tacr3 knockout mice are infertile, although they appear to have reversible central hypogonadism. Interestingly, a mutation in TAC3R was found in one patient with constitutional delay of growth and pubertal development (CDGP) in a study of 50 patients, whereas none have been reported in TAC3.
Disrupting mutations in makorin ring finger protein 3 (MKRN3), a paternally expressed, imprinted gene located in the Prader-Willi syndrome locus, are associated with central precocious puberty. This indicates the presence of a GnRH release-inhibiting pathway centered in the arcuate nucleus.
Initiation of puberty involves coordinated changes in transsynaptic and glial-neuronal communication. Mediating pubertal restraint are the major inhibitory systems: gamma-aminobutyric acid (GABA)ergic, some opioidergic contribution, and gonadotrophin-inhibiting hormone (GnIH), an RFamide-related peptide (RFRP). The major excitatory systems involve glutamate and kisspeptin signaling, with glial cells facilitating GnRH secretion in diverse ways ( Fig. 16.16 ). It appears that GABA receptor signaling develops in advance of glutamate signaling. Increased signaling via glutamate receptors of several types (ionotropic and metabotropic) appears to be the major proximate change in neurotransmission involved in puberty onset. At puberty, however, seemingly as a consequence of glutamate receptor signaling, GABA-A receptor signaling on GnRH neurons increases GnRH secretion. Glial cells facilitate the process through elaboration of TGFs (especially TGF-β1), IGF-1, neuregulins, prostaglandin E 2 , and the elaboration of enzymes that control the concentration of glutamate (glutamic dehydrogenase, which catalyzes the synthesis of glutamate, and glutamine synthase, which converts glutamate to glutamine).
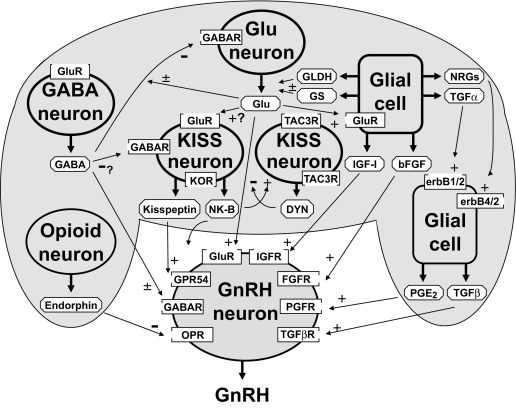
The basis of the change in neurotransmitter balance is becoming clearer. A second tier of control seems to be modulation of these processes by increased hypothalamic expression at puberty of tumor-suppressor genes that act to integrate glial-neuronal interactions. A yet higher echelon of candidate hypothalamic genes have been identified that are transcriptional regulators of the second-tier genes. These genes include Oct-2 , a regulator of the POU-domain homeobox genes, enhanced at puberty 1 ( EAP1 ), knock-out of which delays puberty and decreases fertility of mice, thyroid transcription factor I ( TTF1 ), yin yang 1 ( YY1 ), and CUX1 . Genes contiguous to elastin appear to be involved in the pace of puberty: deletion of chromosome 7q11.23 in Williams syndrome typically leads to an early normal onset but rapid pace of puberty with an abbreviated pubertal growth spurt. Substantial redundancy of these networks and the signaling neurochemicals exists since the onset of puberty is dependent on the expression of many genes, likely arranged in a coordinated network. The gene products may function as activators or repressors of targets important for pubertal onset and progression. Sex steroids have been implicated as important modulators in pubertal onset.
MicroRNAs, specifically the miR-200/429 family and miR-155, have been shown to be important in the epigenetic regulation of puberty by regulating GnRH gene transcription. miR-7a2 is critical for normal pituitary development and deficiency results in gonadotropin deficiency.
Thus the onset of puberty is controlled by an opposing increase in excitatory and a corresponding decrease in inhibitory signaling from neural networks targeting the GnRH neuron. Lesioning studies indicate that inhibitory tracts mainly seem to be routed through the posterior hypothalamus and stimulatory ones through the anterior hypothalamic preoptic area. These studies have been complemented by studies in genetically engineered mouse models. In one such model, the anteroventral periventricular nucleus (AVPV) population of neurons was shown to be the site of estrogen positive feedback in the control of pubertal progression, and kisspeptin cells in the arcuate nucleus of the hypothalamus were shown to be critical for estradiol negative feedback. Indeed, it appears that KNDy neurons integrate negative feedback of sex steroids to regulate GnRH secretion. Postmortem hypothalamic tissues were collected by The Netherlands Brain Bank, and sections were stained for kisspeptin by immunohistochemistry to determine the number of kisspeptin-immunoreactive neurons within the infundibular nucleus. This study showed that the number of kisspeptin neurons is greater in the infant/prepubertal and elderly periods compared with the adult period. In MTF transsexuals, but not homosexual men, female-typical kisspeptin expression was observed. The authors suggest that infundibular kisspeptin neurons are sensitive to circulating sex steroid hormones and that the sex reversal observed in MTF transsexuals might in part reflect an atypical brain sexual differentiation. Neonatal androgenization, which ablates the ability to generate a midcycle LH surge, was shown to selectively inhibit development of the AVPV population of kisspeptin neurons.
An overview of the systems involved in regulating the initiation of puberty is shown in Fig. 16.17 . Pubertal maturation and skeletal maturation seem to have common determinants. Abundant clinical evidence indicates that sex steroid hormones are among these determinants. Thus genes involved in sex steroid hormone metabolism and action are candidate regulators of the onset of puberty. There is limited and inconsistent data on the role of endocrine-disrupting chemicals on the timing of puberty, although some animal and epidemiological evidence supports the potential for some compounds to accelerate the time of pubertal onset and for others to delay the timing. Experience with diethylstilbestrol indicates that fetal exposures can have epigenetic effects. The growth hormone (GH)-IGF system is another determinant. GH facilitates the onset and tempo of puberty. Experimental studies suggest that this occurs through GH or IGF actions at all levels of the neuroendocrine-ovarian axis. Girls generally enter puberty when they achieve a pubertal bone age. Pubertal stage normally correlates better with the bone age (r = 0.82) than with the chronological age (r = 0.72, RLR unpublished data), particularly as menarche approaches. Skeletal age correlates better with menarche than chronological age, height, or weight, and its variance at menarche is half that of chronological age. The bone age at the onset of breast development averages about 10.75 years, and that at menarche averages about 13.0 years. Disorders that accelerate bone maturation, such as congenital adrenal hyperplasia (CAH) or hyperthyroidism, tend to advance the age of onset of true puberty. Disorders that retard skeletal maturation, such as GH deficiency, hypothyroidism, or anemia, tend to delay the onset of puberty. On the other hand, some data suggest that factors linked to intrauterine growth retardation, although not necessarily the growth retardation itself, predispose to sexual precocity.
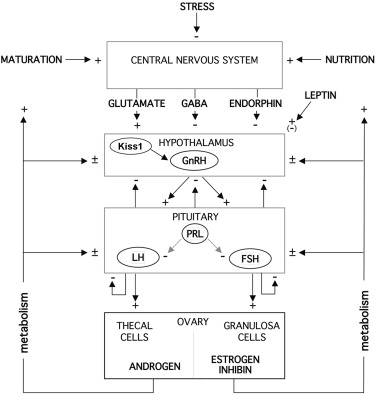
Optimal nutrition is clearly necessary for initiation and maintenance of normal menstrual cycles. The hypothesis that body fat is the weight-related trigger for pubertal development originated with the discovery by Frisch and coworkers that weight correlated with initiation of the pubertal growth spurt, peak growth velocity, and menarche better than chronological age or height. Midchildhood may be a critical period for weight to influence the onset of puberty. Suboptimal nutrition related to socioeconomic factors is an important factor in the later onset of puberty in underdeveloped than in developed countries. Conversely, obesity appears to be an important factor in advancing the onset of puberty in the United States. Some of the obesity effect may be mediated by IGF-1 and adrenal androgen.
Leptin appears to be an important link between nutrition and the attainment and maintenance of reproductive competence. Leptin deficiency causes obesity and gonadotropin deficiency. Paradoxically, prolonged leptin excess can downregulate the leptin receptor and GnRH release. Leptin is secreted by white adipose cells, acting on the hypothalamus to reduce appetite and stimulate gonadotropin secretion. A critical threshold level appears to signal that nutritional stores are sufficient for mature function of the GnRH pulse-generator and, thus to be permissive for puberty. Blood leptin levels rise throughout childhood and puberty to reach higher levels in girls than boys and are positively related to adiposity and negatively related to testosterone levels. Leptin binding protein, a truncated form of the leptin receptor, falls as puberty begins, which suggests that circulating leptin becomes more bioavailable. Whether leptin has a direct role in the pubertal activation of the GnRH pulse generator is unknown. In models of leptin insufficiency, the administration of kisspeptin induced LH secretion. Conversely, leptin’s effect on puberty did not require signaling in kisspeptin neurons in other mouse models.
Other factors also link nutrition and gonadotropic function. Part of the leptin effect is mediated by inhibition of hypothalamic neuropeptide Y (NPY) formation. NPY is a potent appetite-stimulating member of the pancreatic polypeptide family that directly inhibits GnRH release during food deprivation. However, in the preovulatory state, it stimulates GnRH release, an effect mediated by a different neural network acting on a different NPY receptor subtype on the GnRH neuron. NPY is also inhibited by the anorexogenic peptide YY (PYY), a gut hormone secreted in response to food and inhibited by GH; the pubertal fall in PYY has been postulated to permit the coordinated pubertal rise in appetite and gonadotropins. Insulin may also signal nutritional status to KNDy neurons, since deletion of the insulin receptor in KNDy neurons in genetically modified mice resulted in pubertal delay and reduced serum LH levels in both sexes. Interestingly, adult fertility was not affected.
GWAS studies of pubertal timing implicated several genes associated with body weight other than leptin and leptin receptor and include fat mass and obesity-associated protein (FTO) , SEC16 homolog B (SEC16B) , transmembrane protein 18 (TMEM18) , and neuronal growth regulator 1 (NEGR1). Rare heterozygous variants in FTO have been identified in pedigrees with CDGP associated with low body mass index (BMI) and growth and pubertal delay. Mice made heterozygous for the FTO gene knockout displayed delayed puberty, but did not manifest low body mass. Other mediators linking nutrition and puberty include melanocortin (MC)3/4 receptors, signaling from alpha-melanocyte–stimulating hormone (MSH) to increase Kiss1 expression and mediate the permissive effects of leptin on puberty, and ghrelin and mutations in the ghrelin receptor growth hormone secretagogue receptor (GHSR). A small cohort of 31 CDGP patients was analyzed for mutations in GHSR , and 5 patients were found to have point mutations in this gene.
Other cues that provide information on nutritional status to the central reproductive axis may include glucose, ghrelin, and insulin. The effect of these factors on LH pulsatility may be mediated directly at the level of the gonadotroph or indirectly by changes in GnRH secretion. There is little evidence for the role of pineal secretions in human reproduction that is found in lower animals.
The essential element for the onset of puberty is an increase in pulsatile hypothalamic GnRH secretion that is regulated by a complex interplay of excitatory and inhibitory signals that have yet to be fully understood or elucidated. During childhood the activity of the GnRH pulse generating system is restrained, an awakening of the pulse generator occurs gradually during late childhood, and the tempo of GnRH neuronal activation increases during puberty. The underlying mechanisms for all these changes are unclear. The pubertal diminution in tone of the CNS centers that inhibit hypothalamic GnRH secretion during childhood has traditionally been considered to result from decreasing sensitivity of a “gonadostat” to negative feedback by sex steroids. However, this now seems an overly simplistic concept for a mechanism that seems to involve a change in the balance of neural inhibitory and stimulatory signals that impinge upon the GnRH neuron.
Many studies have been performed to help understand the initiating developmental events or the “trigger” for pubertal onset. In fact, it is becoming increasingly clear that there is no single “trigger” for puberty, but a gradual increase in GnRH pulsatility associated with a complex interplay of factors and hypothalamic developmental programs. Thus the apparent “sensitivity of the gonadostat” seems increasingly likely to reflect the degree of activity of the GnRH neuron. That is, when GnRH secretory activity is attenuated, the pulse generator is easily inhibited; when the GnRH neuron is active, the pulse generator is relatively insensitive to negative feedback.
The integration of hypothalamic signaling systems along with the developmental changes in the control of GnRH neuronal function seem to converge to trigger the onset of puberty. In the rat, structural remodeling of the GnRH neuron was demonstrated during pubertal progression by an increase in the density of dendritic and somal spines; the percentage of total neurons with spines being lowest at birth and increased gradually postnatally until puberty. The spiny processes of neurons are the location of excitatory synapses important in neuronal plasticity. The greatest percentage of complex neurons is in the peripubertal period, with the percentage decreasing after completion of puberty. These developmental changes are correlated with an increase in excitatory synaptic input to the GnRH neuron triggering the onset of puberty in mice. Which excitatory synaptic input (e.g., glutamatergic, kisspeptinergic, or yet unknown neurochemical signals) plays a role in the pubertal increase in GnRH secretion is unknown. Whether primate or human GnRH neurons undergo synaptic excitatory remodeling during development is also unknown.
Since its discovery, numerous studies have demonstrated the expression of the kisspeptin-signaling system in several peripheral sites implicating it in biological processes, such as the regulation of ovarian function, embryo implantation, placentation, angiogenesis, and insulin secretion. However, whether kisspeptin is secreted from sites of peripheral expression and the impact on the reproductive axis are currently unclear.
Regulation of Gonadotropin Secretion
An essential feature of the mature HPG axis is the long-loop, negative-feedback control of gonadotropin secretion by gonadal secretory products, as depicted in Fig. 16.17 . The generally tonic nature of gonadotropin secretion is punctuated by two prominent types of periodicity: two- to threefold pulsations of LH above trough levels at 1.5-hour intervals and, in the sexually mature female, by a transient, midcycle, preovulatory gonadotropin surge. The latter is characterized by a greater than 10-fold, rapid rise of LH and a lesser rise of FSH. This surge is brought about by positive feedback when a critical level of estradiol, facilitated by a modest rise in progesterone, is achieved for a critical period of time, as discussed in relation to Fig. 16.13 .
Estradiol, in concert with inhibin, reciprocally regulates FSH in a sensitive, log-dose, negative-feedback loop. Progesterone in high (luteal phase) concentrations is a major negative regulator of GnRH-LH pulse frequency. Androgens have a biphasic long-loop feedback relationship with gonadotropins: at modest elevations they stimulate gonadotropin release and at very high levels they inhibit it.
Estradiol exerts triphasic, and progesterone biphasic, effects on gonadotropin secretion. As estradiol rises after the midpoint of the follicular phase it selectively reduces the FSH response to GnRH, and when it reaches preovulatory levels it transiently exerts positive feedback effects on LH and, to a lesser extent, FSH. At sustained high levels estradiol suppresses both gonadotropins. As progesterone reaches a preovulatory level, it enhances the estradiol positive feedback effect, but at the higher levels that ensue during the luteal phase, it suppresses LH pulse frequency while enhancing LH pulse amplitude.
The GnRH neurons primarily responsible for maintenance of the reproductive cycle are those of the arcuate (infundibular) nucleus ( Fig. 16.18 ). GnRH neurons are inherently pulsatile. Synchrony is promoted by fluxes of ionic calcium into these cells and autocrine GnRH inhibitory feedback. GnRH secretion is modulated by the variety of neurotransmitters and growth factors involved in initiating puberty. Synchrony of the network of GnRH neurons that accounts for pulsatility is conferred when the hypothalamic concentration of GABA periodically falls from levels inhibitory to GABAA receptors in the presence of an excitatory neurotransmitter. EAP1, a hypothalamic protein previously shown to be important for pubertal onset, has also been implicated in the control of menstrual cyclicity in primates.
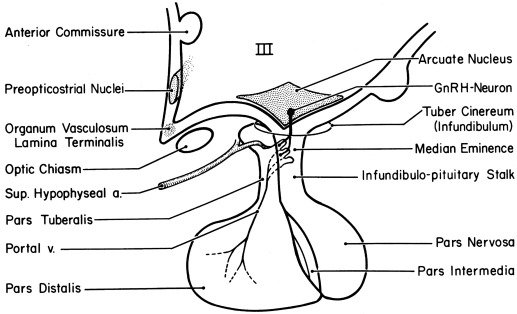
Sex steroid signals are in part conveyed to GnRH neurons indirectly. Regulation of GnRH secretion by estrogen involves in part induction of PRs in the hypothalamus. GnRH neuronal cell lines have been studied in which estradiol directly stimulates and inhibits GnRH gene expression under different experimental conditions. Although progesterone exerts its main inhibitory effect on GnRH secretion, it has effects at higher CNS levels and at the pituitary level. Prolactin suppresses both hypothalamic and gonadotropin GnRH receptor expression.
Other clinically relevant factors affecting GnRH release are sleep, endorphins (endogenous opioids), and interleukins. In sexually mature women, sleep inhibits GnRH pulse frequency and this effect seems to be amplified by female hormones. Endorphins are important physiological regulators of GnRH release after puberty has begun. Hypothalamic β-endorphin suppresses oophorectomy-initiated GnRH secretion, and opiate antagonists reverse this effect, as well as the sleep effect. The inhibitory effect of stress on gonadotropin release appears to be mediated by β-endorphin released from proopiomelanocortin in response to corticotropin releasing hormone (CRH). Interleukins also inhibit gonadotropin release. Serotonin seems to modulate LH pulsatility and facilitate the LH surge.
GnRH receptors on the gonadotroph are maintained in an optimally active state only when GnRH is delivered in pulses approximately 1 to 2 hours apart in man. Pulses substantially less frequent result in a hypogonadotropic state. Paradoxically, continuous administration of an initially stimulatory dose of GnRH results in downregulation of gonadotropin production, after an initial burst of gonadotropin release. This is the physiological basis for the success of long-acting gonadotropin agonists in suppressing puberty in children with true central precocious puberty. However, while gonadotropins are downregulated, free alpha-subunit production is elevated and responsive to GnRH.
Hypothalamic GnRH receptor function is modulated by autocrine and paracrine factors, including GnRH itself and kisspeptin. Pituitary GnRH receptors appear to be directly and indirectly downregulated by GnRH, gonadotropins, and inhibins, as well as sex steroids. LH and FSH themselves inhibit GnRH release (short-loop feedback) and inhibit their own release (autocrine feedback).
