Physics and Biology of Brachytherapy
Brachytherapy (BT) (brachy is Greek for “short distance”) consists of placing sealed radioactive sources very close to or in contact with the target tissue. Because the absorbed dose falls off rapidly with increasing distance from the sources, high doses may be delivered safely to a localized target region over a short time. This chapter reviews the properties and applications of commonly used sealed radionuclides and sources; the basic biologic principles governing clinical response to BT; methods of dose calculation and source-strength specification; and principles of implant design and dose specification for interstitial and intracavitary BT.
BASIC TERMINOLOGY
Implantation techniques may be classified in terms of surgical approach to the target volume (interstitial, intracavitary, transluminal, or mold techniques); the means of controlling the dose delivered (temporary or permanent implants); the source loading technology (preloaded, manually afterloaded, or remotely afterloaded); and the dose rate (low, medium, or high).
Intracavitary insertion consists of positioning applicators (bearing the radioactive sources) into a body cavity in close proximity to the target tissue. Intracavitary BT is used most widely for treatment of localized gynecologic malignancies. All intracavitary implants are temporary implants; they are left in the patient for a specified time to deliver the prescribed dose. With a few exceptions, during temporary implantation, the patient must be confined to a controlled, if not shielded, area in the hospital to manage the radiation safety hazard posed by the large ambient exposure rates around the implant.
Interstitial brachytherapy consists of surgically implanting small radioactive sources directly into the target tissues. A permanent interstitial implant remains in place indefinitely and is not removable; the initial source strength is chosen so that the prescribed dose is fully delivered only when the implanted radioactivity has decayed to a negligible level.
Surface-dose applications (sometimes called plesiocurie therapy or mold therapy) consist of an applicator containing an array of radioactive sources, usually designed to deliver a uniform dose distribution, that is placed on the skin or mucosal surface immediately adjacent to the target tissue.
Transluminal brachytherapy consists of inserting a single line source into a body lumen to treat its surface and adjacent tissues.
Until the early 1960s, radioactive sources (needles for interstitial therapy or preloaded applicators for intracavitary therapy) were implanted directly into the patient. Radiation exposure to the brachytherapist and operating room staff was reduced significantly with the advent of afterloading technology.1,2 Manual afterloading consists of implanting nonradioactive tubes or intracavitary applicators into the patient. Following transport of the patient to his or her room, sources are manipulated into the applicators by means of forceps and other handheld tools. Exposure to staff responsible for source loading and the care of BT patients can be greatly reduced or eliminated by use of a remote afterloading system, which consists of a pneumatically driven or motor-driven source transport system for robotically transferring radioactive material between a shielded safe and each treatment applicator.
According to Report No. 38 of the International Commission on Radiation Units and Measurements (ICRU),3 low–dose-rate (LDR) implants deliver doses at the rate of 40 to 200 cGy/hour (0.4 to 2 Gy/hour), requiring treatment times of 24 to 144 hours, during which the patient is confined to an inpatient treatment room. At the other extreme, high–dose-rate (HDR) BT uses dose rates in excess of 0.2 Gy/minute (12 Gy/hour). In fact, modern HDR remote afterloaders deliver instantaneous dose rates as high as 0.12 Gy/second (430 Gy/hour) at a distance of 1 cm, resulting in treatment times of a few minutes. Such treatments must be delivered in heavily shielded vaults using remote afterloading devices, but allow fractionated BT to be delivered on an outpatient basis. Medium dose-rate delivery, defined as the 2- to 12-Gy/hour range, rarely is used. Although not recognized by ICRU Report No. 38, the ultra-low–dose-rate range (0.01 to 0.3 Gy/hour) is of great importance; it is the dose-rate domain used in permanent implants with 125I and 103Pd seeds.
TABLE 22.1 PHYSICAL PROPERTIES AND USES OF BRACHYTHERAPY RADIONUCLIDES
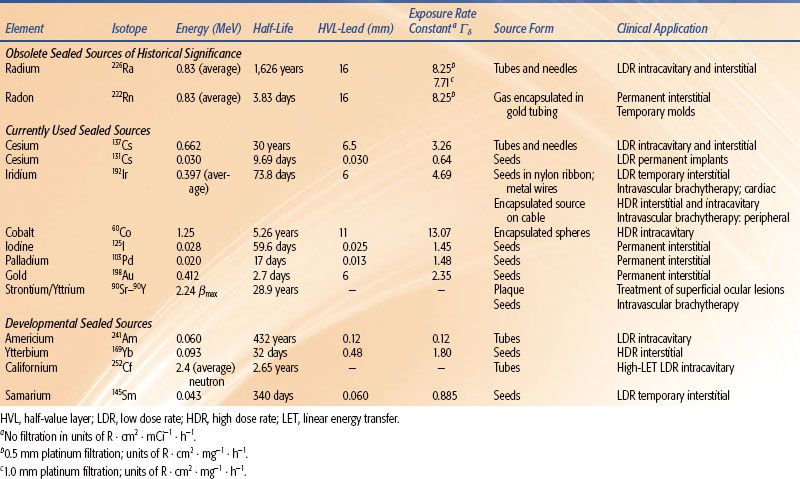
PROPERTIES OF BRACHYTHERAPY SOURCES AND RADIONUCLIDES
The clinical utility of any radionuclide depends on physical properties such as half-life, radiation output per unit activity, specific activity (Ci/g), and photon energy. In addition, the methods of producing the radionuclide and its physical or chemical form strongly influence cost-effectiveness, safety, and toxicity. Detailed properties of BT radionuclides are listed in Table 22.1.
