Physical Barriers to Drug Delivery
Brendan D. Curti
Our understanding of cancer biology has been transformed over the last 20 years. An illustration of how much our consciousness about oncology has changed can be found by looking at the topics covered in the educational sessions of the annual American Society of Clinical Oncology meetings from 1990 through 2009. In 1989, most sessions were named after cancer diagnoses, such as “Breast Cancer,” or “Adult Lymphoma.”1 In 1999, educational sessions were held on angiogenesis, signal transduction, and tumor suppressor genes.2 In 2009, the focus of the meeting was on using real-time and comprehensive knowledge of genomics, signaling pathways, proteomics, and informatics to understand the biology of tumors from individual patients.3 Although genetic events and receptor-protein interactions are central to the biology of any cancer, insights about the macroscopic aspects of tumor physiology complement this molecular knowledge. The study of tumor physiology and the interstitial space of tumors, pioneered by Gullino in the 1960s and continued most recently by Jain and colleagues, suggests a new basis for understanding drug delivery problems in tumors.4, 5, 6, 7 Their work has defined tumor nodules in vivo as distinct physiologic entities with unique, biophysical properties compared to normal tissues. These properties cannot be deduced or reproduced by in vitro work because of the complex interaction of growing tumor cells with the new blood vessels they induce and the surrounding normal tissues. The microenvironment of tumor nodules can generate physical barriers to most therapeutic agents. These barriers cannot be ignored when designing new cancer treatments. Therapeutic agents that target tumor vasculature may alter some of these physiological barriers causing “vessel normalization,” a term first coined in 19728 but now applied to the changes in the tumor vasculature induced by antiangiogenic therapies.9
The characteristics of tumor blood vessels and their behavior in the aggregate influence the transport processes used for the delivery of nutrients and therapeutic agents and the disposal of metabolic wastes. These vessels also have a profound secondary effect on the properties of the interstitial space of tumors. The emphasis here will be to provide a general discussion of physiologic spaces, transport variables, and the properties of tumor blood vessels. These basic concepts will be used as a framework for the discussion of intratumoral pharmacokinetics, selected models for the transport of therapeutic agents in tumors, and measurements of the physical barriers to drug delivery.
Transport Compartments and Variables
The vascular space, vascular endothelium, and vessel basement membrane must be traversed before a therapeutic molecule can percolate through the tumor interstitium to reach the cancer. Each of these physiologic spaces contains potential impediments to transport. To understand these impediments, the general mechanisms for transport of any molecule must be understood.
Convection and diffusion are the most important ways that solutes (i.e., nutrients, toxins, and therapeutic agents) are propelled through physiologic spaces. The relative contribution of these variables to transport depends on the size and physical properties of the molecule and the physiologic space it is moving through. In this discussion, transcytosis is not considered as it does not significantly influence cancer drug delivery. Each transport variable is discussed separately, followed by an explanation of how these variables interact and are influenced by the complex behavior of tumor vasculature.
Convection
Convection is defined as the movement of a solute in the direction of solvent flow. This process can be envisioned as water flowing down a pipe. If a solute (e.g., sodium chloride) is dissolved in the water, the salt molecules will travel with the water flow. Convection depends on a series of constants that describe how the solute and solvent move together, multiplied by a pressure gradient.10
Convection in tumors has been measured in a number of model systems. Fluorescent photobleaching has been applied to the study of single vessels in granulation tissue and VX2 carcinoma grown in a rabbit ear chamber model.11 The velocity of the fluid moving through the interstitium of this preparation was comparable in both granulation tissue and tumor, suggesting that convective flux was the same in these model systems. Indirect data, using gravimetric or implanted chamber techniques, indicate a convective flux from the tumor to surrounding normal tissues that is 10 to 1,000 times greater than in normal tissues. These numbers reflect bulk flow and do not define the events occurring at the blood vessel-tumor interface. Data obtained from measurements of arterial and venous hematocrits in tissue-isolated tumors (i.e., tumors on a vascular pedicle with one artery entering the tumor nodule and one vein leaving it) show a marked hemoconcentration from the arterial to venous circulation.12 In models using Walker 256 and MTW-9 mammary carcinomas, the hematocrit of the tumor efferent veins averaged 1.043 times greater than aortic arterial blood. The authors estimated that 4.5% to 10.2% of the perfusing plasma volume was lost into the tumor interstitium. This implies that plasma left the tumor blood vessels and percolated through the tumor, and it was
discharged into the surrounding normal tissues and absorbed by peritumoral lymphatic vessels or veins. The data show a net convective flux away from the tumor. Direct measurements of convection in human tumor nodules do not exist.