How is differential regulation of gonadotrope LH and FSH release accomplished in response to a single GnRH pulse? The frequency of the GnRH pulse is one determinant. An increased frequency of this signal stimulates LHβ-subunit gene expression, whereas slowing this signal stimulates FSH β-subunit and suppresses follistatin gene expression, altering the FSH/LH ratio. Pituitary adenylate cyclase activating polypeptide amplifies LH responses to GnRH while blocking its effect on FSH.
The sex hormone milieu is also clearly a major differential modulator of gonadotrope LH and FSH release. FSH is more sensitive than LH to inhibition by estrogen; this effect of modest levels of estradiol is of rapid onset and sustained. LH is the more sensitive to the stimulatory effects of higher estradiol levels; this effect is of later onset and short-lived. Similar relationships pertain in aromatase null mice. ER null mice have revealed ER-alpha as the predominant receptor isoform that conveys negative feedback regulation to the gonadotroph. Progesterone exerts both negative and positive feedback effects at the pituitary level, and these effects are antagonized by androgen. The progesterone metabolite 3α-hydroxyprogesterone suppresses FSH release.
Androgens have complex effects on gonadotropin dynamics. Normal androgen action facilitates the midcycle gonadotropin surge in response to positive feedback. Elevated testosterone increases baseline LH pulse amplitude and frequency while inhibiting the capacity to mount the gonadotropin surge. These actions appear to result from antagonizing progesterone action.
Inhibins of gonadal origin seem to be the major nonsteroidal-specific negative feedback regulator of pituitary FSH synthesis and secretion. Inhibins inhibit FSH release at the pituitary level, but they may act at a higher level as well. Serum levels of both inhibins rise upon FSH stimulation. Inhibin-B, produced by small antral follicles in response to FSH, is virtually the only inhibin moiety in blood during puberty. Its blood levels rise during the early follicular phase and then fall thereafter except for a small postovulatory peak, generally paralleling the changes in serum FSH; the latter peak may function to attenuate the FSH surge. Serum inhibin-A, a marker of the preovulatory follicle and corpus luteum, begins to rise in the late follicular phase and thereafter parallels levels of progesterone; its fall late in the luteal phase appears to contribute to the early follicular phase rise in the FSH level.
The structurally related activins seem to be important as regulators of both pituitary and ovary function. Activin is formed by gonadotropes themselves and its primary role is to stimulate FSH release. It also upregulates the activin binding protein follistatin, which arises within folliculostellate cells of the anterior pituitary. Follistatin, by competitively inhibiting binding of activin to its receptor, specifically inhibits activin stimulation of FSH secretion.
Infant and Child
Neuroendocrine Unit
The hypothalamic-pituitary-gonadal (HPG) axis is transiently active during the neonatal period. This is sometimes termed the minipuberty of the newborn ; unlike true puberty, the clinical manifestations are only nascent and do not progress. The regulation of neonatal gonadotropin secretion, like that during puberty, is incompletely known.
Serum FSH and LH are low in cord blood and remain low until estrogen concentrations fall from inhibitory levels upon disruption of the fetoplacental unit at birth. Then the LH and FSH levels of neonates promptly begin to rise in pulsatile fashion to early pubertal levels in the first week of life (see Fig. 16.5 ).
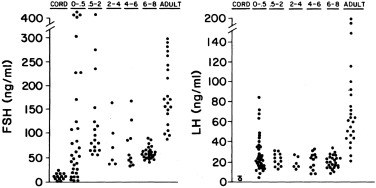
Serum LH and FSH levels rise higher in female than in male premature infants, reaching into the postmenopausal range. This sexual dimorphism seems to be related to lack of negative feedback because of lagging ovarian follicular development: antral follicle development begins near term gestational age. There is parallel hyperprolactinemia without sexual dimorphism.
At their peak between term and 4 months of age, serum gonadotropins and LH/FSH ratios are lower in girls than in boys, apparently because girls lack androgen-programmed accentuation of GnRH pulsatility. Responses to GnRH and GnRH agonist are similar to those of early puberty (see Fig. 16.6 ). In congenital agonadism, gonadotropins reach postmenopausal levels during the neonatal period.
After about 4 months postterm, gonadotropin and prolactin levels begin to gradually fall into the prepubertal range (see Fig. 16.5 ). FSH is higher in girls than in boys, a tendency that tends to persist into early childhood. This appears in part related to negative feedback by the higher activin-A and lower inhibin-B serum levels of girls than boys. GnRH secretion also appears to be greater in girls than in boys at this time.
The decline in gonadotropins may in part be related to the maturation of neural tracts that conduct inhibitory signals from the CNS and/or to an increase in hypothalamic sex steroid receptors. Hypothalamic ERs increase in a pattern reciprocal to the fall in serum gonadotropins in the rat (see Fig. 16.7 ), as do hypothalamic DHT receptors. Increasing sensitivity of the hypothalamus to sex steroid hormone negative feedback could account for the inhibitory effect of the small amounts of circulating estradiol and testosterone.
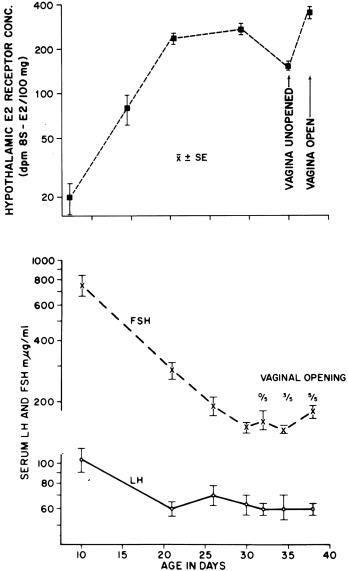
A nadir in both serum gonadotropins occurs by about 6 years of age (see Figs. 16.2 and 16.5 ). At this age, the LH and FSH response to GnRH is minimal, apparently from lack of GnRH stimulation. Furthermore, at this stage, agonadism is seldom reflected in a rise in serum gonadotropins or gonadotropin reserve.
However, gonadotropin production is not completely suppressed in midchildhood. Gonadotropins have been detected in the urine of young prepubertal children, at the limits of sensitivity of classic bioassays: LH excretion averaged 3% and FSH 15% of the adult amounts. Specific monoclonal antibody-based assays have revealed that LH falls to less than 0.2 U/L during the day whereas FSH remains detectable and that the gonadotropins produced at this stage are secreted in micropulses that approximately double in association with sleep. The gonadotropins also appear to be bioactive judging from their sensitivity to estradiol negative feedback in the primate and the active formation of antral follicles during childhood, which indicates gonadotropin stimulation, as discussed in the following section on the adult.
Between 7 and 10 years of age, even prepubertal girls experience subtle but significant increases in gonadotropin levels. This change corresponds with rising secretion of GnRH. These data indicate that the hormonal secretory pattern of the prepubertal 10-year-old child is different from that of the 7-year-old and indicate that the hormonal changes signaling the development of puberty are found late in the first decade of life, antedating by some time the development of secondary sex characteristics.
Ovary
The ovary of the infant and child is not quiescent. Initiation of growth and development of resting follicles occurs throughout childhood. The neonatal ovary typically contains an antral follicle with thecal luteinization, and the number of antral follicles approximately doubles over that in infancy by 7 years and quadruples by 9 years (see Fig. 16.3 ). All these antral follicles normally undergo atresia in childhood, and this augments the amount of stroma. As a result, by midchildhood, the ovaries of normal girls have up to five antral follicles 4 to 9 mm in diameter, and ovarian volume increases up to approximately 3.5 cc. Ovarian follicular development begins to accelerate just before the onset of clinical signs of puberty.
During the first few months of life, early pubertal blood levels of ovarian hormones are found as part of the transient activation of the HPG axis that occurs in the newborn. Serum estradiol and inhibin-B levels parallel those of FSH. In the neonatal period they begin rising to early pubertal levels, remain there for the first few months of life, and fall to low levels during childhood (see Fig. 16.8 ). Specifics about the hormonal changes are discussed later (see Normal Hormonal and Sexual Developmental Stages).
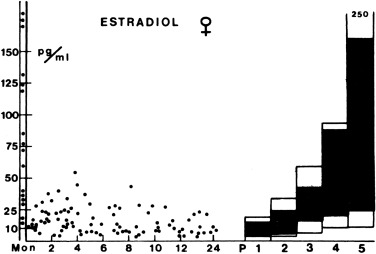
Regulation of Ovarian Secretion
Ovarian secretion results from the combined actions of LH and FSH, as discussed earlier with regards to Figs. 16.13 and 16.14 . The early follicular phase follicle functions according to the two-cell, two-gonadotropin model illustrated in Fig. 16.19 . In response to LH, androstenedione, the most abundant steroid formed in the ovary, is secreted by the theca-interstitial-stromal (thecal) cell compartment. In response to FSH regulation, aromatase then forms estrogen from precursor androstenedione in granulosa cells. FSH also stimulates granulosa cells to secrete inhibins. As by-products of the secretion of both ovarian estradiol and adrenal cortisol, androgens do not normally contribute to negative feedback regulation of gonadotropins. However, they have a biphasic effect on gonadotropin secretion: modest elevations increase GnRH pulse frequency by interfering with progesterone negative feedback and very high levels directly inhibit gonadotropin secretion.
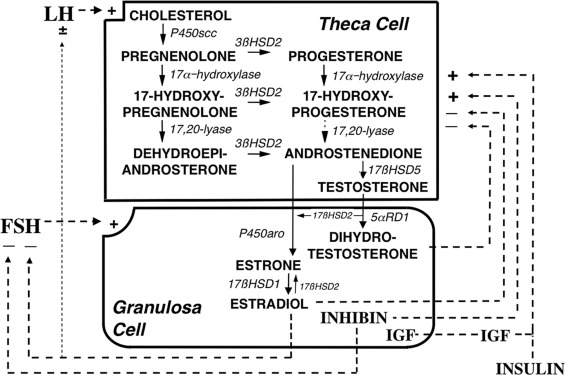
The regulation of the intraovarian androgen concentration is critical to ovarian function. Androgens are important for ovarian function. Androgens are obligate substrates for estradiol biosynthesis. Androgens also increase recruitment of primordial follicles into the growing follicle pool and then act in conjunction with gonadotropins on granulosa cells to stimulate preantral follicle development into small antral follicles, which enhances FSH upregulation of aromatase activity. Androgens also synergize with FSH to luteinize follicles by inducing LH receptors. However, in excess androgens impair selection of the dominant follicle of women; this appears likely to result from premature luteinization of follicles, thus committing the follicle to atresia. Therefore androgen synthesis must be kept to the minimum necessary to optimize follicular development. This means that the synthesis of ovarian androgens must be coordinated with the needs of the follicle. This is achieved by intraovarian intracrine, autocrine, and paracrine modulation of LH action (see Fig. 16.19 ).
LH stimulates theca cell development and steroidogenesis and is necessary for the expression of gonadal steroidogenic enzymes and sex hormone secretion. However, once adult LH levels are achieved, further LH increase normally has little further effect on androgen levels because excess LH causes homologous desensitization of theca cells. Desensitization involves downregulation of LH receptor expression and steroidogenesis. Because steroidogenic downregulation is primarily exerted on 17,20-lyase activity, which converts 17-hydroxycorticoids to 17-KS, 17-OHP levels rise in response to increased LH levels, but the rise in androgens is limited.
A model of the intraovarian interaction among the major regulators of steroidogenesis is shown in Fig. 16.19 . Stimulation of androgen secretion by LH appears to be augmented by specific intraovarian FSH-dependent factors, such as inhibins and IGFs. These processes seem to normally be counterbalanced by other FSH-dependent processes that downregulate androgen formation as LH stimulation increases. Androgens and estrogens themselves seem to mediate at least a portion of this desensitization to LH, with estrogens being critical through an ERα-dependent mechanism.
Insulin and IGFs are important coregulators of ovarian function. Insulin upregulates theca cell LH receptor sites and action and to a lesser extent estrogen biosynthesis in response to FSH. The entire IGF system is represented in the ovary: IGF-1 augments FSH receptor expression and action. and appears to mediate GH promotion of granulosa cell steroidogenesis. Insulin is equipotent with IGF-1 in stimulating thecal androgen biosynthesis, and, although insulin can act through hybrids of the insulin and IGF-1 receptors and at very high levels interacts with the IGF-1 receptor, it appears to primarily act through its own receptor.
Androgen-expressing steroidogenic cells express a previously unrecognized protein variant, DENND (differentially expressed in normal and neoplastic development) 1A.V2, that facilitates steroidogenesis: it upregulates basal and cAMP-stimulated cytochrome P450c17 and side chain cleavage activities. The mechanism by which DENND1A.V2 regulates steroidogenesis is currently unknown. DENND1A is a member of the connecdenn family of proteins, which are involved in protein trafficking, endocytotic processes, and receptor recycling. Thus it is tempting to speculate that it acts by upregulating LH receptor signaling.
Many other peptides modulate ovarian cell growth or function in response to gonadotropins. Inhibin stimulates ovarian androgen production, whereas androgens reciprocally stimulate ovarian inhibin production. Activin opposes the inhibin effect. A variety of other ovarian peptides are also capable of modulating thecal androgen synthesis. Stimulators include catecholamines, for which an intraovarian system exists, prostaglandin, and angiotensin. Inhibitors include leptin, CRH, epidermal growth factor (EGF), tumor necrosis factor, TGF-β, and GDF9. Leptin antagonizes IGF-1 effects. TGF-β is particularly interesting because it suppresses androgen biosynthesis and stimulates aromatase activity; it also stimulates meiotic maturation of the oocyte. Other peptides acting on granulosa cells include cytokines, which have diverse effects, and AMH, which inhibits aromatase. GnRH is also capable of modulating thecal steroidogenesis. A GnRH-like protein has been described in the ovary that may act through ovarian GnRH receptors to suppress steroidogenesis in the human ovary. It inhibits FSH induction of progesterone secretion, aromatase activity, and LH receptors in granulosa cells, downregulates LH receptors, and inhibits the hCG stimulation of progesterone secretion by luteal cells.
Prolactin has complex effects on steroidogenesis. In low concentrations, it enhances ovarian estradiol and progesterone secretion by increasing LH receptors. On the other hand, high levels of prolactin inhibit ovarian estradiol and progesterone biosynthesis. Prolactin also stimulates adrenal androgen production.
Adrenarche and the Regulation of Adrenal Androgen Secretion
Adrenarche denotes the onset of the increase in adrenal androgen production that gradually begins in midchildhood well before the pubertal maturation of the neuroendocrine-gonadal axis. Adrenarchal androgens contribute to the appearance of pubic hair (pubarche) and sebaceous gland and apocrine gland development.
Adrenarche results from a change in the pattern of adrenal secretory response to ACTH ( Fig. 16.20 ). It is characterized by disproportionate rises in the responses to ACTH of the △ 5 -3β-hydroxysteroids 17-hydroxypregnenolone and dehydroepiandrosterone (DHEA), whereas cortisol secretion does not change. Dehydroepiandrosterone sulfate (DHEAS) is the predominant marker for adrenarche. A DHEAS level over 40 mcg/dL is usually considered adrenarchal. Other serum androgens and precursors are ordinarily at the upper end of the prepubertal range at the onset of adrenarche.
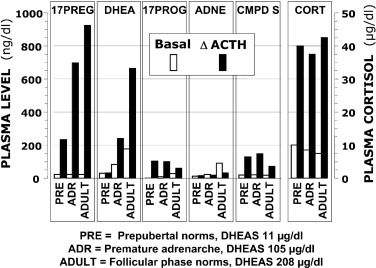
Adrenarche reflects the development of the adrenocortical zona reticularis. Humans and some higher primates are unique in having an adrenal zone with similar structure-function-developmental stage relationships. Although the zona reticularis resembles the fetal zone of the adrenal cortex in its location and function, it appears to originate from stem cells located in the outer definitive zone of the fetal adrenal gland. This zone becomes continuous at about 5 years of age and enlarges steadily over the subsequent decade. Its increasing development correlates with DHEAS levels.
This zone’s secretion pattern results from a unique enzyme expression profile: it expresses low 3β-hydroxysteroid dehydrogenase type 2 (HSD3B2), but high cytochrome b5 (an enhancer of the 17,20-lyase activity of cytochrome P450c17) and steroid sulfotransferase (SULT2A1) activities. The high secretion of DHEA and DHEAS is primarily attributable to these activities. Enhanced expression of 17β-hydroxysteroid dehydrogenase type 5 (HSD17B5) by this zone accounts for the small but significant adrenal contribution to testosterone secretion. Both testosterone and androstenedione are further metabolized by zona reticularis 11β-hydroxylase type 1 (CYP11B1), which underlies adrenal 11β-hydroxyandrostenedione and 11β-hydroxytestosterone secretion. These are further metabolized, primarily in the periphery, by 11β-hydroxysteroid dehydrogenases to their 11-keto cogeners. These 11-oxy metabolites of testosterone are one-fifth as potent as testosterone at half-maximal dosage (ED 50 ).
The factors causing and regulating zona reticularis development are unclear. Body growth is related to adrenarche. Insulin, IGF-1, and leptin have been suggested as determinants of this relationship. Insulin and IGF-1 stimulate expression of adrenal P450c17 and 3βHSD2 activities and may be involved in progenitor cell proliferation. Leptin, an adipocyte hormone, stimulates the 17,20-lyase activity of adrenocortical cells, which shunts adrenal steroidogenesis toward DHEAS production.
Nutritional status, in particular, seems to play a role in the development of adrenarche, particularly in girls. Infants born small for gestational age have higher DHEAS levels at 5 to 8 years of age, and children born large for gestational age have lower levels than those with normal birth weight. Obesity is related to DHEAS levels, and rapid weight gain during early childhood is associated with adrenal androgen levels independently of birth weight.