Photon Spectrum and Dosimetric Characteristics of Brachytherapy Sources
The dose delivered with a BT procedure depends on the individual source strengths, source arrangement, and implant duration, tissue composition, as well as the dosimetric characteristics of the implanted sources. These dosimetric characteristics are described by specifying the distribution of dose rates per unit strength about the source, often in terms of an “away-and-along” table4 in Cartesian coordinates or in terms of the Task Group 43 protocol5 described later in this chapter. The single-source dose distribution is of central importance to treatment planning because commercial computer planning systems estimate dose distribution from the spatial coordinates of the implanted sources using the principle of superposition. The source-superposition algorithm estimates the contribution of each source, given its tip-and-end coordinates and the single-source dose-rate array, to each point of interest. These contribution estimates are summed to estimate the total dose rate at each point. Often, total dose rates are calculated over a two-dimensional (2D) grid of points and are represented as isodose-rate curves.
For conventional BT, for which the therapeutically relevant distance range is 3 to 20 mm, only photons (γ-rays or characteristic x-rays) with energies in excess of 15 keV (kiloelectron volts) contribute to the therapeutic effect. In general, four factors influence the single-source dose distribution for photon-emitting sources: (a) distance (inverse-square law), (b) absorption and scattering in the source core and encapsulation, (c) photon attenuation, and (d) scattering in the surrounding medium (Fig. 22.1). Encapsulation prevents radioactive material from leaking out of the source and absorbs nonpenetrating radiation (β-rays, α-rays, and low-energy photons), which would otherwise give rise to high surface doses while contributing nothing to the therapeutic effect.
FIGURE 22.1. Typical cylindrical brachytherapy source, consisting of an active core (inner cylinder within which radioactivity is uniformly distributed) and the surrounding encapsulation (usually stainless steel or titanium for modern sources). The four principal factors influencing the relative dose distribution include (1) distance, (2) attenuation and scattering in source structure, (3) attenuation by surrounding medium, and (4) accumulation of scattering in surrounding medium.
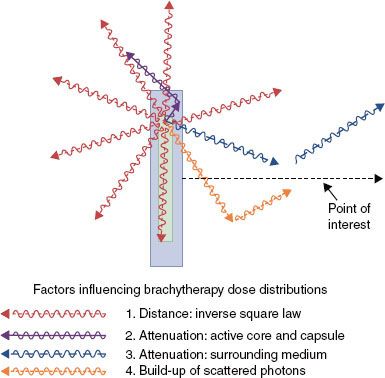
FIGURE 22.2. An isotropic point source of activity, A. To illustrate the derivation of inverse-square law, the source is surrounded by vacuum and placed at the center of two concentric spherical surfaces of radii r1 and r2. By definition, an isotropic point source has no extension and radiates photons with equal likelihood in all directions in straight-line paths.
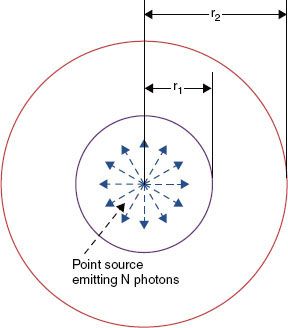
Each voxel of radioactive core material shown in Figure 22.1 can be assumed to be an isotropic point source (Fig. 22.2). Because of the straight-line emission of photons with equal likelihood in all directions, photon intensity or fluence, (r), at any point is proportional to the inverse square of its distance, r:
assuming that attenuation and scattering can be neglected.
As a result of this purely geometric effect, the absorbed doses D(r1) and D(r2) at the two distances r1 and r2 (Fig. 22.2) are related by:
This fundamental law applies exactly to each point of the radioactive core of the source shown in Figure 22.1 assuming that there is no attenuation and scattering of photons by the surrounding medium. However, Eq. (2) will not accurately describe the “collective” dose fall-off arising from the combined action of the point sources distributed throughout the core, unless both r1 and r2 are large relative to the active source dimensions. Of the four factors influencing the dose distribution (Fig. 22.1), inverse-square law is by far the most important. For a pure isotropic point source, dose will decrease by a factor of 100 between the distances of 0.5 and 5 cm. The influence of the remaining factors over the same distance range rarely exceeds a factor of 2 or 3. Consequently, most of the clinical characteristics of implants (e.g., the heterogeneous dose distribution within the target tissue and rapid fall-off of dose outside the implanted volume) can be accounted for by applying inverse-square law to each pointlike element of radioactivity within the implant. Control of intersource spacing and positioning relative to the target and dose-limiting tissues is the most challenging issue in delivering BT.