discharged into the surrounding normal tissues and absorbed by peritumoral lymphatic vessels or veins. The data show a net convective flux away from the tumor. Direct measurements of convection in human tumor nodules do not exist.
Diffusion
Diffusion is defined as the movement of solute along a concentration gradient. The force that causes a drop of ink to spread through a beaker of water is diffusion (stirring the water in the beaker would move the ink by convection). Diffusion is dictated by the concentration gradient and a constant that depends on the transport medium.10
Diffusion across individual tumor blood vessels was measured in a rabbit ear chamber model using fluorescein-labeled immunoglobulin (Ig) and showed that tumor blood vessels have high effective diffusion coefficients compared to normal tissues.13 The high diffusion coefficient implies that transport by diffusion was greater across tumor blood vessels than normal vessels in this model. Diffusion varied greatly among individual tumor vessels. Some tumor vessels have diffusion coefficients that are nearly normal, whereas others are approximately four times higher than normal vessels.
Again, no direct measurements of diffusion have been made in humans, but estimates of the diffusion coefficient in pancreatic, hepatic, prostate, breast, ovarian, lymphoma, head and neck, and brain tumors have been made with magnetic resonance imaging (MRI) (reviewed by Padhani et al.14). The apparent diffusion coefficient (ADC) measured with MRI is based on the movement of water through tissues and is normally dictated by Brownian motion, but it is also influenced by cell density, cell swelling, apoptosis, and amount of extracellular matrix.15 The ADC in most tumors is less than normal tissue or benign cysts.16 It should be emphasized that the ADC measured by MRI is more akin to bulk flow within a tumor and is not measuring the same physical property as the transcapillary diffusion coefficient discussed above. Although there is not yet a consensus on the best technique and calibration of ADC by MRI,14 it is becoming a common assessment tool in diagnosing tumors and evaluating response to therapy.
Diffusion is influenced by both the composition of the interstitium and the cellularity of the tissue. The relative contribution of each component has not been defined until recently. Chauhan et al.17 combined spatial Fourier analysis fluorescence recovery after photobleaching (SFA-FRAP) with multiphoton fluorescence recovery after photobleaching (MP-FRAP) using confocal microscopy. This combination of techniques allows diffusion to be assessed over multiple path lengths and distinguishes between diffusion in cellular and extracellular matrix. The investigators examined dorsal skinfold chambers in severe combined immunodeficiency (SCID) mice implanted with human sarcoma and colon cancer xenografts. They also made collagen/hyaluronan gels of differing composition ratios for study. Diffusion was enhanced by reducing cell content and collagen. Decreasing hyaluronan did not affect diffusion in the studied model systems.
Interstitial Space
Before discussion of the interactions and relative importance of these transport variables in tumors, the composition of the interstitial space must be reviewed, along with an additional physiologic variable—namely, interstitial pressure (IP).
Composition and Properties of Tumor Interstitium
The interstitial space of tumors is greatly different from that found in normal tissues. Furthermore, the interstitium varies between tumor types, sites of metastasis and perhaps even between different nodules of the same histology.18,19 Even though the tumor interstitium is quite variable, some generalizations can be made to illustrate the differences between normal tissues and tumors.