A pituitary hormone (“adrenarche factor”) may well be required to bring about their adrenarchal development. It has been postulated to be an ACTH-related hormone distinct from ACTH because adrenal androgen production is more sensitive to glucocorticoid suppression than is cortisol production, falls more slowly than cortisol after dexamethasone administration, and rises more sluggishly after its withdrawal. Candidates for a dexamethasone-suppressible adrenarchal factor include pro-ACTH–related peptides and CRH, but the data have not been convincing. Prolactin seems to be required. Currently the only established adrenal androgen-stimulating hormone in postnatal life is ACTH. Because the adrenarchal secretion pattern represents a change in the pattern of steroidogenic response to ACTH, an adrenarche factor need only control the growth and differentiation of zona reticularis cells or regulate their unique pattern of steroidogenic enzyme expression.
ACTH effects on adrenal androgen production are modulated by diverse signaling networks. Modulators of the androgenic response to ACTH include a stimulatory isoform of DENND1A (DENN/MADD domain-containing protein 1A; DENND1A.V2) that is known to be overexpressed in polycystic ovary syndrome (PCOS) theca cells, and BMP4, which is inhibitory. Intraadrenal cortisol may participate in the regulation of adrenal DHEA secretion through inhibition of 3β-hydroxysteroid dehydrogenase (3β-HSD) activity. In addition, interleukin-6 is strongly expressed in the zona reticularis, where it directly stimulates production of all classes of adrenal steroids independently of ACTH. Although gonadal dysgenesis is associated with earlier adrenarche, paradoxically, ovariectomy precipitates an early decline in DHEAS levels that is not reversed by estrogen replacement.
Adrenarchal levels of androgens suffice to successively initiate sebaceous gland development, apocrine gland development, and the growth of pubic hair. Sulfation of DHEA within the adrenal cortex prevents adrenal hyperandrogenism, and circulating DHEAS is a precursor for ovarian testosterone formation. It has been proposed that DHEAS elevation in response to obesity exerts a protective effect on plasma lipids. Whether adrenarche plays a more fundamental role in normal puberty is not established.
There has been considerable interest in the possibility that adrenarchal steroids play a role in human neurobiological development. DHEA and testosterone serum levels differentially correlate with specific structural developmental changes in the cerebral corticolimbic system. DHEAS and its precursor, pregnenolone sulfate, as well as the progesterone metabolite allopregnanolone, have direct nongenomic neuroactive effects, which include modulation of neurotransmitter signaling and neuroplasticity. These steroid sulfates are actively transported across the blood-brain barrier. The association of adrenarchal changes with the emergence of sexually dimorphic sexual attraction, stress-adaptive, and social maturational behavior during middle childhood, before true puberty, has led to the suggestion that adrenarchal steroids play a role in activating these behaviors. Many functions attributed to DHEAS have been inconsistent, and whether they differ from those of low-dose testosterone remains to be established.
Hormonal Secretion, Transport, Metabolism, and Action
Peptide Hormones
Peptide hormones act after binding to specific receptors located in the plasma membranes of target cells. GnRH receptors and gonadotropin receptors are members of the 7-transmembrane receptor family. These receptors are necessary for the actions of their cognate hormones. Receptors expressed in nonclassical sites are not necessarily functionally mature. Mature receptors signal after coupling to a guanine nucleotide (G-protein) subunit ( Fig. 16.21 ). Gs signaling activates adenylate cyclase and acts via phosphodiesterase-regulated cAMP to activate protein kinase A. Gq signaling activates phospholipase C, which acts via protein kinase C and Ca 2 + ; Ca 2 + may also be mobilized by other factors that influence ion channels. Phosphorylation of various cytoplasmic and nuclear proteins ultimately mediates the action of the peptide hormones, and secondarily involves the RAS and EGF signaling cascades in the case of gonadotropins. The diversity among target cells in their responses to the action of protein kinases in part relates to diversity and type of kinase, intracellular compartmentalization, substrate availability, and other differences in gene expression that are specific to each type of target cell.
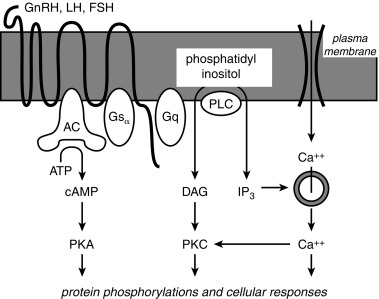
GnRH is a decapeptide [pyro]Glu-His-Trp-Ser-Tyr-Gly-Leu-Arg-Pro-Gly-NH 2 . One gene encodes the single precursor protein for both GnRH and prolactin release-inhibiting factor. GnRH not only effects prompt release of preformed gonadotropins (the “readily releasable pool”), but also stimulates the synthesis of gonadotropins (the “reserve pool”). Repeated administration of GnRH augments the pituitary responsiveness to subsequent GnRH pulses (“self-priming”). This has been ascribed partly to upregulation of GnRH receptors. GnRH has an important paradoxical effect. As discussed earlier, it acutely stimulates gonadotropin secretion, but, upon protracted, continuous administration it downregulates pituitary gonadotropin secretion. The significance of the expression of GnRH and its receptor in nonhypothalamic reproductive tissues is unclear.
An evolutionarily conserved form of GnRH (GnRH-II) acts primarily through the type 2 GnRH receptor; GnRH-II and the type 2 GnRH receptor are products of unique genes, rather than being modified products of the GnRH or type 1 GnRH receptor genes. The cell bodies of the GnRH-II neurons lie predominantly in the midbrain and only a minority project to the hypothalamic-pituitary area. GnRH-II function in humans is unknown; there is speculation that it is a neurotransmitter involved in sexual behavior. A recent study using functional neuroimaging demonstrated that kisspeptin administration enhanced limbic brain activity in response to sexual and couple-bonding stimuli and attenuated negative mood. Whether kisspeptin may become a therapeutic agent for patients with reproductive function dysfunction is currently unclear.
LH and FSH are synthesized in a single type of cell, and both are sometimes identified within the same cell. A vestigial population of hCG-secreting pituitary cells has been described. LH, FSH, and hCG are glycoprotein hormones that consist of two chains. After synthesis of these hormones on the ribosomes, the carbohydrate moieties, which constitute about 16% of the weight, are added in the rough endoplasmic reticulum and Golgi apparatus. The α chains of LH, FSH, hCG, and thyroid-stimulating hormone (TSH) are identical in amino acid sequence (92 amino acids). Although the β chain of each hormone is different in both primary amino acid sequence and length, these β chains nevertheless share 30% to 80% amino acid homology. Biological activity is conferred when an α and β chain are glycosylated and assemble within the cell. The α/β dimer is stabilized by a β-subunit derived “seat belt” that wraps around the α-subunit. Neither the isolated α nor the β glycosylated protein subunit exhibits biological activity unless noncovalently bound to one another.
The gonadotropins exhibit considerable molecular heterogeneity. The major basis for this is variation in the relative degree of glycosyl sialylation or sulfonation, steps which occur reciprocally in the pituitary gland. These differences affect in vitro and in vivo bioactivity. Polymorphisms in amino acid sequence of the LH-β and hCG-β gene also may affect the expression or bioactivity of LH or hCG. Reproductive status affects isoform distribution, with sialylated forms predominating in the hypogonadal state. Androgens increase and estrogens decrease in vitro LH biopotency by altering LH sialylation. Thus the pituitary gland contains multiple isoforms of LH and FSH that vary in bioactivity. Consequently, different pituitary LH and FSH standards, as well as serum, contain variable proportions of immunoreactive material of varying bioactivity.
One corollary of the molecular heterogeneity is that the antibodies generated from these gonadotropin moieties detect heterogeneous epitopes that are not necessarily bioactive; indeed some may even act as gonadotropin antagonists. These factors combine to cause the gonadotropin B/I to vary in a wide variety of circumstances. The purest of standards, even recombinant ones, interact very differently in the diverse immunoassay systems. Likewise, the putative level of LH or FSH in a serum sample differs substantially among immunoassays. Furthermore, bioactivity assessments vary with the bioassay model system. Monoclonal antibody–based immunometric assays yield results that correlate with, but are not necessarily equivalent to, those by bioassay. The “third-generation” immunometric assays have the advantage of being more sensitive and specific for low levels of gonadotropins in serum than polyclonal antiserum-based RIA, but B/I discrepancies remain.
The major determinant of in vivo gonadotropin bioactivity is the serum half-life. Terminal sialic acid residues retard clearance by the liver, the primary site of metabolism, whereas sulfonated ones facilitate clearance. About 10% to 15% of gonadotropins are excreted in urine according to RIA ; only about one-third of this is in a biologically active form.
LH is cleared more rapidly from the blood than FSH or hCG. LH disappears from blood in an exponential pattern: RIA indicates that the half-life of the first component is about 20 minutes and that of the second component is about 4 hours. The bioactive LH half-life is about 25% to 50% shorter. These respective components for immunoreactive FSH are 4 and 70 hours; those for hCG are 11 and 23 hours. Hormone production rates in follicular phase women, which approximate midpubertal values, are given in Table 16.2 .
Hormone | Production Rate |
---|---|
Luteinizing hormone | 615 IU/day b |
Follicle-stimulating hormone | 215 IU/day b |
Androstenedione | 3.4 mg/day |
Dehydroepiandrosterone | 7.0 mg/day |
Dehydroepiandrosterone sulfate | 7.0 mg/day c |
Dihydrotestosterone | 0.06 mg/day |
Estradiol | 0.1 mg/day |
Estrone | 0.1 mg/day |
Progesterone | 1.1 mg/day |
17-Hydroxyprogesterone | 1.2 mg/day |
Testosterone | 0.2 mg/day |
a These production rates are roughly equivalent to those in midpuberty. The average daily production of those hormones that fluctuate cyclically is substantially greater. For example, estradiol production transiently peaks to about 0.5 mg/day, and thus the average production over the monthly cycle is about 0.2 mg/day or 6 mg/mo.
b In terms of second International Reference Preparation, human menopausal gonadotropin.
c Approximate urinary production rate, expressed as unconjugated steroid.
Prolactin has structural and functional similarities to GH and placental lactogen. Prolactin has a considerable degree of structural heterogeneity; this results from genetic and posttranslational events within pituitary cells, as well as modifications, such as glycosylation in the periphery. Lactotrope growth and prolactin secretion are stimulated by estrogens. Prolactin release from the anterior pituitary is primarily under the control of hypothalamic inhibition, probably primarily mediated by dopamine. A prolactin release-inhibiting factor has been described within the same precursor protein as GnRH, thus providing a potential mechanism for reciprocal control of these two peptides. Prolactin secretion also is inhibited by thyroxine and is directly responsive to thyrotropin-releasing hormone (TRH). Estrogen and suckling are stimulatory. These signals may be positively mediated by α-MSH.
Inhibins and activins are members of the TGF-β superfamily and signal accordingly. Inhibin was discovered as the result of the search for the nonsteroidal gonadal hormone capable of specifically suppressing FSH. Activin was serendipitously discovered as the FSH-stimulating activity in the side-fractions in these studies. These hormones are formed by the differential disulfide-linked dimerization of two of three subunits (α, β A , and β B ), each encoded by a distinct gene. The combination of an α – and β-subunit yields the inhibins, inhibin-A (αβ A ) and inhibin-B (αβ B ). Activins are dimers of β-subunits, β A β A , β B β B , and β A β B (activin-A, B, and AB). Inhibin antagonizes all known actions of activin. The genes for all three subunits are differentially expressed in a wide variety of tissues. Furthermore, these factors, particularly activin, have proven to exert effects not only on gonadotropes, but within other pituitary cells, the gonads, and in nonsexual target tissues.
Steroid Hormones
The ovary and adrenocortical zona reticularis share the core of the steroid biosynthesis pathway ( Fig. 16.22 ). Gonadal cholesterol seems to be derived mostly from the cholesterol esters of low-density lipoprotein in man. Most steroidogenic steps are mediated by cytochrome P450 family members. These are the terminal enzymes in electron transfer chains, which include P450 oxidoreductase (POR) as the clinically relevant electron donor for all in the endoplasmic reticulum. The initial step in the biosynthesis of all steroid hormones is the conversion of cholesterol to pregnenolone. This is a two-stage process. The rapidity of the process depends upon the transport of cholesterol from the outer to the inner mitochondrial membrane by the steroidogenic acute regulatory protein (StAR). The conversion itself is carried out by the cholesterol side chain cleavage activity (scc) of cytochrome P450scc. The next steps are either the 3β-HSD step or 17 α-hydroxylation. 3β-HSD converts △ 5 -3β-hydroxysteroids to steroids with the △ 4 -3-keto configuration (e.g., pregnenolone to progesterone, 17-hydroxypregnenolone to 17-OHP, and DHEA to androstenedione). This step is obligatory for the synthesis of all potent steroid hormones. The type 2 3β-HSD isozyme accounts for the vast majority of the 3β activity in the human ovary and adrenal; the type 1 isozyme accounts for 3β-HSD activity in liver and skin. Pregnenolone alternatively undergoes a two-step conversion to the 17-KS DHEA along the △ 5 -steroid pathway: this conversion is accomplished via cytochrome P450c17. P450c17 is a single enzyme with 17α-hydroxylase and 17, 20-lyase activities, the latter being less efficient and critically dependent on electron transfer from cytochrome b . Progesterone undergoes a parallel transformation to androstenedione in the Δ 4 -steroid path: 17α-hydroxylation of progesterone by P450c17 forms 17-hydroxyprogesterone, but in humans P450c17 does not efficiently utilize 17-hydroxyprogesterone as a substrate for 17,20-lyase activity, so P450c17 seems to form little if any androstenedione. There is some evidence for the existence of a P450c17-independent Δ 4 -pathway to androstenedione, but most seems to be formed from DHEA by the action of 3β-HSD. Sulfotransferase 2A1 is uniquely expressed in the adrenal zona reticularis and requires the cofactor 3’-phosphoadenosine-5’-phosphosulfate synthase type 2. Other sulfotransferases (e.g., for formation of estrone sulfate) and steroid sulfatase (for the reverse reaction) are widely expressed.
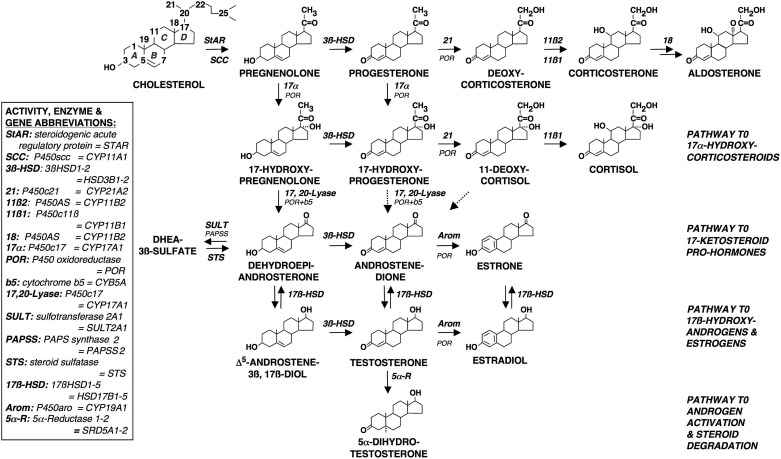
17β-Hydroxysteroid dehydrogenase (17β-HSD) and aromatase activities are required for the formation of potent sex steroids. In the ovary, androstenedione is the major precursor for sex steroids. The conversion of 17-KS to 17β-hydroxysteroids by 17β-HSDs is essential for the formation of both androgen and estrogen: testosterone is formed in the ovary by 17β-HSD type 5 (also termed aldoketoreductase , AKR,1C3), whereas estradiol formation requires 17β-HSD type 1. Aromatase activity, effected by P450arom, is essential for estradiol formation. Alternate promoters are used by the P450arom gene in the gonads, placenta, and adipose tissue, which yields alternatively spliced forms of aromatase. The organization and regulation of steroidogenesis in the developing follicle is depicted in Fig. 16.19 .
The ovary normally accounts for about 25% of testosterone secretion in the mature female (0.06 mg daily), but it secretes about 30 times as much androstenedione (1.6 mg daily). These amounts are similar to those secreted by the adrenal. However, the ovary secretes less than 1/10 as much DHEA as the adrenal.
The “production rate” of a hormone equals its secretion rate plus (in the case of hormones formed outside of endocrine glands) the rate of formation of the hormone by peripheral conversion of secreted precursors. The “blood production rate” is calculated as metabolic clearance rate X serum concentration; in the steady state the amount of hormone irreversibly leaving the plasma compartment equals the amount entering it. Because of extensive steroid interconversions, the quantity of these hormones excreted in urine is not necessarily indicative of the amount reaching target tissues. For example, so large a fraction of urinary testosterone glucuronide is formed directly from androstenedione by compartmentalized metabolism within the liver that the range of urinary excretion of testosterone in women overlaps that in men ( Fig. 16.23 ). Estrone sulfate, like DHEAS in the androgen pathway, forms a circulating reservoir of inactive estrogen that can be returned to the active pool by hepatic sulfatase activity. The blood production rates of representative steroid hormones are given in Table 16.2 and are shown for estrogens in Fig. 16.24 . During the luteal phase of the menstrual cycle, estradiol production doubles and progesterone production rises 16-fold or more.
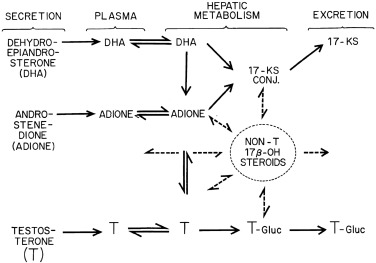
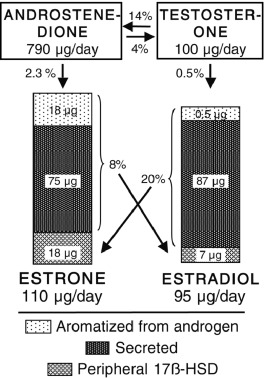
Sex hormones also have environmental origins. Structurally distinct biological estrogens include equine estrogens and plant-derived phytoestrogens. Synthetic estrogens include pharmacological compounds, such as ethinyl estradiol, diethylstilbestrol, selective ER modulators (SERMs), and some industrial chemicals, such as organochlorines (p,p”-dichlorodiphenyltrichloroethane [DDT] and others) and plasticizers (such as bisphenol A and phthalates). Endocrine-disrupting chemicals (EDCs) interfere with any aspect of hormone action, with mechanisms including mimicking or blocking hormone signaling through its receptor, and modulating the synthesis, release, transport, metabolism, binding or elimination of natural hormones. These compounds therefore may impact development of the reproductive tract and function of the reproductive axis. Animal studies have indicated that EDCs can impair ovarian development, inhibit ovarian follicle growth, increase follicular atresia, and disrupt steroid hormone levels.