Although inverse-square law dominates the dose distribution, the surrounding medium and the source structure do significantly affect the dose distribution (Fig. 22.1). The source core and surrounding capsule reduce dose at the point of interest through absorption and scattering of primary photons. Primary photons contributing dose to points located near the longitudinal source axis (cylindrical axis of the source or axis of rotation) must traverse longer path lengths of capsule and core material and therefore experience more attenuation than photons contributing dose to equidistant points on the transverse source axis (plane perpendicular to the longitudinal source axis that bisects its active core). At a fixed distance from the source center, the dose near the longitudinal axis is usually smaller than on the transverse axis. This phenomenon is known as oblique filtration and is the main cause of dose anisotropy (variation of dose as a function of polar angle at each fixed distance relative to the source center) characteristic of extended BT sources. Because BT sources are cylindrically symmetric, the dose distribution will be equatorially isotropic (constancy of dose as a function of azimuthal angle for each fixed polar angle and distance).
The tissue-equivalent medium surrounding the source affects the dose distribution in two important and competing ways (factors (3) and (4) of Fig. 22.1). At each point of interest, the intervening medium reduces the dose distribution by attenuating primary photons (deflecting them from their straight-line trajectories). At the same time, photons are being emitted in all directions from the source and interacting with the medium by means of Compton scattering and photoelectric absorption. Thus, each volume element of tissue is effectively radiating scattered photons in all directions, many of which contribute to dose at the point of interest. This mechanism, known as scattered-photon buildup, enhances the dose. The overall influence of the surrounding medium is the combined effect of these two competing processes: photon attenuation and scattered-photon buildup. In contrast to external-beam therapy, in which the scattering volume is limited to a narrow cone, scattered photons dominate BT dose distributions at distances >2 cm. Photon scattering is the main source of complexity in BT dose measurement and algorithm development.
Figure 22.3 demonstrates that the relative dose versus distance from the source is nearly independent of its photon energy so long as the average photon energy is >200 keV. In this energy range, dose deviates from inverse-square law by <5% over the 1- to 5-cm distance range. All of the “radium substitute” isotopes, including 137Cs, 192Ir, and 198Au, fall into this energy range. This behavior, which greatly simplifies BT dosimetry, is the result of equilibrium between primary photon attenuation and buildup of scattered photons. Only for low-energy sources (e.g., 103Pd and 125I) does the depth-dose curve significantly deviate from inverse-square law. Because photon absorption rather than Compton scattering dominates energy deposition below 40 keV, scatter buildup is unable to compensate for loss of dose resulting from attenuation.
For radium-substitute radionuclides, Figure 22.4A,B shows that the absolute dose rate (cGy/hour to fat or water tissue per mgRaEq or unit air-kerma strength [SK]) and the relative dose distribution are nearly independent of both energy and composition of the surrounding medium above 100 keV. Compton scattering, which dominates photon absorption and scattering above 100 keV, depends mainly on electron density (electrons/g) of the medium, which is nearly constant for all biologic materials. Below this energy range, absolute and relative dose distributions vary significantly with energy and composition (atomic number) of the surrounding medium. Implanting an 125I seed in fat medium (effective atomic number of Zeff = 6) will deliver about half the absorbed dose at 1 cm, compared to the expected dose in water (Zeff = 7.5). This is because energy absorption per unit mass from photo-effect interactions is proportional to the cube of the atomic number (Zeff3) of the medium. Despite the significant impact of tissue composition heterogeneities on low-energy seed BT dose delivery, current treatment planning and dose measurement practices assume that patients are composed of uniform homogeneous water media.
FIGURE 22.3. A: Variation of dose as a function of distance for point sources of 60Co, 226Ra, 137Cs, 198Au, 192Ir, and 125I. The results are normalized to 100% at 1-cm distances. The function (1/r2) is plotted for comparison. B: Relative dose (normalized to 1.0 at 1 mm) versus distance for various cylindric sources (0.65 mm diameter and 5 mm long) over the 1- to 5-mm distance range. (From Amols HI, Zaider M, Weinberger J, et al. Dosimetric considerations for catheter-based beta and gamma emitters in the therapy of neointimal hyperplasia in human coronary arteries. Int J Radiat Oncol Biol Phys 1996;36:913–921, with permission from Elsevier.)