Peripheral conversion of secreted prehormones by nonendocrine organs accounts for a major portion of sex hormone production. The ovary and the adrenal cortex are sources of prehormones, as well as secreted hormones. About 50% of serum testosterone (0.1 mg daily) normally is formed indirectly by peripheral conversion. Although 85% of normal estrogen production in women arises by secretion in midcycle, 50% of estrogen production can arise from extraglandular sources during the low-estrogen phases of the menstrual cycle. Peripheral formation of active steroids occurs in a wide number of sites, including liver, fat, and target organs. For example, the liver has high levels of 3α- 3β-, and 17β-hydroxysteroid dehydrogenase and 5α-reductase activities (see Fig. 16.22 ).
Peripheral androgen metabolism is not tightly regulated by the neuroendocrine system. It seems determined to some extent by the perinatal androgenic milieu, the effect of which is possibly mediated by GH. Postnatally, it is influenced by the sex hormone binding globulin (SHBG) level and the state of nutrition. Adipose tissue becomes a major site of conversion of androstenedione to both estrone and testosterone in the obese. Cytochrome P450 mixed function oxidases, the most important of which is CYP3A4, affect steroid efficacy by forming hydroxylated steroid metabolites of varying potency. They are subject to induction or inhibition by numerous drugs. Phytoestrogens increase estradiol bioavailability by inhibiting hepatic sulfotransferase.
Plasma steroids appear to reach their sites of action and metabolism by simple diffusion from the vascular compartment. The bioactive portion of serum testosterone seems to be the free testosterone and a portion of the albumin-bound testosterone that differs among tissues according to the diffusion characteristics of the vascular bed. About 98% of serum testosterone and estradiol are bound to albumin and SHBG. The SHBG concentration determines the fraction of serum testosterone and other ligands (e.g., estradiol, DHT) that are free or bound to albumin. It is also a major determinant of ligand egress from plasma ( Fig. 16.25 ). Some sex steroid effects may be mediated by SHBG binding to membrane receptors and activation of adenylate cyclase. A number of physiological and pathological states affect the SHBG level. It is increased by estrogen and thyroid hormone excess; it is decreased by androgen, insulin-resistant obesity, glucocorticoid, GH, and inflammatory cytokines.
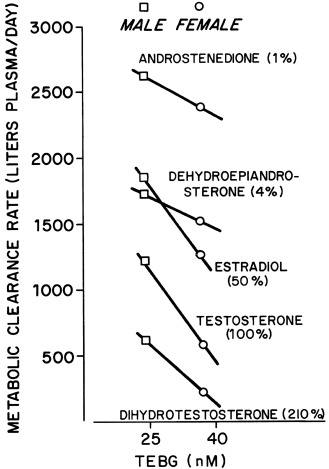
Target cell metabolism influences the cell’s response to the steroid hormones that reach it ( Fig. 16.26 ). The intracellular conversion of testosterone to DHT by one of the two isozymes of 5α-reductase is important for many but not all effects of testosterone, dependent upon the tissue-specific pattern of steroid metabolism. An important mode of testosterone action is via estradiol, notably within the brain. Although transformation is not fundamental to the mode of action of estradiol, estradiol effectiveness is influenced by target cell metabolism: the induction of 17β-hydroxysteroid oxidation in target tissues by progesterone, resulting in conversion of estradiol to the less potent estrogen estrone, counterbalances estrogenization. There is also evidence that novel steroid metabolites exert tissue-specific effects.
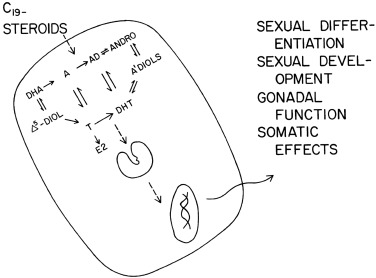
Within target cells, all steroid hormones regulate the genome similarly, starting with binding to high-affinity intracellular receptors ( Fig. 16.27 ). The steroid hormone receptors belong to the superfamily of nuclear hormone receptors. The estrogen, progesterone, and androgen receptors are, thus, homologous. Classic sex hormone effects are exerted by the interaction of steroid with receptor, not by either alone. Steroid binding triggers the dissociation of inhibitory chaperone heat shock proteins from the receptor. The active receptor-ligand complex then undergoes noncovalent dimerization and binding to its specific hormone response element on the gene. The deoxyribonucleic acid (DNA) bound steroid-receptor complex acts as a transcriptional regulator of the target gene promoter. Sensitivity to steroids is also modulated by molecular chaperone proteins that influence receptor configuration, intracellular trafficking, and receptor turnover, all which are determinants of steroid action.
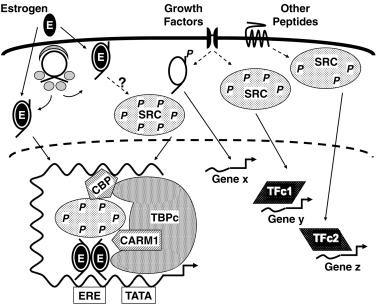
The binding properties of steroids to their cognate receptors are the initial determinants of classical steroid action. Ligand-based selectivity is one element of this interaction. Estradiol is a more potent estrogen than estrone and estriol partly because it binds best to the steroid binding domain of the ER. DHT is an inherently more potent androgen than testosterone mainly because of its higher association rate constant and its lower dissociation rate constant.
The antiestrogens tamoxifen and clomiphene and the antiandrogens cyproterone acetate and spironolactone competitively inhibit the active ligands from binding to their specific receptor sites by weakly and transiently occupying receptor sites. These differences result from potent agonists snugly fitting into the binding pocket, which induces a receptor conformation different than that of antagonist-bound receptor. One such change is the C-terminal tail of the receptor flipping over to close the “door” when a potent agonist enters; this simultaneously provides a different outer surface for interaction with coregulator proteins.
Thus ligand-based selectivity arises not only because of tighter ligand binding, but because alternative ligands produce both intermediate and unique conformational changes in the receptor, which in turn induce altered receptor interactions with coregulator proteins that result in a spectrum of activities. Thus steroids do not simply switch receptors on; they induce selective functions that depend on the nature of the coregulators that are recruited to the complex. In part, this selectivity arises because different domains of these receptors mediate these different functions. For example, the activation function (AF)-1 domain of the ER mediates interactions with mitogen-activated protein (MAP) kinase and TGF-β3, whereas the AF-2 domain mediates interactions with coregulator proteins. Coactivators, in turn, regulate alternative splicing, gene activation and repression (in some cases via their dual enzymatic functions), ubiquitin-proteosome-mediated turnover of the receptor-coregulator complex, and also determine cell-specific, site-based actions, as discussed later.
Receptor-based selectivity is a second element in steroid action. There are now known to be two isoforms of each of the sex steroid receptors. The α and β forms of the ER, although homologous, are coded by separate genes. A and B forms of the progesterone and androgen receptors exist. These forms of the PR arise by transcription from alternate promoters within the same gene, whereas those of the androgen receptor arise from posttranslational modification of a single messenger (m)RNA. These isoforms have variously been shown to manifest a differential tissue expression pattern and respond differentially to antagonists. Interactions of a steroid with different forms of receptors can regulate some target genes differentially. One role of ERβ is apparently to modulate ERα activity: ERα and ERβ can have opposite actions at AP-1 and SP-1 sites, and studies of transcriptional activity in bone and breast tissue of mice indicate a restraining effect of ERβ on responses to estradiol. Thus different target tissues exposed to the same hormone may respond selectively because of a distinct repertoire of receptor isoform expression. Some examples are notable. Although both forms of ER are expressed in most target tissues, the classic form of the ER, ERα, plays the key role in regulation of LH and estrogen actions on the uterus, breasts, sex-specific behavior, and bone. In the mouse ovary, knockout experiments show that ERα is expressed in thecal cells where it prevents androgen excess in response to LH. In contrast, ERβ is expressed only in granulosa cells, where its inhibition of androgen receptor expression is critical to prevent premature follicular atresia. Both are necessary for oocyte survival and the ability of preovulatory follicles to rupture. Furthermore, loss of both causes transdifferentiation of granulosa cells to Sertoli-like cells and massive oocyte death. Liganded PR A is essential for ovulation and is the more effective antagonist of ER action. In addition, sequence variation in hormone response elements contributes to differential gene regulation.
Effector site-based selectivity is a third variable in classical sex hormone action. In other words, the potency and character of a response to a ligand-receptor complex are not simply inherent properties of the complex. Rather, they depend on the array of effector molecules present in the site of action. Thus the array of genes expressed locally and the relative expression level of coregulators (coactivators and corepressors) are extremely important in the determination of appropriate and graded responses to a ligand by a target cell. Heterodimerization of the ER with other nuclear receptors can modulate its action. Androgens appear to exert some of their genomic effects by directly complexing with transcription factors other than the androgen receptor. Both estrogen and androgen appear to exert antiapoptotic effects in osteoblasts and osteocytes by activating a ligand-dependent, but nongenomic, kinase-mediated signaling pathway.
Nuclear receptor coactivators are critical in sensing cell-specific environmental signals and to coordinate signals emanating from membrane receptors with nuclear receptor action. Surface receptors send signals through kinase pathways that result in specific serine/threonine phosphorylation patterns of coactivators. These phosphorylation patterns serve as a code for the coactivator to preferentially bind and activate distinct sets of downstream transcription factors (see Fig. 16.27 ). Overexpression of steroid receptor coactivator-3 (SRC-3) is as important in the pathogenesis of some breast cancers as is ER positivity.
The effects of a given ligand-ER complex often differ from those of the estradiol (E2)-ER complex among cell types. This is the basis for the development of SERMs: these compounds exert effects in a tissue-specific manner depending on the cell context. The chemical structure of a SERM—or any ER ligand, for that matter—determines the configuration of the ER, resulting in a spectrum of activities from agonist to antagonist, depending on which coregulators are available for recruitment in the target cell. Raloxifene is an estradiol agonist in bone and epiphyseal cartilage but is antiestrogenic in uterus and breast; tamoxifen is estrogenic in uterus but antiestrogenic in breast and bone. Both appear to retain neural and endothelial estrogenic activity.
Nonclassical mechanisms play a role in sex steroid action. The nonclassical mechanisms are of two general types: (1) genotropic estrogen response element (ERE)-independent signaling, in which liganded ER acts as a coregulator of other transcription factors that act through their specific DNA response elements and (2) nongenotropic signaling, in which E2 binding to membrane-associated receptors, including ERα, rapidly stimulates phosphorylation pathways.
The nongenomic effects via membrane signaling occur rapidly (within minutes) and can mediate cell proliferation, apoptosis, and migration in cell-specific ways. Nongenomic E2 actions account for most of the LH-inhibitory and energy balance effects of E2. These effects can be mediated by binding to nuclear ER in plasma membrane domains provided by scaffolding proteins, such as caveolin. On such platforms, the E2-ER complex acts like a membrane receptor, coupling with G-proteins and activating cytoplasmic pathways involving SRC and MAP kinase. Androgens appear to act similarly. Nongenomic actions of nuclear PR have also been reported. Some nongenomic effects seem to involve the activation of novel G-protein–coupled transmembrane receptors for E2 and progesterone that interact either with steroids or their metabolites.
Genomic ER signaling may also be ligand independent. For example, cell membrane signaling by growth factors or other peptides stimulate ER phosphorylation. EGF activates phosphorylation of the ER and simulates diverse estrogen effects. Activation of unliganded ERα seems to be involved in repressing expression of the androgenic 17β-HSD testicular isoform in the ovary.
Steroids that act by binding to membrane-bound receptors in the brain are termed neuroactive . Neuroactive steroids synthesized in the brain are termed neurosteroids . The best documented of these effects are on neurotransmitters, which control ion channels. Allopregnanolone (3α-hydroxy-5α-tetrahydroprogesterone) and 3α-androstanediol are GABA A receptor agonists and so have sedative and antiepileptic properties. Pregnenolone sulfate and DHEAS have the opposite effect, the former also stimulating the glutamate receptors. Receptors for 5-hydroxytryptamine have been implicated in mediating some of the effects of sex steroids and certain of their metabolites. Some estrogen effects in brain are membrane-mediated.
The tissue-specific posttranscriptional events involved in sex steroid signaling are poorly understood. Estradiol and progesterone modulate the actions of each other through effects on their specific receptors: increased estrogens in the preovulatory phase of the cycle upregulate target organ receptors for both estradiol and progesterone; luteal phase levels of progesterone then suppress the production of both receptors. Estrogen prevents bone loss by blocking the production of proinflammatory cytokines. Androgen action has been reported to be mediated by prostaglandins in genitalia, and testosterone stimulates the IGF-1 system in epiphyseal cartilage.
Maturation of Sex Hormone Target Organs
Genital Tract
The Müllerian system of the embryo gives rise to the uterus, cervix, upper vagina, and fallopian tubes in the absence of AMH secretion by fetal testes during the first trimester of gestation. Genital swelling develops to engulf the base of the penis-like clitoris between 11 and 20 weeks’ gestation in parallel with the development of the ovarian follicular system. ERs are expressed in the labia minora, prepuce, and glans in females, but not in the homologous structures of males. An association between antiestrogen and genital ambiguity has been reported. Diethylstilbestrol induces dysplasia of the genital tracts. These data suggest that estrogen may play a direct role in female genital tract differentiation. However, knock-out of ERs has no obvious effect on genital tract differentiation.
The infantile uterus and cervix enlarge under the influence of estrogen during puberty. The endometrium and cervical glands then undergo cyclical changes in concert with cyclic ovarian function. In response to rising estrogen during the follicular phase of the cycle, the endometrial epithelium and stroma proliferate. The uterine glands increase in number and lengthen. Endometrial hyperplasia is prevented by progestin and androgen excess. In response to progesterone secretion after ovulation, the endometrium increases in thickness: stromal edema occurs, and the uterine glands enlarge, become sacculated, and secrete a glycogen-rich mucoid fluid. The coiled arteries lengthen further during this time and become increasingly spiral. These changes are critical to permit implantation. High-dose progestin is an effective postcoital contraceptive because it prevents implantation when taken within 3 days of unprotected intercourse.
Endocervical gland secretions lubricate the vaginal vault. The endocervical mucus is scanty and relatively thin during the low-estrogen phase of the cycle. The increase in mucus flow with advancing follicular development seems to require tissue-specific stimulation of the cystic fibrosis transmembrane regulator by estrogen. Cervical mucus becomes more viscous and elastic as estrogens rise in the later follicular phase of the cycle—the extent to which it can be stretched into a long spindle, spinnbarkeit, is a function of the estrogen level.
The mucosa of the vagina and the urogenital tract is comprised of hormone-responsive stratified squamous epithelium ( Fig. 16.28 ). The basal layer is the regenerative area. In the absence of estrogen, there is only a parabasal layer of cells over this, and the vagina is thin, with a tendency to alkalinity, which predisposes it to local infection (nonspecific vaginitis). In response to estrogen, epithelial proliferation occurs, with formation of successive intermediate and superficial layers. With this maturation, the cytoplasm of each cell first expands, leading to formation of small intermediate cells. With further estrogenization, the nuclei become pyknotic and large intermediate cells form. Greater estrogenization brings about their transformation to cornified squamous superficial cells: the cytoplasm changes from basophilic to acidophilic with the accumulation of glycogen. Resistance to infection of the fully developed vaginal mucosa results from its thickness and from its acid pH, which occurs from the fermentation of the glycogen of the superficial cells. In response to luteal phase progesterone, degenerative changes appear in vaginal mucosal cells: superficial cells decrease, the cytoplasm assumes a “crinkled” appearance, cells degenerate, and bacterial proliferation increases.
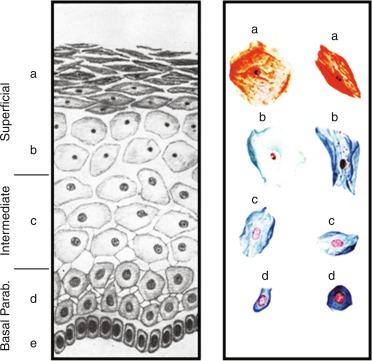
Vaginal smears show the characteristic cyclic changes in the cell types comprising the vaginal epithelium (see Fig. 16.28 ). In the prepubertal years, parabasal cells predominate, and characteristically 10% or less are small intermediate cells. A pattern consisting entirely of intermediate cells is typical of early puberty. The early follicular phase of the menstrual cycle is characterized by the predominance of large intermediate cells with few, if any, superficial cells. Peak maturation is reached at midcycle, at which time 35% to 85% of the cells seen on vaginal smear are superficial; the remainder are large intermediate cells. This cornification develops over a 1-week period in response to estradiol levels of about 70 pg/mL and persists 1 to 2 weeks after estrogen withdrawal (see Fig. 16.11 ).
Progesterone antagonizes estrogen effects on the vaginal epithelium and cervix. Inhibition of cervical ripening by progestins is used to prevent recurrent spontaneous preterm delivery.
Many normal variations have been recognized in the appearance of the hymen. The transverse diameter increases with age.
Mammary Glands
Multiple rudimentary branching mammary ducts are found beneath the nipple in infancy; they grow and branch very slowly during the prepubertal years. Estrogen stimulates the nipples to grow, mammary terminal duct branching to progress to the stage at which ductules are formed, and fatty stromal growth to increase until it constitutes about 85% of the mass of the breast. GH (via IGF-1) and glucocorticoids play a permissive role. These hormones interact with breast stroma and local growth factors to stimulate the development of breast epithelium. Lobulation appears around menarche, when multiple blind saccular buds form by branching of the terminal ducts. These effects are caused by the presence of progesterone. The breast stroma swells cyclically during each luteal phase. Full alveolar development normally only occurs during pregnancy under the influence of additional progesterone and prolactin. Prolactin does not play a role in breast growth without priming by female hormones.
Estrogen and progesterone also play a role in breast cancer susceptibility. Earlier than average age at menarche is a modest risk factor for breast cancer, regardless of BRCA status; notably, however, breast cancer risk has not been shown to be increased in precocious puberty, The BRCA1 gene normally restrains mammary growth, at least in part, by inhibiting expression of ERα and PRs, and cancer-related mutations reverse these processes.