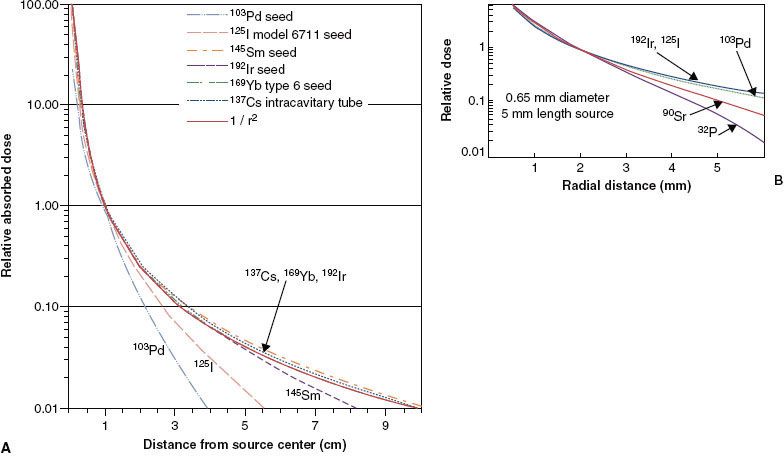
Figure 22.4B demonstrates that the inverse-square law actually underestimates relative dose at 5 cm by as much as a factor of 2 in the 60- to 100-keV energy range. In this narrow energy range, called the intermediate low-energy range, photoelectric effect is negligible, whereas Compton scattering transfers most of the colliding primary photon energy to the scattered photon rather than to the Compton electron. As a result of this imbalance between energy absorption and photon scattering, buildup of scattered photons overcompensates for loss of dose as a result of primary photon attenuation out to distances of 4 to 6 cm.
Above the 100-keV threshold, the photon-energy spectrum is much less important to optimizing BT dose-rate distributions than in external-beam therapy. Because artificial BT radionuclides in this energy range (60Co, 137Cs, 192Ir, 198Au) have dose-rate distributions nearly identical to those of 226Ra in the 1- to 5-cm distance range, they are referred to as radium substitutes.
Figure 22.4C demonstrates that although photon energy is a relatively unimportant determinant of tissue dosimetry, it significantly influences the cost, weight, and thickness of shielding required to protect critical anatomic structures in the patient and personnel involved in patient care. The half-value layer (HVL) in lead varies from 0.5 mm for a 100-keV source to 12 mm for 60Co BT sources. Thus, for classical radium-equivalent BT, a radionuclide with a mean energy of about 100 to 200 keV is optimal. The major benefit of 125I and 103Pd BT sources is the ability to provide complete protection by thin lead foils (0.1 to 0.2 mm), greatly reducing exposure to physicians during the implant procedure and allowing permanent-implant patients to be released from medical confinement without posing a radiation safety hazard to the general public. Recently, interest has been expressed in using radionuclides in the intermediate low-energy range (60 to 120 keV).6,7–8 Tissue dose distributions are still approximately radium equivalent in this energy range, and thin layers of lead provide significant sparing of dose-limiting normal tissues near the implanted volume.
FIGURE 22.4. Variation of dosimetric properties of monoenergetic point sources as a function of photon energy. The location (in terms of average energy) of commonly used radionuclides is indicated by the labeled vertical arrows. A: Absolute dose rate per unit source strength in fat and water media at 1-cm distance. Source strength is specified in terms of output in air. B: Dose at 5 cm as a fraction of dose at 1 cm in fat and water media. The effect of inverse-square law, (1/5)2 = 0.04, is shown for comparison as a heavy black line. C: Half-value layer in lead, the thickness (mm) in lead required to reduce primary dose by a factor of 2.
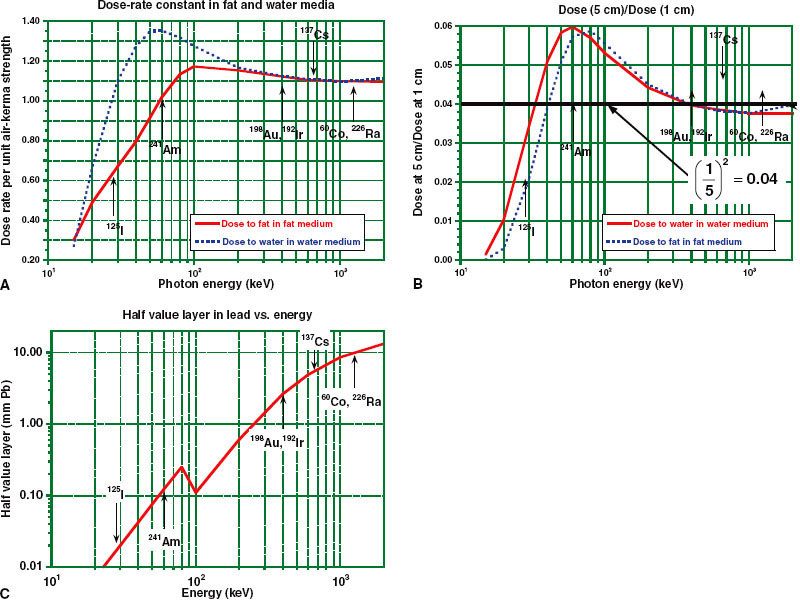
Sources for Low–Dose-Rate Intracavitary Brachytherapy
Since the 1930s, sources for classical LDR intracavitary BT have taken the form of “tubes” having a physical length of 2 to 2.5 cm and an external diameter of about 3 mm. For treatment systems influenced by the Manchester9 and M.D. Anderson10 treatment techniques, active lengths of 1.3 to 1.5 cm are typical. Radionuclides for intracavitary applications should have a half-life long enough to support a 5- to 10-year working life without large variations in prescription dose rate so that the high cost of these reusable sources can be amortized over a large number of patient treatments. The average photon energy should be at least 60 to 100 keV, as the dose fall-off for lower-energy sources (e.g., 125I) is too rapid to adequately treat the target volume periphery (2 to 5 cm from the applicator center) without overtreating the mucosal surfaces in contact with the applicator system.