Pilosebaceous Unit
The pilosebaceous unit (PSU), with but few exceptions, consists of both a piliary and a sebaceous component. Androgens are a prerequisite for the growth and development of PSUs in their characteristic pattern. Androgens exert their effects both on the dermal papilla, which regulates the hair growth cycle, and on PSU epithelium. Before puberty, the androgen-dependent PSU consists of a prepubertal vellus follicle in which the hair and sebaceous gland components are virtually invisible to the naked eye ( Fig. 16.29 ). Under the influence of androgens, in the sexual hair areas, the PSU switches to producing a medullated terminal hair follicle that expresses a unique type of keratin that is androgen responsive. The difference in the apparent density of sexual hair between men and women is caused by differences in the density of terminal hairs that develop in response to androgen. In the balding-prone area of scalp in individuals genetically predisposed to male-pattern alopecia, androgens weakly attenuate the hair growth cycle, so that the PSU gradually generates only vellus follicles. In acne-prone areas, androgen causes the prepubertal vellus follicle to develop into a sebaceous follicle, in which the sebaceous epithelium develops and the hair remains vellus. Adrenarchal levels of androgens suffice to successively initiate sebaceous gland development and the growth of pubic hair. Progressively, greater amounts of androgen are in general required to stimulate terminal hair development along a pubic to cranial gradient. All these effects of androgen are to some extent reversible by antiandrogens.
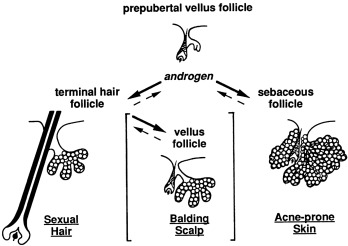
Estrogens modestly stimulate hair growth, probably by inhibiting the catagen (resting) phase of the hair cycle; this may well be caused by induction of androgen receptors by estrogen. Estrogens also directly inhibit sebum secretion. GH synergizes with androgen action on the PSU, in part via IGF-1 signaling. Retinoic acid receptor agonists antagonize the effects of androgen on the sebaceous gland by inhibiting sebocyte proliferation and differentiation. Insulin, prolactin, glucocorticoids, thyroxine, and catecholamines also play roles in PSU growth, development, and function.
Bone
Increased secretion of sex hormones clearly initiates the pubertal growth spurt. About half of this effect of sex hormones is caused by their stimulation of the GH-IGF axis. The remainder of the effects of sex steroids on skeletal growth is direct.
Differences between the actions of sex hormones contribute to women’s bones being shorter and narrower than men’s. The basis for these differences are diverse and involve interactions with IGF-1 and effects on cortical, cancellous, and periosteal bone formation.
Estrogen and androgen both stimulate epiphyseal growth. Estradiol is the critical hormone that brings about epiphyseal closure. Estrogen also is particularly effective in reducing bone turnover. To some extent these effects may be prenatally programmed. Bone accrual during puberty is a major determinant of adult fracture risk. Menarche after 15 years carries a 1.5-fold increase in fracture risk, and the risk rises with age of menarche.
Adipose Tissue
Women have a greater percentage of body fat than men. During puberty, they develop both more and larger fat cells than men in the lower body, which favors a lower body (gluteofemoral) fat distribution, in contrast to men’s upper-body (visceral) fat accumulation. The critical periods for establishment of the adipocyte population are fetal life and adolescence, after which lipid accumulation occurs primarily by cell hypertrophy. Serum levels of leptin rise throughout puberty to reach higher levels in females than males, whereas levels of the antilipolytic adipocytokine adiponectin remain stable in females but fall in males in response to androgen.
Insulin signaling is of major importance to the size and function of adipose tissue—stimulating adipogenesis (development of preadipocytes into adipocytes) and lipogenesis, while inhibiting lipolysis. Beta-adrenergic catecholamines stimulate lipolysis, countering inhibition by insulin. Visceral white adipose tissue (VWAT) lipolysis is less sensitive to insulin and more sensitive to catecholamines than subcutaneous SCWAT.
Androgens cause a masculine physique at puberty primarily by inhibiting adipogenesis reciprocally to stimulating myogenesis. They act by inhibiting adipogenic differentiation of human mesenchymal pluripotent stem cells reciprocally to their stimulation of the myogenic lineage, in a dose-dependent fashion. Local androgen generation by adipose tissue as it differentiates in response to insulin likely serves to limit insulin-generated adipogenesis. In adipocytes, androgen has been reported to inhibit lipogenesis. Androgen also inhibits catechol-stimulated lipolysis in female SCWAT, opposing the effect of insulin, but not in omental WAT.
Estrogen has been reported to suppress lipogenesis through inhibition of adipocyte lipoprotein lipase activity, according to most in vitro studies. Estradiol attenuates the lipolytic response to catecholamines, specifically in SCWAT adipocytes, but promotes lipolysis by stimulating hormone-sensitive lipase. The development of obesity in postmenopausal women and oophorectomized animal models has led to the concept that estrogen deficiency causes obesity, but the role of FSH elevation in mediating this obesity by inhibiting the formation of brown adipose tissue confounds the interpretation of all such studies. Progesterone generally counters estrogen and androgen effects on white and brown adipose tissue in experimental models.
Female sex steroid effects on serum lipids are modest. Physiological (transdermal) estradiol replacement slightly raises high-density lipoprotein cholesterol (HDL-C) and lowers very low-density lipoprotein (VLDL) triglycerides. The lower LDL-C during the normal luteal phase seems primarily because of its consumption by the corpus luteum as steroidogenic substrate. Oral estrogen replacement therapy raises HDL-C more than does transdermal replacement, but differs from it in raising VLDL-triglycerides and decreasing LDL-C, whereas androgenic progestins lower HDL-C.
Progesterone deficiency is responsible for the increased postprandial chylomicron triglyceride concentrations that occur as the result of low peripheral lipoprotein lipase activity when the pituitary-gonadal axis is acutely suppressed. In humans, use of the progesterone analogue megestrol acetate is approved to stimulate appetite and weight gain; use of progestins is associated with insulin resistance.
Muscle
Testosterone administration increases muscle mass and decreases fat mass reciprocally. Androgen does so by promoting the commitment of mesenchymal pluripotent stem cells to the myogenic lineage while inhibiting adipogenesis. Testosterone effects are exerted via androgen-receptor–mediated and nonclassical pathways. Human skeletal muscle formation of DHT is mediated primarily by type 3 5-alpha-reductase. Androgens then exert dose-related stimulation of muscle cell hypertrophy, as well as hyperplasia along with associated tissues, such as motor neurons. Hyperandrogenic women have increased muscle mass and strength, which seems to give them an advantage in athletic competition. Consequently, there is active debate about regulation of women’s androgen levels in elite athletic competition.
Central Nervous System
Concordance of gender identity (self-identification as male or female) and gender orientation (sexual preference) with gender assignment on the basis of genital anatomy is the norm, which is consistent with an important role of androgen in programming these aspects of neuropsychosocial development. However, nonhormonal genetic and epigenetic factors influence sexually dimorphic aspects of human development.
Before sex hormone differences are detectable, several genes are differentially expressed in the brains of male and female mice. Sex chromosomes directly program sexually dimorphic neuronal differentiation and behaviors, such as aggression, parenting, and social interaction. The maternally inherited X chromosome is preferentially expressed in glutamatergic neurons of the cerebral cortex, and sex-specific imprinting of autosomal genes of the hypothalamus is common and appears to be the default state in females.
Testosterone exposure during both the period of transient activation of the HPG axis in the fetal-perinatal period and again during puberty plays a role in organizing neural gene expression and development in a sexually dimorphic manner according to extensive studies in animal models, which are consistent with observations in humans. The critical period for this hormonal programming on behavioral patterns closes after puberty. This has consequences both for sex-typical neuroendocrine function and sex-typical and nonsexual behavior that is activated by the pubertal hormonal milieu.
The critical period for hormonal sensitivity of sexually dimorphic areas of the brain occurs during the early newborn period of rodents, which is thought to be comparable to the early second trimester of humans. In rats, the preoptic nucleus of the hypothalamus is larger in males, and treatment of newborn females with testosterone (or estradiol) permanently increases neuronal development to duplicate this effect and causes subsequent masculinized sexual behavior and anovulation. The anovulation results from masculinization of the LH secretory pattern (that is, both increased LH secretion and suppressed capacity to mount LH surges in response to estrogen priming) that appear to be the consequence of permanent suppression of hypothalamic PR expression.
Testosterone administration to experimental animals stimulates the growth of sexually dimorphic brain areas to adult male size. Maintenance of differences in adult nuclear size and androgen receptor expression of sexually dimorphic areas of the brain is dependent upon the ambient androgen level. Peripubertal testosterone and female hormone administration have different effects on behavior.
Several human brain structures are sexually dimorphic, some becoming so at puberty. Characteristic cortical and subcortical sex differences are discernable at puberty by structural magnetic resonance imaging (MRI), although most human brains have a mosaic of “male” and “female” features. Fetal amniotic testosterone reportedly correlates positively with many of the regional male sexual dimorphisms in the gray matter, including the amygdala, as with diverse differences in gender behavior. Functional MRI has shown endogenous testosterone levels to correlate with, and exogenous testosterone administration to females to activate, amygdala and parahippocampal regions and other brain areas in response to social-affective stimuli. Studies in transsexuals have shown that virilizing doses of testosterone affects the size of specific cortical and subcortical areas of the brain, and antiandrogen/estrogen treatment robustly inhibited hippocampal size.
Some testosterone effects are androgen specific. However, many testosterone behavioral effects appear to be mediated by intraneuronal aromatization of testosterone to estradiol in a manner that is regulated in site-specific fashion by androgen and estrogen. Thus it has been postulated that low levels of estradiol promote the development of the brain and greater amounts masculinize it. These higher levels of estradiol are generated in the male brain by neuronal aromatization of circulating testosterone. ERα knock-out in female mice reduces sexual behavior and parenting behavior, while increasing aggressiveness.
The mechanism by which estrogens mediate androgenic masculinization of rodent sexual behavior involves prostaglandin E 2 mediation. Estradiol acts through ERα to induce microglia, the resident immune cells of the brain, to secrete prostaglandin E 2 , which reduces DNA methyl transferase activity so as to release epigenetic repression of the default female behavioral state; this initiates enhanced neurite dendritic spine formation and masculinized behavior. Release of epigenetic repression appears to be an important postreceptor mechanism of testosterone action in the brain, also affecting embryonic neural stem cells. The involvement of prostaglandin E 2 in mediating the androgen effect on neural synapsing lends credence to a role for androgen excess contributing to autism spectrum disorders.
This hormonal organization of the brain involves hormone-specific effects on cell proliferation versus survival and synapse formation versus pruning. Androgens have a trophic effect on the dendritic spine cells of sexually dimorphic nuclei of rodents that promotes increased synaptic density. Estrogen alters the pattern of synaptic connections in spatially-specific and precise patterns that appear to fine-tune the sensitivity of certain regions of the brain to excitatory and inhibitory amino acids. Hypothalamic changes in synaptic remodeling have been correlated with the preovulatory surge of GnRH. There is also sexual dimorphism in cerebral progestin receptors, and progesterone attenuates testosterone effects on the brain. Progestin-estrogen administration is neuroprotective in animal studies. These hormones may counteract brain and spinal cord injury in adult women, but not in the sexually immature state.
On average, women tend to perform better than men on tasks that involve object memory, verbal skills, processing speed and accuracy, and fine-motor skills, whereas men tend to excel in visual-spatial memory, while the sexes do not differ in vocabulary or math skills. These differences are quantitatively modest, of the order of 0.4 to 1.0 standard deviation (SD), leading, therefore, to large overlaps in these skills among the sexes. The male advantage in visual-spatial skills is established by 4.5 years of age. Because both boys and girls who are congenitally sex hormone deficient are relatively poor in visual-spatial abilities, and sex hormone treatment at puberty does not ameliorate these deficits, this difference seems to be the result of estrogen-mediated patterning in both sexes. The extent to which this difference is innate or because of sociocultural factors is a subject of considerable debate.
A wide variety of gender dimorphic behaviors are found in young children, but normally they have a different character than in adults. Gender identity is established in midchildhood, probably by 3 years of age. Sexual orientation is established by 10 years of age; it has been postulated that this is dependent upon adrenarche rather than true puberty. Early pubertal amounts of androgen or estrogen have little effect on sexual behaviors, but increase some aspects of aggressive behavior. Only in later puberty is there activation of the sex drive, which has been programmed in earlier development.
Discordant gender identity (transsexualism/transgenderism) and sexual orientation (homosexuality, bisexuality) occur in a small proportion of the population. Their prevalence seems to be increased in disorders of sex development (DSD). Studies of DSD indicate that a male level of androgen acting through the androgen receptor pre- or perinatally is an important determinant of male gender behavior (role) and mildly disruptive to female gender identity. However, DSD is uncommon among homosexuals and transsexuals, whereas heritability estimates approximate 20% to 60%. Neuroimaging studies suggest that these disorders have a biological basis. Homosexuality is associated with loss of sex differences in brain structures and transsexuality with less differentiation of brain areas dealing with body and self-perception. Neuroimaging indicates that male homosexuals have a pattern of nuclear activation in response to pheromone-like chemosignals resembling heterosexual women rather than that of heterosexual men, and homosexual women have an intermediate type of activation.
Androgen and estrogen metabolites in sweat and urine, which contain unusual steroids, such as androst-4,14-diene-3-one, have been found to exert sexually dimorphic activation of the anterior hypothalamus that is independent of their odor. Therefore they appear to act as pheromone-equivalent chemosignals. Human pheromones appear to modulate the timing of ovulation and mood. It is likely that a dedicated population of olfactory receptors that project to GnRH neurons act as pheromone receptors.
Other Targets of Sex Hormone Action
Sex steroid hormones affect a wide variety of tissues in ways that are often unrecognized. An estrogen effect on stabilizing muscle integrity has been noted in muscular dystrophy.
Autoimmune disorders are in general more common in females, particularly after puberty. Estrogen downregulates blood levels of the inflammatory cytokine interleukin-6 and thymic autoimmune regulator ( AIRE ) gene expression. Progesterone has a similar effect and androgen the opposite effect on AIRE. Sex dimorphism in predisposition to autoimmune disorders is partly explicable by sex hormone action on AIRE network genes during the neonatal minipuberty. However, sex genotype influences the autoimmune system independently of sex steroids.
The cardiovascular effects of estrogen include upregulation of estrogen and PRs in vascular tissue and nongenomic effects on endothelial nitric oxide synthase. Estrogen improves the disturbed endothelial dysfunction of young hypogonadal women and is necessary for the cardioprotective effect of exercise. Estradiol replacement therapy, oral or transdermal, lowers blood pressure, although estradiol causes salt and water retention. This contrasts with contraceptives containing the more potent estrogen ethinyl estradiol, which raise blood pressure significantly, unless containing an antimineralocorticoid progestin.
Estrogens and progestins also exert hemostatic effects that are associated with increased resistance to the anticoagulant action of activated protein C. Combined oral contraceptives containing estrogen carry about a fourfold increased risk of venous thromboembolism in first-time users. The risk falls with decreasing dose of estrogen and duration of use and rises about 50% in those containing third-generation (e.g., desogestrel) and antiandrogenic progestins. Nevertheless, the risk is less than that of pregnancy. Progestin-only contraceptives are not associated with any increased risk of venous or arterial thrombosis.
The differences between the sexes in lipid levels are not explained by physiological differences in estrogen levels. Although oral estrogens raise triglycerides, this is caused by a first-pass hepatic effect. Differences in androgen (lowers HDL-cholesterol) and progesterone (lowers triglycerides and HDL-cholesterol) levels only explain part of the difference.
Normal hormonal and sexual developmental stages
The Fetus and Neonate
The fetus grows in a richer steroidal milieu than the pubertal female owing to the function of the fetoplacental unit. Concentrations of estrogens in fetal serum are extremely high. Umbilical cord plasma free testosterone levels are modestly greater than those of normal adult females. Dehydroepiandrosterone sulfate is at an adrenarchal level. The newborn shows some signs of the pubertal degree of hormonal stimulation from the intrauterine environment. Hypertrophic labia minora and superficial cell transformation of the urogenital epithelium are consistently observed estrogen effects, and a palpable breast bud is present at term in one-third of babies. The mean (SD) uterine length at birth is 4.15 ± 0.56 cm. Sebaceous gland hypertrophy results from the androgenic state, and the clitoral shaft sometimes is prominent, particularly in small premature babies.
Steroid hormone levels from birth through puberty are shown in Table 16.1 . Upon birth, withdrawal from the intrauterine environment occurs. Pituitary-gonadal axis hormone levels fall to a prepubertal-like nadir within days of withdrawal from the intrauterine environment. Menstrual bleeding and colostrum production sometimes occur as the newborn is withdrawn from the estrogenic environment. The mini-puberty of the newborn then begins.
This neonatal mini-puberty evolves according to a developmental program determined by gestational age. At term gestational age, it commences with a gradual but transient rise to pubertal hormone levels. In girls these reach maximal values in the early pubertal range at 3 to 4 months of age, about 2 months later than in boys, before they regress as seen in Figs. 16.5 and 16.8 . The activation of the HPG axis of the newborn stimulates breast and genital tract development that commonly persists for several months.
Premature neonates, in contrast to term newborns, develop high gonadotropin levels, of the magnitude seen in ovarian insufficiency, that persist until antral follicle development begins near 40 weeks, gestational age. As antral follicles develop, ovarian estrogen and AMH secretion commence, and the compensatorily high gonadotropins gradually fall to the low levels normal for term infants. Coincidentally, adrenal contributions to steroid intermediate levels are higher in premature infants because of the persistence of the fetal adrenocortical zone and immaturity in size and apparent 11β-hydroxylase activity of the definitive adrenocortical zones.
Transient ovarian hyperstimulation has been reported in preterm babies as a consequence of high gonadotropin levels persisting until or beyond late-term corrected gestational age. It manifests at several months of age as ovarian cysts with hyperestrogenism, causing genital swelling, persistent breast development, and/or delayed menstrual bleeding.