Radium 226 Sources
Radium 226, a naturally occurring radionuclide, was the first radionuclide isolated, intensively investigated, and used in clinical BT. The unit of activity, the curie (Ci), originally was defined as the rate of disintegration within 1 g of 226Ra. Radium 226 has a complex decay scheme, consisting of a cascade of transformations from one daughter product to another, ending with a stable isotope of lead, Pb. Radium decays to gaseous 222Rn with a half-life of 1,626 years. Approximately 75 γ-rays are emitted by radium and its decay products, ranging in energy from about 0.05 to 2.4 MeV, giving an average energy of about 0.8 MeV. The maximum β-ray energy is about 3.26 MeV. The exposure-weighted average energy of 226Ra is 1.25 MeV when its photon spectrum is filtered by 0.5 mm of platinum. Nearly all 226Ra BT sources are filtered by at least 0.5 mm Pt, which reduces the surface dose contributed by β particles to a negligible level.
Clinical 226Ra sources consisted of discrete cells of radium salt (radium sulfate plus filler) placed in needles or tubes with platinum walls of thickness of 0.5 and 1.0 mm, respectively. Intracavitary radium tubes were usually 22 mm long, containing 5 to 30 mg of radium (SK = 30 to 200 μGy · m2 · h−1), with active lengths of 15 mm. For interstitial BT, the full-, half-, and quarter-intensity needles popularized by the Manchester LDR implant system typically contain 0.66 mg, 0.33 mg, or 0.165 mg of radium per centimeter of active length, respectively.
The clinical use of radium has disappeared and is now only of historic interest. The potential for damaged sources to leak radioactive salts or emit radon gas (222Rn) is the major reason for its decline, as well as the exposure hazard to interstitial BT practitioners.11 Another factor is the high cost of extracting radium from pitchblende ore in comparison with the cost of radium-substitute sources. Finally, the safe disposal of spent 226Ra sources is a significant financial liability. However, because of its many years of therapeutic use, several widely used quantities for source-strength specification and prescription of intracavitary treatment are derived from the early experience with 226Ra.
Cesium 137 Sources
Cesium 137, a fission byproduct, is a popular radium substitute because of its 30-year half-life. Its single γ-ray (0.66 MeV) is less penetrating (HVLPb = 0.65 cm) than the γ-rays from radium (HVLPb = 1.4 cm) or 60Co (HVLPb = 1.1 cm). Because 137Cs decays to solid barium 137, 137Cs sources have virtually replaced 226Ra intracavitary tubes in LDR gynecologic applications.
Cesium 137 BT sources were introduced in the early 1960s.12,13 Recently marketed sources (e.g., the Amersham model CDCS-J tube [Amersham, UK] and 3M model 6500 intracavitary tube [St. Paul, MN]) consist of radioactive cesium distributed within an insoluble glass or ceramic matrix,4 which produces far less radiochemical hazard from ruptured sources than does the radon gas or cesium salts. These sources are encapsulated in stainless-steel sheaths with wall thicknesses of 0.5 to 1.0 mm, active lengths of 13.5 to 15 mm, diameters of 2.6 to 3.1 mm, and total lengths of about 20 mm. Figure 22.5 shows that cesium and radium sources produce nearly identical transverse-axis dose-rate distributions when their active lengths and source strengths are the same. However, the 226Ra tube isodose curves exhibit significant retraction along the longitudinal source axis as a result of oblique filtration of 226Ra γ-rays through the dense (ρ = 21 g ⋅ cm–3) 1-mm-thick platinum capsule. In contrast, lightly filtered 137Cs tubes produce nearly elliptical isodose curves. Consequently, vaginal applicator systems containing modern 137Cs sources with their axes positioned perpendicular to the coronal patient plane (e.g., the Fletcher colpostat) always will give rise to higher bladder and rectal doses than when loaded with 226Ra tubes.14
Many other 137Cs source designs have been used over the last 20 years including spherical steel-encapsulated 137Cs pellets for the Selectron-composable source-train remote afterloader.15 For preoperative treatment of endometrial cancer16 or definitive treatment of medically inoperable endometrial cancer, afterloading sources with nominal strengths of 72 μGy · m2 · h−1, external diameters of about 1.2 mm, and lengths of 12 mm attached to the end of long metal stems (Heyman-Simon sources) were widely used up to the present time. However, all of these 137Cs source configurations have disappeared from the market, reflecting the widespread conversion of LDR intracavitary BT to HDR techniques. As of this writing, only intracavitary tubes are commercially available from two manufacturers: Isotope Product Laboratories (Valencia, CA)17 and Bebig-IBt (Berlin, Germany).18
Experimental Intracavitary Brachytherapy Radionuclides
Californium 252 is a unique radionuclide that decays by α-emission with a half-life of 2.65 years and emits neutrons by spontaneous fission with average energies of 2.1 to 2.3 MeV. Depending on the distance from the source, one-half to two-thirds of the total dose is the result of the neutron component. Assuming a relative biologic effectiveness (RBE) of 6 for the neutron component, approximately 90% of the biologically effective dose derives from the neutron component. The radiobiologic rationale for using 252Cf, especially in treating bulky gynecologic malignancies, is that the high linear energy transfer (LET) neutron component more effectively depopulates the tumor’s radioresistant hypoxic core, thereby improving local control, while the rapid dose fall-off maintains an acceptable level of late complications.19 Californium 252 sources require carefully designed radiation protection and source handling procedures to reduce radiation exposure hazards to an acceptable level, due to the high neutron quality factor of 10 to 20 that is assumed by radiation protection standards.20 Afterloading tube sources, suitable for use in LDR intracavitary BT, are fabricated at Oak Ridge National Laboratories (Oak Ridge, TN).21
Ytterbium 1697 and americium 2416 are examples of so-called intermediate low-energy photon emitters, giving rise to 60-keV and 100-keV photons, respectively. The emitted photon energy is low enough that relatively thin lead foils can be used to shield personnel and dose-limiting tissues in the patient but high enough that the resultant dose distributions in tissue remain approximately radium equivalent.22 Because relatively thin lead sheets can be used to shield critical structures (e.g., 0.4-mm-thick lead for 50% dose reduction from 169Yb), customized rectal and bladder shielding can be more easily fabricated. Ytterbium 169 seeds7 (100-keV mean energy, 32-day half-life) have been investigated as a possible substitute for 192Ir in interstitial implants23 and for intracavitary treatment. In addition, 169Yb has an extremely high specific activity. An HDR 169Yb source and an associated single-stepping source remote afterloading system has recently been approved for sale.8
FIGURE 22.5. Comparison of isodose curves for a modern steel-clad 137Cs source (left) containing radioactive ceramic pellets14 and a 226Ra tube (right) consisting of a RaSO4 core encapsulated in 1-mm-thick Pt. Both sources have an air-kerma strength of 72 μGy · m2 · h−1 (10 mgRaEq).
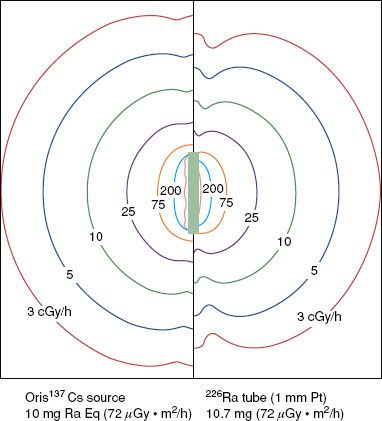
Sources for Low–Dose-Rate Temporary Interstitial Brachytherapy
The main additional requirement for radionuclides used in temporary interstitial BT is a specific activity sufficient to support fabrication of miniaturized sources (<2 mm external diameter) so as to minimize trauma to the implanted tissues. Current interstitial implantation techniques favor disposable sources containing short-lived radionuclides that support afterloading and customization of active length.
Nonafterloading (“Preloaded”) Sources: Radium and Cesium Needles
Radium 226 needles were the mainstay of interstitial BT until about 1970. These sources had external diameters of 1.5 to 2 mm, active lengths ranging from 3 mm to 4.5 cm, and Pt-Ir alloy encapsulation ranging from 0.5 to 0.65 mm in thickness. Because needle implantation can result in large exposures to the radiation oncologist’s fingers, as well as whole-body exposure to operating room and implant imaging staff, interstitial implantation of 226Ra nor 137Cs needles has been abandoned.
Afterloading Interstitial Sources: 192Ir Ribbons and Wires
Temporary interstitial BT experienced a renaissance in the 1960s because of the introduction of 192Ir.11 This useful radionuclide is produced by bombarding nonradioactive 191Ir (available in relatively pure form) with thermal neutrons in a nuclear reactor. 191Ir has an extremely large neutron-capture cross-section, and produces no significant contaminant radioisotopes. Because of these properties, very high specific activities can be achieved. Miniaturized interstitial sources can be fabricated relatively cheaply. The use of 192Ir in BT was pioneered by Ulrich Henschke,24 who developed a family of widely used afterloading techniques, and by Pierquin and Dutreix,25 who developed the 192Ir-based Paris interstitial system in the early 1960s.