These phenomena then regress progressively through later infancy as the inhibitory tone of the neuroendocrine-gonadal axis undergoes juvenile maturation. Nevertheless, according to an ultrasensitive recombinant cell bioassay, girls’ estrogen levels in late infancy are several fold greater than those of boys, averaging 1 pg/mL and ranging up to 3 pg/mL. On occasion, there may be subclinical but detectable estrogen effects on urogenital cytology. Whether the transient “minipuberty” activity of the neuroendocrine-gonadal axis in the newborn has a programming influence on subsequent behaviors remains unclear.
The developmental pattern of serum AMH differs from that of other reproductive hormones because it reflects follicular growth and development rather than neuroendocrine activity. AMH rises from undetectable to low in cord blood to 0.6 to 4.1 ng/mL (4.3–29 pM) at 3 months; it then continues to slowly rise about 1.5-fold more to reach an adult level by the postmenarcheal period.
Childhood
As the neuroendocrine-gonadal axis becomes quiescent and the fetal zone of the adrenal cortex regresses, steroid hormone levels fall through infancy to reach a nadir in midchildhood (see Table 16.1 ). The earliest hormonal change during childhood is the adrenarchal rise in serum DHEAS that is discernable at about 6 years (see Table 16.1 ). Although childhood gonadotropin levels are low and there is seldom obvious sexual development as a consequence of prepubertal gonadotropin production, there is a low level of bioactive gonadotropin production and ovarian follicular development and occasional evidence of transient estrogen secretion. In midchildhood, GnRH agonist stimulation of gonadotropin secretion elicits a prompt small rise in estradiol secretion. AMH levels of girls rise minimally in midchildhood, to levels about 3% those of boys.
In late prepuberty, girls begin to experience increasing diurnal production of gonadotropins, and estradiol levels rise in diurnal fashion to approximate 10 pg/mL in midmorning.
Adolescence
Hormonal
The earliest hormonal changes of true puberty occur gradually during late preadolescence. Clinically prepubertal 10-year-olds develop greater average gonadotropin and sex hormone levels than do prepubertal 7-year-olds.
In the average girl, serum gonadotropins achieve pubertal levels after 8 years of age. However, the chronological age at which puberty begins varies considerably among children. Therefore the pubertal rise in gonadotropins is best appreciated by relating gonadotropin levels to pubertal stage. Daytime serum LH rises 25-fold from prepuberty to late puberty according to bioassay, but this rise is underestimated by polyclonal RIA. “Third-generation” immunometric assays, using monoclonal antibodies and a more purified standard than earlier RIAs, show a rise similar to that found by bioassay.
The hallmark of early puberty is an increase in the sleep-related rise in LH (see Fig. 16.12 ). Daytime sampling underestimates the rise in gonadotropins in early puberty because it does not detect most of this sleep-related increase. In early puberty, current assays show that LH rises during sleep to reach peaks in the lower adult range, generally above 1.0 U/L and then typically falls during the day to 0.6 U/L or less. A single daytime sample also does not necessarily truly represent a child’s pubertal status because it does not account for episodic and cyclic changes in gonadotropin secretion. The serum LH response to GnRH is slightly more indicative of the pubertal status than a morning basal sample (see Fig. 16.9 ) ( Table 16.1 ). An LH level 1-h post-GnRH agonist of 3.2 U/L or more is 90% sensitive and 5.5 U/L or more is 95% specific for the onset of puberty in girls. The response of LH to GnRH or GnRH agonist administration increases more than that of FSH during puberty, with a resultant increase in the LH:FSH ratio. GnRH agonists add a dimension to GnRH testing: they provide a sufficiently potent and prolonged stimulus to LH and FSH release to bring about an increase in ovarian estradiol secretion in pubertal girls. These responses likewise increase characteristically with sexual maturation (see Fig. 16.6 ).
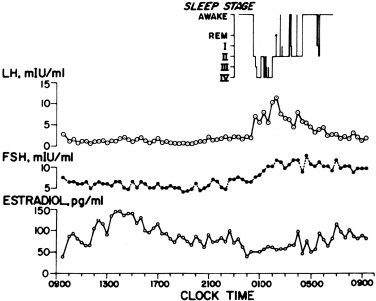
Sex hormone levels rise further as the consequence of ovarian and adrenal maturation. Pubertal levels are intermediate between those of prepubertal and sexually mature individuals. Table 16.1 shows typical normal ranges for serum levels of the major steroid hormones. Once pubertal levels of estrogens and androgens are achieved, their effects ordinarily become obvious within 6 months.
Serum AMH levels stabilize at an adult level of 0.5 to 6.2 ng/mL (3.5–45 pM) in postmenarcheal females with normal ovarian morphology. They do not fluctuate during the normal menstrual cycle, reflecting a balance between recruitment of growing follicles and growth of antral follicles. The AMH serum level is an indicator of the number of growing follicles, and thus indexes the size of the oocyte pool (“ovarian reserve”); AMH begins to fall with the oocyte pool in the premenopausal adult and becomes undetectable after menopause. AMH levels also reflect the intrafollicular androgenic status of fertile females, probably because androgens stimulate the early phases of follicular growth. Serum prolactin rises moderately in females at about 14 years of age. This may be a response to estrogen secretion because it does not occur in boys.
Clinical
The first physical sign of puberty is breast development (thelarche). In a minority of girls, pubic hair development (pubarche) occurs before thelarche. Thelarche represents a response to estrogen, and pubarche a response to androgen. When pubarche precedes thelarche, it is usually a reflection of adrenal androgen production (adrenarche) rather than a sign of true puberty. The stages of breast and pubic hair development are shown in Fig. 16.30 . Tanner stage 1 is prepubertal. The initial stage of breast development (stage 2, B2) is appreciated as a palpable subareolar bud before it can be seen as an elevation. Stage B3 is obvious enlargement and elevation of the whole breast. Stage B4, the phase of areolar mounding, is very transient and may not necessarily appear. Stage B5 is the stage of attainment of mature breast contour. Pubic hair first starts as presexual pubic hair development (PH2)—hairs which are shorter, lighter, and straighter than sexual pubic hairs, but longer than vellus body hair. Hypertrichosis is sometimes mistaken for stage 2 pubic hair; these can be differentiated by comparing genital hair to that on the forearm. Sexual pubic hair development (PH3)—curly terminal (long, dark) hair—usually commences on the labia majora before spreading to the pubis. Pubic hair then gradually progresses to the mature female escutcheon (inverted triangle pattern, stage 5). Axillary hair usually appears about a year later than pubic hair and passes through similar stages.
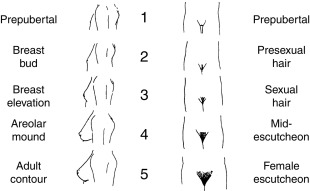
The age at which pubertal milestones are normally attained is not known with certainty. There has been considerable debate about the normalcy of pubertal changes between 6.0 and 8.0 years of age. The debate stems from office practice observations that breast and pubic hair development were found more frequently than expected in black girls in this age range. The prevalence of pubertal milestones has subsequently been estimated for the general US population by modeling cross-sectional data collected 1988 to 1994 on children 8.0 years of age and older by the National Health and Nutrition Examination Survey III (NHANES III). NHANES data had the advantage of nationally representative sampling, but the breast stage data was based on observation and so data quality was questionable, and modeling assumptions that permit extrapolation to younger ages may not be valid. The median ages at which the major pubertal milestones were attained in normal-weight US girls according to this database are given in Table 16.3 . These data indicated that puberty begins before 8.0 years in less than 5% of the normal general female population, although breasts may normally appear during the seventh year in blacks and Mexican Americans.
Stage | 5% | 50% | 95% |
---|---|---|---|
Breast Stage 2 | 8.25 | 10.2 | 12.1 |
Pubic Hair Stage 3 | 9.25 | 11.6 | 13.9 |
Menarche | 11.0 | 12.6 | 14.1 |
A more contemporary study of female puberty was conducted from 2004 to 2011. The design was improved: data collection was longitudinal and breast development was ascertained by palpation. However, although the design included broad racial/ethnic and socioeconomic representation, it was not nationally representative, the study population enriched for Asians, for example. The overall distribution of pubertal timing was shifted to earlier ages by approximately 0.75 year for breast development and 0.35 year for menarche compared with NHANES III. The data confirmed that normal-weight non-Hispanic black girls achieved pubertal milestones earlier than white girls, by about 0.75 to 1.0 year for thelarche and 0.5 to 0.75 year for menarche; thus thelarche normally may occur in the seventh year of life in blacks. Pubertal milestones in Asian girls were similar to those in whites, and in Hispanics to blacks.
Obesity and ethnic factors now appear to be independent factors that advance the onset of puberty and menarche, with obesity having the greater influence. Longitudinal study indicates that obesity is associated with advancement of the age of thelarche (0.7 year) more closely and twice as much as advancement of the age of menarche (0.3 year).
Pubertal tempo, the span of time between the onset of breast development (B2) and menarche, is normally 2.3 ± 1.0 years. However, obesity is paradoxically associated with significant advancement of the age of thelarche while significantly slowing pubertal tempo and preserving height potential. It is possible that the advancement of the age of thelarche by obesity is not entirely because of an advancement of neuroendocrine puberty but rather arises in part from extragonadal formation of estrogen from adrenal precursors in excess adipose tissue; this may explain, in part, the apparent blunting of early neuroendocrine puberty in obese girls with early thelarche. On the other hand, a subgroup of early maturers with a history of intrauterine growth retardation seem to have an unusually rapid tempo of puberty and lose height potential.
The onset of puberty is more closely related to an individual’s bone age than to chronological age. This is particularly important in the case of subjects who are later than average in entering puberty, as discussed previously. The great majority of girls can be expected to begin puberty by the time their skeletal age reaches 12.5 years and to experience menarche by the time skeletal age reaches 14 years.
The pubertal growth spurt in girls occurs during early adolescence. The peak of linear growth velocity corresponds most closely with stage B2 and the increase in serum alkaline phosphatase levels with B3. Fat accumulation increases and fat distribution changes as well. As a consequence of these pubertal changes occurring out of phase with chronological age, girls begin to differ considerably in size and habitus during the ninth year of life.
Adult Menstrual Cycle
The menstrual cycle of young adults averages 28 days in length (normal adult 24–38 days). The variation in cycle length is almost entirely because of differences in the duration of the follicular phase. The luteal phase, the time between ovulation and the onset of menses, invariably lasts 14 ± 1 (SD) days.
The cyclic changes of LH, FSH, estradiol, and progesterone serum levels during the menstrual cycle are shown in Fig. 16.13 . Diurnal and episodic fluctuations are superimposed upon these cyclic changes. Since testosterone and androstenedione have both adrenal and ovarian origins, their levels fluctuate to some extent in cyclic, diurnal, and episodic patterns. For example, testosterone levels tend to be 20% greater in the morning than in the evening and to double in midcycle. The normal range for most of the important ovarian sex hormone levels of women during the early follicular phase of the menstrual cycle is given in Table 16.1 . Progesterone levels are below 100 ng/dL until the periovulatory phase of the cycle and then peak to over 500 ng/dL in the midluteal phase. Hormonal production rates for the midfollicular phase in women are given in Table 16.2 . Serum prolactin increases transiently in midcycle with maximum ovarian estradiol secretion. Prolactin levels also transiently rise in response to mammary stimulation and psychological factors.
Normal Variations in Pubertal Development
Although the onset of breast development (stage B2) characteristically precedes the appearance of sexual pubic hair (PH3) and the onset of menses substantially (see Table 16.3 ), there is considerable variation in the sequence of these events. Pubic hair may appear before breasts begin to develop, a situation arising from lack of direct linkage between adrenarche and gonadarche. Menarche may occur within months after the appearance of breasts; however, this is so unusual that its occurrence demands exclusion of an abnormal hyperestrogenic state.
A common normal variant is the unilateral onset of breast development. Unilateral breast development may exist up to 2 years before the other breast becomes palpable. This phenomenon seems related to an asymmetry that normally persists into adulthood. Excisional biopsy of a normal unilateral breast papilla in search of a nonexistent tumor should be avoided, because such a procedure excises the entire breast anlage.
Two extreme variations of normal are the most common causes of premature sexual development. These are the isolated appearance of breast development (premature thelarche) and the isolated appearance of sexual hair (premature pubarche).
Premature Thelarche
Breast development before 8.0 years is traditionally considered premature. Premature thelarche is a very mild, non- or slowly progressive incomplete form of premature puberty that is a variant of normal. In the 6- to 8-year age group, it is usually because of obesity, which may either accelerate the onset of puberty, account for increased peripheral estrogen production, or cause artefactual adipomastia. Otherwise, it usually seems to be caused by idiopathic subtle overfunction of the pituitary-ovarian axis, occurring in those girls whose FSH levels tend to be sustained about the upper end of the prepubertal normal range. Average serum levels of FSH at baseline and in response to GnRH are significantly increased, whereas those of LH are not. Estradiol levels are generally below the level of detection in most standard assays, but are significantly elevated according to ultrasensitive assay, and intermittent low-grade estrogenization of the urogenital mucosa is sometimes found (see Fig. 16.11 ). Ovarian ultrasound examination shows an increased prevalence of antral follicles (“microcysts”) and uterine enlargement. Nevertheless, a growth spurt does not occur, the bone age advancement rate is not abnormal, and menses do not appear until the usual age.
In infants, the syndrome seems to be caused by a lag in inhibition of the transient activation of the HPG axis of the newborn and is usually unsustained. In older children, the breast development is more likely to persist. A subgroup with “exaggerated thelarche” has an increased growth rate with relatively proportionate bone age advancement. Their unsustained or intermittent neuroendocrine activation seems to lie on a spectrum between ordinary premature thelarche and true sexual precocity ( Fig. 16.31 ). However, the McCune-Albright syndrome mutation is found in the peripheral blood of about 25% of such patients. Premature thelarche may be the first sign of feminizing disorders (see Precocious Puberty). Therefore follow-up of these patients is indicated.
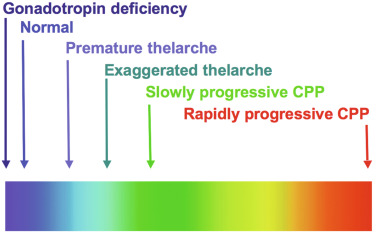
Premature Pubarche
The isolated appearance of sexual pubic hair before 8.0 years of age in girls (premature pubarche) is usually caused by premature adrenarche, as discussed next. However, it may occur at androgen levels that are normal for preschool children (idiopathic premature pubarche). This likely reflects increased sensitivity of the PSU to low preadrenarchal androgen concentrations, analogous to idiopathic hirsutism in adults, in which increased sexual hair growth occurs in the absence of other evidence of hyperandrogenism. The mechanism may be caused by increased androgen receptor gene activity.
Premature adrenarche is a very mild, slowly progressive incomplete form of premature puberty that is usually a variant of normal. It usually appears to be caused by advanced development of the zona reticularis as indicated by increase of adrenal androgen levels above those of preschool children. The condition is ordinarily detected when a child presents with premature pubarche or other clinical manifestation of androgen action, such as adult-type body odor or acne. The androgen excess is ordinarily so subtle that there is no obvious growth spurt, the bone age typically does not advance abnormally, and there are no other signs of sexual maturation. The diagnosis requires biochemical demonstration of a serum steroid pattern indicative of adrenarche before 8 years of age in girls, for example, DHEAS 40 to 130 mcg/dL and other androgen levels only slightly elevated for age (see Table 16.1 ). The term is sometimes used to describe biochemical evidence of adrenarche irrespective of clinical manifestations.
Exaggerated adrenarche is a clinically extreme type of premature adrenarche. These girls have clinical features that suggest subtle androgen excess (e.g., significant bone age advancement, but not clitoromegaly) or insulin resistance (e.g., central adiposity or acanthosis nigricans). Such children generally have a slightly advanced onset of true puberty, but height potential is not compromised. The term exaggerated adrenarche has been variously applied but is here when the atypical clinical picture is associated with serum androgen levels above normal for early puberty (see Table 16.1 ), for example, DHEAS above 130 mcg/dL or testosterone over 35 ng/dl, or bone age above height age by 20%. Virilizing disorders should be excluded in girls with exaggerated adrenarche so defined.
The cause of premature adrenarche usually seems simply to be caused by advanced onset of normal zona reticularis development, but it sometimes seems to be an early manifestation of the steroidogenic dysregulation of polycystic ovary syndrome. Premature adrenarche appears to carry about a 10% to 20% risk of developing polycystic ovary syndrome; it is unclear whether those with exaggerated adrenarche are primarily at risk. Premature adrenarche with insulin resistance is independently associated with obesity, rapid weight gain during early childhood, and low birth weight. The IGF-1 excess of GH treatment may be a risk factor. Associations also exist between premature adrenarche and temporal lobe lesions and poliomyelitic scoliosis. Heritable factors are also important. The heritability of DHEAS sulfate blood levels is estimated at 26% to 66%. Carriers for CAH may be overrepresented in this group. Epigenetic programming seems to be a factor: prenatal virilization of nonhuman primates causes functional adrenal hyperandrogenism as part of the PCOS spectrum, and fetal undernutrition has been postulated to program for insulin resistance by activating the limbic-hypothalamic-pituitary-adrenal system to cause fetal corticoid excess.
The differential diagnosis of premature pubarche and adrenarche includes virilizing disorders, of which nonclassic CAH is the most common. Girls with premature adrenarche should be followed through puberty for the possible development of hyperandrogenism. A suggested approach to rule out serious hyperandrogenic disorders in childhood is suggested in the accompanying algorithm ( Fig. 16.32 ).

- A.
Diagnoses of idiopathic premature pubarche, premature adrenarche, or exaggerated adrenarche, or based on bone age and/or hormonal measurements are provisional diagnoses. Even if these tests are normal or only mildly elevated, clinical follow-up is indicated to rule out inordinate progression of pubarche or evidence of virilization. This is because bone age interpretation and the precision of testosterone assays at low levels are often problematic, and because this algorithm will not detect some very mild hyperandrogenism cases.
- B.
A bone age that is significantly advanced for chronological age is an indication for a screening laboratory workup for childhood hyperandrogenism, consisting of dehydroepiandrosterone sulfate (DHEAS) and testosterone levels. A bone age that exceeds height age indicates less than average height potential. A compromised height potential (indicated by a predicted adult height less than expected for the family or a bone age > 120% of height age in a young child) or DHEAS or testosterone level above the normal adrenarchal range (130 mcg/dL and 35 ng/dL, respectively) suggests that the premature pubarche may be caused by a virilizing disorder rather than ordinary premature adrenarche.