Iridium 192 has a 73.8-day half-life and a complex decay scheme, dominated by β decay to 192Pt, but also including some electron capture and β+ decay. Its photon spectrum includes characteristic x-rays and γ-rays ranging from 63 keV to 1.4 MeV and has an exposure-weighted average energy of 397 keV. Compared with higher-energy 137Cs, the thicknesses of lead and concrete shielding can be reduced by 33% and 20%, respectively.26 More important advantages of 192Ir sources are compatibility with afterloading techniques, technical flexibility, and patient comfort.
In the United States, 192Ir is available in the form of seeds, 0.5 mm in diameter and 3 mm long, for LDR BT (Fig. 22.6). Iridium seeds, encapsulated in a 0.8-mm-diameter nylon ribbon and spaced at 1-cm or 0.5-cm center-to-center intervals, are available in strengths of 1 to 150 μGy · m2 · h−1 (0.1 to 20 mgRaEq). In Europe, 192Ir is used in the form of a wire (0.3-mm or 0.6-mm outer diameter) consisting of an iridium-platinum radioactive core encased in a 0.1-mm sheath of platinum. In addition to eliminating radiation exposure hazards in the operating room, 192Ir ribbons and wires can be trimmed to the appropriate active length for each catheter. Generally, 192Ir ribbons or wires are used only for one to three patient procedures and then returned to the vendor for disposal.
Low-Energy Sources for Temporary Interstitial Brachytherapy
High-intensity 125I sources27 have been proposed for temporary interstitial implantation at classical dose rates. High-intensity model 6711 or 3631 A/M 125I seeds now are used routinely as temporary interstitial sources for episcleral plaque treatment of intraocular choroidal melanoma.28 By placing a 0.5-mm-thick gold shield over the episcleral plaque, tissues posterior to the eye are shielded, and radiation directed toward the tumor is partially collimated.29 A disadvantage of high-intensity 125I seed therapy is its high cost relative to 192Ir seeds.
FIGURE 22.6. A: Construction and dimensions (in mm) of the two types of commercially available 192Ir seeds. (A from Williamson JF. The accuracy of the line and point dose approximation in Ir-192 dosimetry. Int J Radiat Oncol Biol Phys 1986;12:409, with permission from Elsevier.) B: The 0.8-mm external diameter nylon carrier or ribbon in which the seeds are “encapsulated.” (B from Anderson LL, Nath R, Weaver KA, et al. Interstitial brachytherapy: physical, biological and clinical considerations. New York: Raven, 1990.)
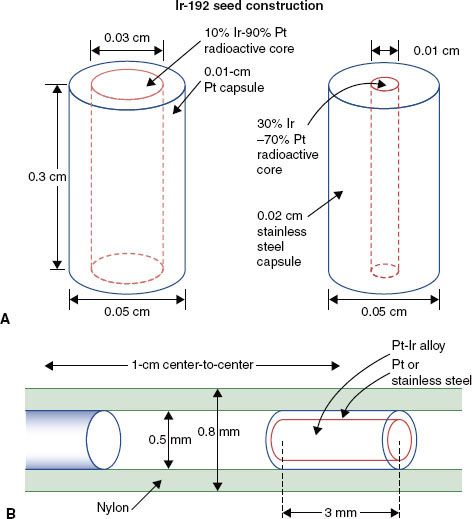
Sources for Permanent Interstitial Brachytherapy
There are two basic approaches to permanent implantation. Classical LDR permanent BT originally used 222Rn seeds, and more recently 198Au seeds, both of which have half-lives of a few days. To manage the radiation hazard as a result of the high-energy γ-rays emitted by these sources, the patient must be confined to the hospital until the source strength decays to a safe level (two to three half-lives or about 10 days). The contemporary approach to permanent implantation, ultra-low–dose-rate (ULDR) BT, uses longer-lived but low-energy photon emitters (e.g., 103Pd and 125I). The patient’s tissues or a thin lead foil are sufficient to reduce ambient exposure rates to negligible levels, eliminating the need to hospitalize patients solely for radiation protection. During the implant procedure, low-energy photon sources markedly reduce radiation exposure to operating room personnel and to the radiation oncologist’s hands.
Mathematics of Radioactive Decay
The phenomenon of exponential decay results in a reciprocal relationship between dose rate achieved and radionuclide half-life. The total activity, A(t), present in the implant after an interval of time t has elapsed after source insertion is given by Figure 22.7:
where A(0) is the activity at the time of insertion, ln2 is the natural logarithm of 2 (equal to 0.693), and T1/2 is the half-life of the radionuclide. The quantity ln 2/T1/2, represented by the symbol λ, is called the decay constant. Eq. (3) is applicable to any measure of source strength (SK, equivalent mass of radium, etc.). Because dose rate, (t) at time t is proportional to activity, that is,
, we can write:
where (0) is the dose rate at the time of source insertion. The total dose, D(T), accumulated over time interval T after source insertion is the shaded area under the curve of Figure 22.7 and can be obtained by integrating Eq. (4):
The product Ta = 1.443T1/2 is called the average life of the radionuclide and is the time required for all radioactive atoms to decay, assuming the rate of decay remains fixed at its initial value, A(0). Eq. (5) should be used to calculate the total dose delivered by any implant when the treatment time, T, is more than 5% of the half-life. For shorter treatment times (<4 days for 192Ir or <3 days for 125I), the approximate expression
is accurate within 2% and may be used.