- C.
An 8:00 AM baseline measurement of 17-hyroxyprogesterone (17-OHP) is an option to an adrenocorticotropin hormone (ACTH) test in a family, population, or ethnic group in which the risk is relatively low for nonclassical congenital adrenal hyperplasia caused by 21-hydroxylase deficiency. A level over 170 ng/mL (5.1 nmol/L) has a 95% or greater sensitivity and specificity for this disorder among premature pubarche patients when obtained at 8:00 AM, before the level wanes with diurnal ACTH secretion. A normal baseline level does not necessarily exclude this or more rare forms of nonclassical congenital adrenal hyperplasia.
- D.
For ACTH testing, the typical response in congenital adrenal hyperplasia (CAH) is that the steroid immediately before the enzyme block is extremely elevated, and steroids earlier in the biosynthetic pathway are successively less elevated the further removed they are from the block. For example, in 21-hydroxylase deficiency, 17-OHP is extremely elevated and androstenedione and testosterone are successively less elevated; in 3beta-hydroxysteroid dehydrogenase type 2 deficiency, 17-hydroxypregnenolone and DHEA responses are extremely elevated and 17-hydroxyprogesterone, androstenedione, and testosterone are mild to moderately elevated. An elevated baseline level of 17-OHP may preclude a clear response to the ACTH test. For ACTH testing, an atypical response pattern is one that is not typical for any type of CAH. An example of an atypical response would be baseline or post-ACTH androstenedione or testosterone elevations greater than those of 17-OHP.
- E.
The dexamethasone androgen-suppression test consists of administering dexamethasone, 1 mg/m 2 /day in three to four divided doses daily for 4 days, and then measuring the serum cortisol, DHEAS, and androgens on the morning of the fifth day after a final dexamethasone dose. Normally, serum cortisol falls below 1 mcg/dL (28 nmol/L), testosterone to less than10 ng/dL, and DHEAS to less than 40 mcg/dL. Serum androgen intermediates also fall to prepubertal concentrations.
Constitutional Delay of Growth and Pubertal Development
By statistical definition, delayed puberty occurs in 3% of girls. Most of these girls are otherwise normal, in which case this is termed constitutional delay of growth and pubertal development (CDGP). It is familial; 80% in a large series had a parent with objectively delayed puberty. A family history of delayed puberty is found in 50% to 75% of patients with CDGP. Although its inheritance is likely complex, some predisposing genetic factors seem to have a dominant effect. It has long been recognized that delayed puberty was overrepresented in families with idiopathic hypogonadotropic hypogonadism or hypothalamic amenorrhea cases, and rare variants in genes underlying these conditions appear to contribute to the etiology. Recent molecular investigation revealed that CDGP indeed segregates within families with complex patterns of inheritance that include X-linked, autosomal dominant and recessive and bilineal, although sporadic cases also occur. Autosomal dominant is the most prevalent pattern of inheritance (with or without complete penetrance). Initially, candidate genes associated with CDGP have been identified using GWAS, linkage analysis, and targeted sequencing strategies. However, recently whole exome and genome sequencing are increasingly being used to identify novel candidate genes. Despite these advances, the genetic basis and neuroendocrine pathophysiology remains unknown in the majority of patients with CDGP.
Mutations in several genes have been associated with CDGP. Six unrelated families from a Finnish cohort with delayed puberty were found to have two mutations in immunoglobulin superfamily member 10 (IGSF10) and four other families were found to have two rare variants with unknown significance. Mutations in IGSF10 appear to cause decreased levels of IGSF10 expression during embryogenesis resulting in delayed migration of GnRH neurons from the olfactory bulbs to the hypothalamus. The dysregulation of GnRH neuronal migration results in an abnormal configuration of the GnRH neuronal network and a functional defect in GnRH secretion that becomes crucial when a threshold level of GnRH secretion must be achieved for pubertal onset. IGSF10 mutations were also found in women with a hypothalamic amenorrhea and in patients with congenital hypogonadotropic hypogonadism (CHH), although these mutations did not alone appear sufficient to cause the phenotype. Loss-of-function mutations in IGSF1 have been identified in patients with X-linked central hypothyroidism, delayed pubertal growth, and delayed increase in testosterone levels.
Mutations in genes found in patients with CHH are also found in CDGP. Mutations in heparan sulfate 6-O-sulfotransferase 1 (HS6ST1), fibroblast growth factor receptor 1 (FGFR1), and Klotho β (KLB) have been found in several kindreds with CHH and their relatives with CDGP. Variants in the genes encoding gonadotropin-releasing hormone receptor ( GNRHR ), tachykinin 3 (TAC3) and its receptor (TACR3), interleukin-17 receptor D (IL17RD), and semaphorin 3A (SEMA3A) known to cause CHH have been found in patients with CDGP. Mutations in 24 genes associated with GnRH deficiency were found in probands with CHH with greater frequency than in CDGP, leading to the conclusion that CHH and CDGP are likely to have different genetic backgrounds. Mutations in Kallmann syndrome genes such as anosmin 1 ( ANOS1 ) and N-methyl-D-aspartic acid receptor synaptonuclear signaling and neuronal migration factor (NSMF) have not to date been identified in pedigrees with CDGP. Loss-of-function mutations within the GnRH receptor are the most frequent cause of autosomal recessive CHH, accounting for 16% to 40% of patients. Mutations have been found within the extracellular, transmembrane, and intracellular domains of the receptor leading to impaired GnRH action. A homozygous partial loss-of-function mutation in GNRHR was found in two brothers, one with CDGP and one with idiopathic HH, and a further heterozygous mutation was found in one male with self-limited CDGP. Thus the genetic background of CHH and GDGP may be different, or shared by as yet undiscovered genes.
Girls with constitutional delay are generally more slight in habitus and have lower bone mineral density upon entering puberty than earlier maturing girls. Girls do not usually become concerned about this until they enter high school at 14 years of age and realize that not only has pubertal development not begun, but also most of their friends are menstruating. When puberty does ensue in such subjects it is perfectly normal in tempo. Endocrinological status is normal for the stage of puberty. The differential diagnosis includes chronic endocrine, metabolic, and systemic disease of almost any kind, as well as gonadotropin deficiency, which it closely resembles and from which it is distinguished with difficulty (see Gonadotropin Deficiency and Functional Hypothalamic Anovulation).
Physiological Adolescent Anovulation
Immaturity of the hypothalamic-pituitary-ovarian axis causes menstrual cycles to be longer and more irregular during the early postmenarcheal years ( Fig. 16.33 ). About half of menstrual cycles during the first 2 years after menarche are anovulatory by standard criteria; half of these actually have evidence of the attenuated ovulation that results in luteal insufficiency (see Luteal Phase Defects). Although about half of these cycles with ovulatory abnormalities are irregular, half are of normal length by adult standard, so normal adolescent menstrual cyclicity differs only slightly from that of reproductive-age adults. Thus most adolescent ovulatory abnormalities are asymptomatic, with cyclic menstrual bleeding occurring at 21- to 45-day intervals even in the first postmenarcheal year ( Fig. 16.34 ): this paradox arises because immature cyclic ovarian function is usually occurring during these intervals. Serum hormonal changes during normal adolescent menstrual cycles confirm that substantial but immature cyclic follicular development occurs in such girls and some aluteal adolescents ( Fig. 16.35 ).

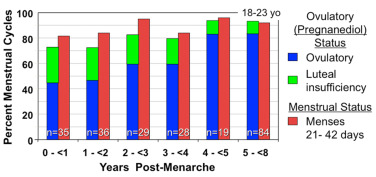
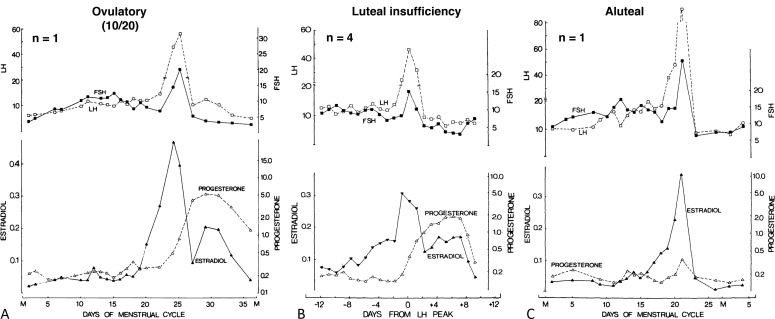
Although there is considerable variation in the time it takes for menstrual cycles to mature, menstrual regularity approximates adult standards in most girls within a year of menarche: three-quarters have a cycle length between 21 and 45 days, and 5% more fall within these bounds each of the next 3 years. By 5 gynecological years, 90% of menstrual cycles last 22 to 40 days, and about 80% of cycles are normal ovulatory ones. Menstrual cycle length narrows to 24 to 38 days in midadulthood as ovulatory rates approach 90%.
Menstrual abnormalities in adolescence can be defined similarly to abnormal uterine bleeding in adults. Primary amenorrhea is failure to begin menses at a normal age (by 15 years of age or within 3 years of thelarche when it has been delayed). Secondary amenorrhea is the absence of menstrual periods for 90 days or more after initially menstruating. Oligomenorrhea is defined as subnormal menstrual frequency, the normal limits for which gradually change during the 6 years after menarche ( Box 16.2 ). Anovulatory cycles may also cause excessive uterine bleeding, as discussed in the later section Ovulatory Dysfunction: Dysfunctional Uterine Bleeding.
Descriptor | Definition |
---|---|
Primary amenorrhea | Lack of menarche by 15 years of age or by 3 years after the onset of breast development a |
Secondary amenorrhea | Over 90 days without a menstrual period after initially menstruating (in consecutive periods during first year post-menarche) |
Oligomenorrhea (infrequent abnormal uterine bleeding) | Postmenarcheal year 1: average cycle length > 90 day (fewer than four periods in the year) |
Postmenarcheal year 2: average cycle length > 60 days (fewer than six periods in the year) | |
Postmenarcheal years 3: average cycle length > 45 days (fewer than eight periods per year) | |
Postmenarcheal years ≥ 4: cycle length > 38 days (< 9 periods per year) | |
Excessive uterine bleeding b | Menstrual bleeding that occurs more frequently than every 21 days (19 days in year 1) or is prolonged (lasts > 7 days) or heavy (soaks more than one pad or tampon every 1–2 h large clots, or gushing) |
a Bone age of 15 years may be substituted for chronological age in girls with earlier than average age at puberty onset.
b Encompasses frequent, intermenstrual, heavy, and/or prolonged abnormal uterine bleeding. Alternatively termed dysfunctional uterine bleeding or excessive abnormal uterine bleeding caused by ovulatory dysfunction .
Symptomatic menstrual abnormalities in adolescents are increasingly unlikely to represent “physiological” adolescent anovulation with time. By 1year postmenarche, failure to establish and sustain a normal adult menstrual pattern carries approximately a 50% risk of persistent oligoovulation, and failure to do so by 2 years after menarche carries approximately a two-thirds risk ( Fig. 16.36 ). Thus persistence of menstrual irregularity for 1 to 2 years or more is a strong indication for investigation (see section Abnormal Puberty).
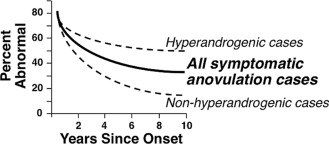
Serum LH, testosterone, and androstenedione levels are significantly higher in adolescents with anovulatory than those with ovulatory cycles. It is unclear whether this is the cause or the result of the anovulation; however, if hyperandrogenemia is found, it seldom regresses. A polycystic ovary is common in adolescents and is usually a variant of normal, unless associated with menstrual abnormality or hyperandrogenism.
Other Normal Adolescent Variations
Three-quarters of adolescent girls experience mild to severe comedonal acne, and one-quarter mild inflammatory acne. Mild hirsutism also arises commonly among perimenarcheal girls. However, inflammatory acne that is moderate or severe (i.e., > 10 lesions of face or other region) is uncommon during the perimenarcheal years, and hyperandrogenism should be considered in such girls as it should in those with mild hirsutism and menstrual irregularity (see Hyperandrogenism in Adolescence). The initiation of acne is more closely related to blood levels of DHEAS than of other androgens, as is cystic acne.
Profound psychological changes occur during adolescence. Sexually immature girls tend to be socially immature, and the onset of puberty is associated with increased independence and profound changes in outlook on life and intellectual capacities. The extent to which these developments occur in reaction to the physical changes of puberty and the extent to which they are direct effects of sex hormones are unknown. Masculine tomboyish traits usually have no clear hormonal basis, although there is some evidence that they may have prenatal hormonal determinants. Social interactions have effects on these aspects of development. They affect even the synchrony of the menstrual cycle.
Despite the popular notion that adolescence is inherently a period of turmoil, the majority of teenagers do not develop significant social, emotional, or behavioral difficulties. Occasional experimentation and risk-taking are normal, as are withdrawal from and conflict with parents. Adolescent behavior must be understood in the context of individual susceptibility, family upbringing and interactions, peer group interactions, changes in brain maturation, and adolescents’ reaction to their perception of the bodily changes and to the sexual urges that are the direct consequences of puberty. Simply because a problem is displayed during adolescence does not mean that it is a direct consequence of puberty.
Many behavioral problems that emerge during adolescence have earlier roots. Although the prevalence of depression increases during puberty, many children who develop depression during adolescence have had preexisting symptoms of psychological distress. Likewise, most delinquent teenagers have had antecedent problems at home and school.
Early-maturing girls in Western cultures are more popular, but they have more emotional problems; lower self-image; and higher rates of depression, anxiety, and disordered eating than their peers. Early maturation appears particularly to be a risk factor for problem behavior among girls who have had a history of difficulties before adolescence, when they have more opposite sex friendships and relationships, and when they attend coeducational schools.
Short-term administration of testosterone or estrogen has minimal effects on behavior or mood in adolescents. Thus variation in hormone levels accounts for only a small fraction of adolescents’ affective issues, and social influences account for considerably more. Although there is little evidence that psychological difficulties stem directly from hormonal changes during normal puberty, it is likely that the bodily changes of adolescence play a role in the development of a negative body image when they occur out of synchrony with sociocultural norms.
Problems with initiating and maintaining sleep are common in adolescents and contribute a small amount to poor school performance. Although insufficient sleep might be caused by environmental factors (e.g., social and academic pressures), intrinsic factors clearly play an important role. A 50% decline in the intensity of deep (slow wave, delta) sleep occurs during adolescence, and one-half of this change occurs between 12 and 14 years of age. Recent evidence indicates that this change is related to age and sex, beginning earlier in girls, but not to pubertal stage. It has been proposed that this shift is a manifestation of the widespread synaptic pruning that is related to the emergence of adult cognitive capacity.
The causal direction of the link between pubertal development and the quality of family relationships has come into question. Several studies have indicated that family dynamics may affect the timing and course of puberty, with earlier and faster maturation observed among adolescents raised in homes characterized by more conflict and among girls from homes in which the biological father is not present.
Abnormal puberty
Abnormal Development
Disorders of Sex Development
Patients with DSD (formerly termed intersex )—those whose genitalia are ambiguous or inappropriate for their gonadal sex as a result of endocrinopathy—may come to a physician’s attention for the first time at puberty. These syndromes have been categorized as 46, XX DSD, which encompasses cases formerly termed female pseudohermaphroditism and including 46, XX testicular DSD (formerly termed XX sex reversal ); 46, XY DSD, which encompasses cases formerly termed male pseudohermaphroditism and including 46, XY complete gonadal dysgenesis (XY sex reversal, Swyer syndrome); and sex chromosome DSD, which includes Turner syndrome, Klinefelter syndrome, mixed gonadal dysgenesis, and chimeric ovotesticular DSD. In the absence of chromosomal mosaicism, ovotesticular DSD, formerly termed true hermaphroditism , is categorized as either 46, XX DSD or 46, XY DSD. Patients with any of these disorders may undergo inappropriate puberty. They may present with clitoromegaly and be found upon examination to have a degree of genital ambiguity which was previously overlooked. Virilization beginning at puberty is sometimes the presenting complaint. Ovotesticular DSD or 46, XX DSD because of CAH are compatible with fertility. Androgen insensitivity syndrome in a genetic male may present as primary amenorrhea in an otherwise phenotypically normal adolescent girl. The disorders of sexual differentiation are reviewed in detail in Chapter 6 .
Congenital virilization of the female developmentally programs the emergence of PCOS at puberty. These observations are consistent with studies of fetal androgenization of the female in several species, including primates. There is a persistent increase in LH pulse frequency and impairment of the negative feedback effect of progesterone on LH release that appear to be related to suppression of hypothalamic PR in response to estradiol. Experimental animals exposed to androgen excess early in gestation develop classic PCOS features: these animals have elevated LH levels, ovarian and adrenal hyperandrogenism, oligomenorrhea, and polyfollicular ovaries. They also have abdominal obesity, insulin resistance, impaired glucose tolerance, and dyslipidemia, which likewise appear to result from developmental programming.
Genetic males with complete androgen insensitivity have low LH levels and poor LH responsiveness to GnRH in the neonatal period. However, gonadotropin levels are normal-to-high at puberty, and, paradoxically, androgen signaling via the androgren receptor enhances the capacity of females to mount an LH surge in response to estrogen positive feedback.
Other Dysgenetic Disorders
Failure of the onset of menses can result from structural abnormalities of the genital tract that do not have an endocrinological basis. The hymen may be imperforate, which results in hydrocolpos if the vagina is intact. The vagina may be aplastic, which will result in hydrometrocolpos if the uterus is intact. The uterus may be congenitally aplastic. Uterine synechiae develop as the consequence of endometritis, which may result from infection or irradiation (Asherman syndrome). Congenital absence of the vagina may be associated with varying degrees of uterine aplasia; this is the Mayer-Rokitansky-Kuster-Hauser syndrome. This syndrome seems to occur as a single gene defect or as an acquired teratogenic event involving mesodermal development and the mesonephric kidney, the latter resulting in abnormalities of the genital tract and sometimes the urinary tract. A subtype caused by Wnt4 gene defects is associated with hyperandrogenism.