For permanent implants, the total dose administered to the patient, Dtot, resulting from complete decay of the implant can be obtained from Eq. (5):
This equation demonstrates that initial dose rate and radionuclide half-life are in reciprocal relationship with one another: the longer the half-life, the lower the dose rate will be. Typical total dose rates and total doses are given in Table 22.2 for commonly used permanent implant sources. These sources fall into two categories: short-lived radium-substitute sources with initial dose rates within the classical LDR range and longer-lived low-energy sources with dose rates below the classical range (ULDR).
TABLE 22.2 TOTAL DOSE AND INITIAL DOSE RATES FOR PERMANENTLY IMPLANTED RADIONUCLIDES
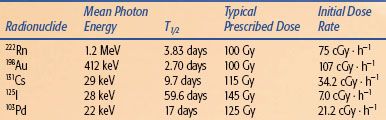
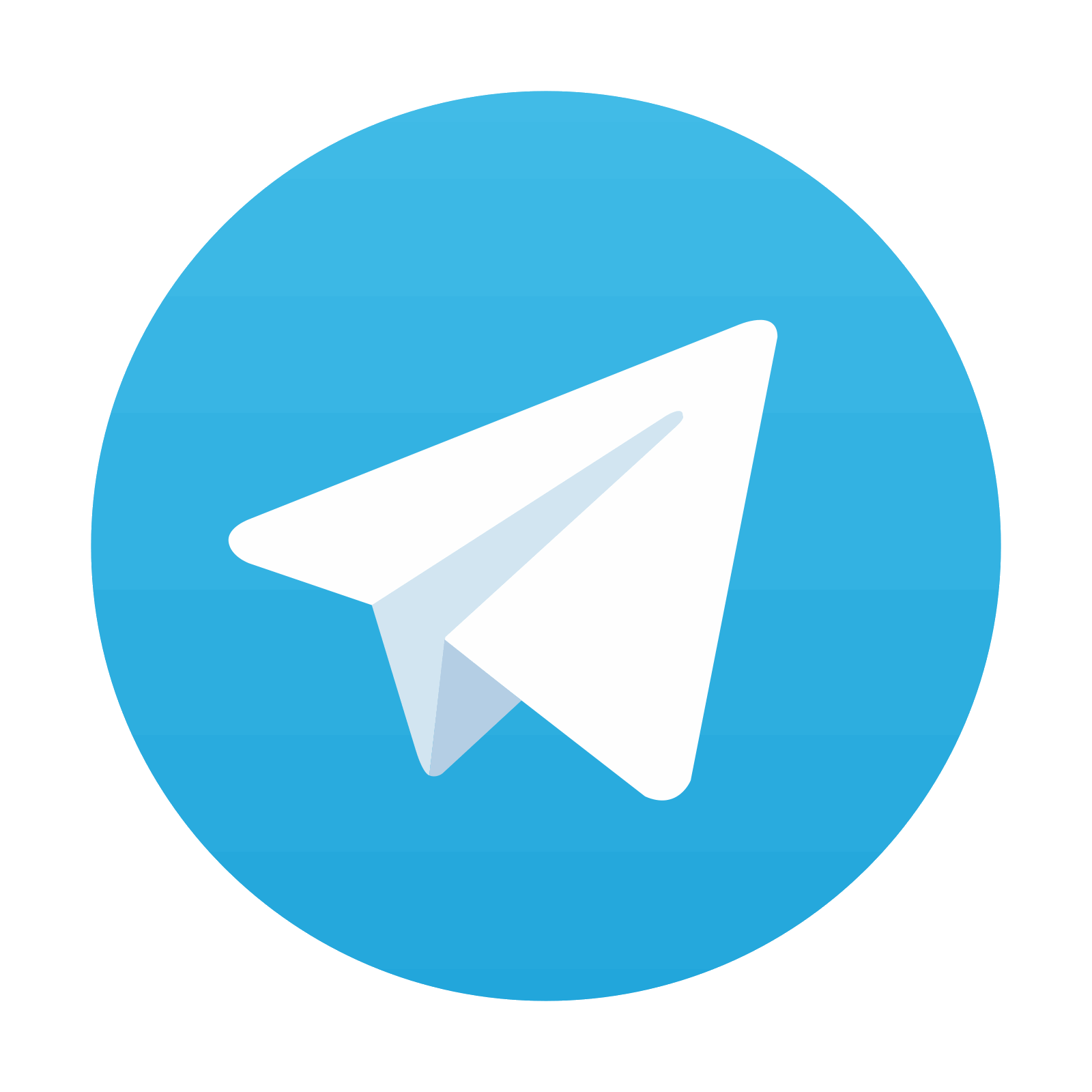