Precocious Puberty
Causes
When breast or sexual pubic hair development begins before the age of 8.0 years or menses begin before the age of 9.5 years, puberty is traditionally considered precocious, or premature. It should be kept in mind that breast development during the seventh year is within normal limits in ethnic minority girls. In addition, presexual pubic hair (stage 2) may be normal in 6- and 7-year-old ethnic minority girls.
Puberty can occur prematurely as an extreme variation of normal, because of a disturbance in the HPG axis normally involved in sexual maturation, or because of a disturbance outside the HPG axis. Depending on which part of this hormonal axis is involved, different forms of precocious puberty are distinguished. A classification of the causes of premature puberty together with typical findings is given in Table 16.4 .
Locus | Type | HA a | BA a | Estrogens Ü | Androgens Ü | LH/FSH Ü | Pathology | Characteristics |
---|---|---|---|---|---|---|---|---|
Complete precocity (Central, Gonadotropin-Dependent) | ||||||||
Hypothalamic | Isosexual | + | ++ | + | + | + | Idiopathic Neurogenic Advanced somatic maturation | 95% of female cases |
Incomplete precocity (Peripheral, Gonadotropin-Independent) | ||||||||
Normal variant | Isosexual | – | − | ± | − | ± | None | Thelarche |
Isosexual | − | − | − | ± | − | None | Pubarche/adrenarche | |
Neuroendocrine | Contrasexual | + | ++ | − | + | +/+++ | LH/hCG excess | Familial or tumor |
Isosexual | Low | Low | − | − | ± | Hypothyroid | Growth arrest | |
Ovary | Isosexual | + | ++ | +/+++ | − | − | McCune-Albright | Bone lesions ± nevi ± ovarian cysts |
Isosexual/contrasexual | + | ++ | +/+++ | +/+++ | _ | Tumor | ||
Adrenal | Contrasexual | + | ++ | ± | +++ | − | Congenital adrenal hyperplasia | Dexamethasone suppressible |
Contrasexual/isosexual | + | ++ | +/+++ | +/+++ | − | Tumor | ||
Ectopic | Isosexual | + | ++ | +/+++ | − | − | Aromatase excess | |
Exogenous | Contrasexual/isosexual | ± | ± | − | − | − | Sex steroid exposure | |
End organ | Isosexual/contrasexual | − | − | − | − | = | Vaginal foreign body, abuse, tumor |
a HA (height for age) and BA (bone age); − normal; + advanced; ++ markedly advanced.
It is important to distinguish between true precocious puberty and pseudoprecocious puberty. True precocious puberty is gonadotropin dependent; thus central is another term applied to this type of precocity. Maturation is complete: both breasts and pubic hair develop as the result of CNS activating pituitary secretion of the respective gonadotropins FSH and LH (although breast development may be the sole manifestation of early complete precocity for as long as 6–12 months). Patients with true precocious puberty have “isosexual” precocity because the secondary sexual characteristics are appropriate for the sex of the child. Pseudoprecocious puberty is gonadotropin independent; it is not mediated by pubertal pituitary gonadotropin secretion and is sometimes termed peripheral . Maturation is incomplete, with only one type of secondary sexual characteristic developing early. Peripheral precocity has diverse causes. In some patients with pseudoprecocity, pubertal development is isosexual, in others it is “contrasexual,” meaning that characteristics of the opposite sex are manifested.
Complete Precocious Puberty
True isosexual precocity results from pubertal function of the HPG axis. About 95% of true precocity in girls is idiopathic. Idiopathic true sexual precocity appears to be caused by premature triggering of the normal pubertal mechanism. Pubertal development usually is qualitatively and quantitatively normal except for its early occurrence. The predominance of the idiopathic cases in females and its benign nature are compatible with the likelihood that this disorder is an extreme exaggeration of the normal tendency of girls to have higher gonadotropin levels than boys. Most cases are sporadic, a few familial. The majority of these patients seem to go on to have normal menstrual cycles and fertility. Indeed, pregnancy has been documented to occur as early as 4 years of age.
Rapidly progressive puberty with a growth spurt ensues when activation of the pituitary-ovarian axis is sustained. However, precocious puberty is not necessarily sufficiently intense or sustained to cause inexorable progression or bring about deterioration of height potential. Precocity in the 6- to 8-year age range usually is not rapidly progressive and most commonly seems to be caused by excessive adiposity.
Any type of intracranial disturbance can cause true isosexual precocity. These neurogenic disturbances are presumed to cause true sexual precocity by increasing the prevalence of excitatory inputs or by interfering with CNS inhibition of hypothalamic GnRH secretion. These include congenital brain dysfunction, such as cerebral palsy or hydrocephalus, or acquired disorders, such as irradiation, trauma, chronic inflammatory disorders, or masses in the region of the hypothalamus. The activation of GnRH release by hypothalamic injury may be mediated by TGF-β, trauma, chronic inflammatory disorders, or masses in the region of the hypothalamus. An empty sella is occasionally found. The precocity of neurofibromatosis type I (von Recklinghausen disease) usually results from an optic glioma, which is often of low-grade malignancy, or from a hamartoma, although occasionally with neither. Hamartoma of the hypothalamus may cause central sexual precocity; this effect is most likely caused by an anatomic effect on hypothalamic structures rather than by acting as an “accessory hypothalamus” that releases pulses of GnRH into the pituitary portal circulation. Fig. 16.37 shows a hypothalamic hamartoma.
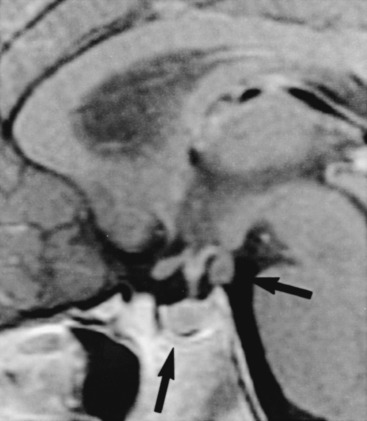
A small proportion of pineal tumors cause true sexual precocity. The incidence of sexual precocity is about 3.5 times as great in nonparenchymatous neoplasms (such as gliomas and teratomas) as in parenchymatous pineal tumors. This suggests that these tumors cause sexual precocity via absence of a normal pineal inhibitory factor rather than by destructive effects on inhibitory tracts. Although pineal masses may cause paralysis of upward gaze by pressure on the corpora quadrigemina, this sign is present in only a minority of cases.
Pineal or hypothalamic hCG-secreting germ cell tumors occasionally cause true sexual precocity. Because hCG is an LH receptor agonist, possible explanations for this unusual situation are disinhibition of hypothalamic GnRH release attributed to a mass effect of the neoplasm, the weak FSH effect of massive elevation of hCG, or the capacity of some dysgerminomas to secrete estradiol, as well as hCG.
Advancement of somatic maturation caused by peripheral endocrine disorders that advance the bone age to a pubertal level sometimes cause true sexual precocity. Thus true puberty may begin after correction of virilizing or feminizing disorders that have advanced the bone age to 10 to 12 years.
The hypergonadotropinism of premature ovarian insufficiency (POI) has been reported to cause sexual precocity or rapid progression of puberty before premature ovarian failure. Although PCOS has occasionally been reported to follow true sexual precocity, there is currently no clear evidence of a significant association.
A family history of complete precocious puberty (CPP) is common in patients with early puberty; however, a clear genetic etiology is usually unknown. Monogenic causes of CPP have recently been identified in four genes. Gain-of-function mutations have been found in kisspeptin and its receptor, KISS1R. The patients were heterozygous for the mutations, with an autosomal dominant inheritance. The first mutation to be described was in an adopted girl who had progressive thelarche from birth, with accelerated growth velocity and skeletal maturation noted at age 7 years. A heterozygous activating mutation of KISS1R (p. Arg386Pro) was identified and in vitro studies showed that the mutation led to prolonged activation of intracellular signaling pathways resulting in higher sustained inositol phosphate accumulation. A mutation in kisspeptin, p. Pro74Ser, was identified in a boy with CPP at age 1 year, with high concentrations of serum LH and testosterone. Interestingly, his mother and maternal grandmother had normal pubertal development, carried the p. Pro74Ser mutation in the heterozygous state, suggesting incomplete penetrance. In vitro, the mutated protein was able to stimulate signal transduction to a greater extent than the wild type, suggesting it may be more resistant to degradation, resulting in greater kisspeptin bioavailability. However, large-scale studies in children with CPP have not been able to identify additional patients and families with CPP with these mutations, hence kisspeptin and KISS1R activating mutations are relatively rare causes of CPP.
Another genetic abnormality linked to CPP involves loss-of-function mutations in the Makorin RING-finger protein 3 ( MKRN3 ) gene, a maternally imprinted gene located on the long arm of chromosome 15 (Prader-Willi region) encoding a protein that is involved in gene transcription and ubiquitination. Expression of MKRN3 was found to be high in the arcuate nucleus in prepubertal mice, decreases before puberty, and is low after puberty, thus MKRN3 appears to be acting as an inhibitor of puberty. Interestingly, MKRN3 polyubiquinylates Nptx1, another protein of unclear function that is also expressed at low levels prepubertally, and highly during puberty. Fifteen families with a history of CPP were studied, and mutations in MKRN3 that resulted in truncated proteins or missense mutations predicted to disrupt protein function were found in one-third of them. At present, 10 different mutations have been found, and include frameshift, missense, or nonsense mutations predicted to result in loss-of-function of the protein. MKRN3 is maternally methylated, explaining the autosomal dominant paternal inheritance in all cases, with no de novo mutations described. All patients described to date exhibit a typical pattern of early pubertal development. A few patients with MKRN3 mutations were described as having syndromic features that included esotropia, a high-arched palate, dental abnormalities, clinodactyly, and hyperlordosis. In a genetic study of 20 boys with idiopathic CPP, eight were found to have MKRN3 mutations and one had a KISS1 -activating mutation, perhaps indicating that MKRN3 mutations are a relatively frequent cause of CPP.
Delta-like homolog 1 ( DLK1 ), a paternally expressed imprinted gene, encodes a protein expressed in kisspeptin-expressing neurons. DLK1 is a part of the delta-notch pathway, which is an evolutionarily conserved signaling pathway with roles in proliferation and differentiation during development. In the pituitary, DLK1 and notch signaling appear to be important in pituitary cell type differentiation. A mutation in DLK1 was found in a family with five girls with CPP. The mutation was a 14-kb deletion along with a 269-bp duplication. Serum levels of DLK1 were undetectable in these girls. In another study, the DLK1 gene was sequenced in 60 girls with idiopathic CPP, and no mutations were found.
Distinct chromosomal abnormalities associated with specific syndromes may include CPP. These include: 1p36 deletion, 7q11.23 microdeletion (Williams-Beuren syndrome), 9p deletion, maternal uniparental disomy of chromosomes 7 (Silver-Russell syndrome) and 14 (Temple syndrome), inversion duplication of chromosome 15, de novo interstitial deletion and maternal uniparental disomy of chromosome 15 (Prader-Willi syndrome), and a de novo deletion in the cyclin-dependent kinase-like 5 gene ( CDKL5 ; located in the Xp22 region) (phenotype similar to Rett syndrome).
Incomplete Precocity
The most common causes of incomplete sexual precocity in girls are the extreme variants of normal mentioned previously, premature thelarche and premature pubarche. These are incomplete forms of sexual precocity in which either breast development (thelarche) or sexual hair development (pubarche) is of a degree appropriate for an early stage of puberty and isosexual. Isolated prepubertal menses is a rare disorder that has been attributed to transient ovarian activity.
LH- or hCG-producing tumors have not been reported to virilize girls, perhaps because of their limited capacity for thecal androgen production over short periods of time. However, familial isolated elevation of LH has been reported to cause mild virilization of siblings: one was a girl who developed premature pubarche and clitoral hypertrophy at 4 years of age, with slight to moderate advances in height and bone age in association with an adrenarchal level of DHEAS and a moderately elevated testosterone level (91 ng/dL).
The van Wyk-Grumbach syndrome is one of the most puzzling pediatric complexes. This is an unusual syndrome of sexual precocity associated with juvenile hypothyroidism. A case is illustrated in Fig. 16.38 . This syndrome is often characterized by galactorrhea, which is often not spontaneous; a few drops of milky fluid may become apparent only upon “milking” the subareolar ductal tissue. Multicystic ovaries are often demonstrable by ultrasonography. Modern assays show that levels of LH at baseline and post-GnRH are suppressed and those of FSH are early pubertal. There is little, if any, sexual hair development. There is another clinically unique feature about the sexual precocity of hypothyroidism: it is the only form of sexual precocity in which growth is arrested rather than stimulated and is an exception to the general rule, indeed followed by most chronically hypothyroid children, that a delayed growth pattern is associated with delayed puberty.
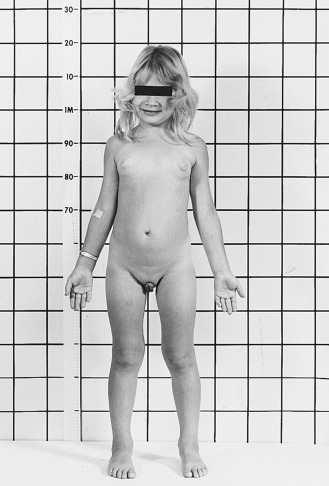
Van Wyk and Grumbach postulated that this syndrome resulted from hormonal “overlap” in the negative feedback regulation of pituitary hormone secretion, with overproduction of gonadotropins, as well as TSH in response to the thyroid deficiency. The specific nature of hormonal overlap has been considerably clarified in recent years, but the pathogenesis of the precocity remains unclear. The increases in serum TSH and prolactin that characterize the syndrome could well be accounted for by common neurohumoral control systems, TRH stimulating and dopamine inhibiting both hormones. It has been suggested instead that the ovarian FSH receptor is activated by the weak intrinsic FSH activity of extreme TSH elevation, analogous to the rare ovarian hyperstimulation syndrome in which pregnancy levels of hCG activate the FSH receptor.
Prolactin excess has been postulated to underlie the FSH-predominant gonadotropin pattern by slowing GnRH pulsations, and it is this FSH stimulation of the TSH-sensitized ovary that seems to be the proximate cause of the sexual precocity. Hyperprolactinemia alone does not correspond with pubertal development in normal or hypothyroid children. However, hyperprolactinemia may itself also sensitize the ovaries to gonadotropins. Induced hyperprolactinemia causes sexual precocity in female rats. Ovarian estrogen and progesterone responsiveness to hCG is increased by prolactin, possibly by its induction of ovarian LH receptors. Conversely, suppression of hyperprolactinemia in experimental hypothyroidism blocks the ovarian cyst formation characteristic of hypothyroidism.
McCune-Albright syndrome is another intriguing disorder causing incomplete isosexual feminization. This is a syndrome of precocious puberty, cafe-au-lait pigmentation occurring in nevi that have an irregular (“coast of Maine”) border, and polyostotic fibrous dysplasia. The disorder is caused by a somatic activating mutation of the G s -alpha subunit protein that couples transmembrane receptors to adenylate cyclase. The syndrome has been recognized predominantly in females and occurs in incomplete, as well as expanded forms. Precocious puberty or monoostotic bone lesions may occur in the absence of cutaneous pigmentation; not all patients have sexual precocity. The sexual precocity is of the gonadotropin-independent type. Luteinized follicular cysts within the ovaries function autonomously. Pituitary adenomas capable of secreting excess LH, FSH, GH, and/or prolactin have been reported. Patients may have Cushing syndrome and hyperthyroidism because of autonomous multinodular hyperplasia. These girls may be at increased risk of breast carcinoma. Nonendocrine abnormalities include cardiopulmonary disease, hypertension, and hepatobiliary disease (including severe neonatal cholestasis). Molecular studies have shown an R201H mutation in over 90% of cases where an affected tissue could be studied, but in only 50% of blood samples. Because of the variation in the number and degree of tissue involvement in individual patients, caused in large part by the extent of mosaicism present, precocious puberty may be the only feature present in an individual who is mosaic for the activating mutation of G s -alpha. Thus these mutations have been found in blood samples from 25% to 33% of subjects with isolated gonadotropin-independent precocity or exaggerated thelarche.
CAH is a well-known cause of premature pubarche. Either nonclassic CAH, a form of the disorder which is so mild that there is no genital defect in girls, or poor control of classic CAH may be responsible. Each form on occasion has been reported to mimic true sex precocity.
Tumor may cause isosexual or contrasexual development. The most common tumor is the feminizing benign ovarian follicular cyst. Most are isolated and large (> 1.0 cm in diameter). The cells lining these cysts are often luteinized. Estrogen levels may be markedly elevated. Testosterone levels tend to be in the adult female range (about 40 ng/dL). Many function intermittently. They may be gonadotropin dependent and respond to GnRH agonist or progestin therapy. A case is illustrated in Fig. 16.39 . The second most common hormonally active ovarian neoplasm in girls is the juvenile granulosa cell tumor. These have variable degrees of ovarian sex cord-stromal elements and are usually localized and benign in spite of having a malignant histological appearance. They are more commonly feminizing than masculinizing in young children. They may produce hCG, AMH, and inhibin. Elevation of hCG is found in many ovarian dysgerminomas (a primitive germ cell tumor), with hypercalcemia in some, although less frequently than in small cell carcinoma. Granulosa-theca cell tumor is occasionally associated with mesodermal dysplasia syndromes. It has been reported in the adrenal gland, presumably arising in an ovarian rest. FOXL2 mutations are typical of adult-type granulosa cell tumors, but are found in only 10% of the juvenile type. A related feminizing ovarian sex cord-stromal tumor may be caused by loss of a tumor-suppressor gene, as in Peutz-Jeghers syndrome. Adult-type ovarian carcinoma of epithelial cell origin is rare. Ovarian masculinizing tumors are discussed in the section Hyperandrogenism in Adolescence.
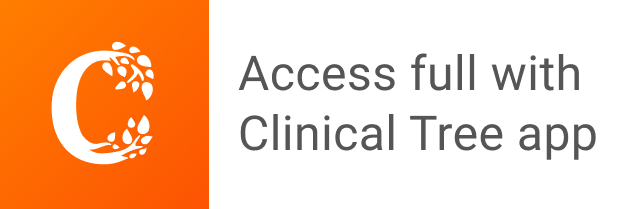