There is a range of values in the literature for different components of the interstitial space measured in different tumor models. The volume of the interstitial space of several animal tumor models has been measured and was found to be 36% to 53% of the total tumor volume.19 This compares with 14% to 34% in normal tissues.20 The total protein content of the interstitial fluid can be either higher or lower than normal serum, depending on the model studied. Gullino et al.4 showed by implantable capsules in a rat model that total protein in the interstitial fluid in a variety of tumor implants was consistently lower than aortic serum by approximately 33%. Sylven and Bois21 compared tumor interstitial fluid sampled by micropipettes to peritoneal fluid in a variety of carcinomas grown subcutaneously in mice. These researchers found that the protein content of the tumor fluid was 5% higher than that of peritoneal samples. The composition of the protein matrix in tumors is also different from normal tissues. The amount of type IV collagen and glycosaminoglycans is much greater in tumors than in the normal interstitial space.18,22
Direct measures of the interstitial space pH and oxygen tension in human tumors have been made. Measurements of pH in a series of melanomas ranged from 6.4 to 7.3.22,23 Some authors have reported tumor pH values less than 6 in squamous cell carcinomas and astrocytomas.24 The extremely acidic interstitial milieu of tumors was thought to occur from lactic acid production during anaerobic and aerobic glycolysis. Studies of glycolysis-deficient ras-transfected lung fibroblasts grown in subcutaneous tumors in nude mice have shown that an acidic microenvironment in vivo can be generated by tumor cells that do not make lactic acid.25 There was no significant difference in interstitial pH between glycolysis-deficient and parental tumor cells. These data suggest that alternative pathways exist for tumor to create and maintain an acidic microenvironment. The aberrant and decreased flow of blood within tumor vascular networks helps to establish and maintain the acidic microenvironment. Despite low pH values in the tumor interstitium, intracellular pH is normal.
Direct oxygen tension measurements in human breast cancers have shown partial pressures of oxygen as low as 0 mm Hg.26,27 Six out of fifteen nodules tested had oxygen tensions between 0.0 and 2.5 mm Hg. Intertumoral oxygen tension differences were more pronounced than intratumoral values. Additional evidence on the heterogeneity of tumor pH and oxygen gradients was described by Helmlinger et al.28 These investigators studied single vessel pH and oxygen measurements at a resolution exceeding 10 μm in human colon adenocarcinoma (LS-174T) xenografts implanted in SCID mice using fluorescence ratio imaging and phosphorescencequenching microscopy. There was no correlation locally between
pH, oxygen profiles, and blood vessel flow. Perfusion of blood vessels did not assure oxygen delivery to the tissue served by those blood vessels. Although local conditions within the tumor implants were variable, there was a correlation between mean pH and oxygen tension. Durand and LePard29 used laser Doppler and image analysis techniques to assess perfusion and oxygenation in human cervical (SiHa) and colon (WiDr) cancer cell lines in nude or SCID mice after doxorubicin or mitomycin-C chemotherapy. Radiosensitivity was used as a surrogate measure of oxygenation. The investigators found a dose- and time-dependent decrease in tumor blood flow and oxygenation from doxorubicin, whereas mitomycin-C caused increases in blood flow.
pH, oxygen profiles, and blood vessel flow. Perfusion of blood vessels did not assure oxygen delivery to the tissue served by those blood vessels. Although local conditions within the tumor implants were variable, there was a correlation between mean pH and oxygen tension. Durand and LePard29 used laser Doppler and image analysis techniques to assess perfusion and oxygenation in human cervical (SiHa) and colon (WiDr) cancer cell lines in nude or SCID mice after doxorubicin or mitomycin-C chemotherapy. Radiosensitivity was used as a surrogate measure of oxygenation. The investigators found a dose- and time-dependent decrease in tumor blood flow and oxygenation from doxorubicin, whereas mitomycin-C caused increases in blood flow.
One can conclude from these data that the interstitial space of tumors is profoundly different from normal tissues, varies over time, and that tumors display a remarkable degree of metabolic diversity and resilience. These differences represent a mechanism for potential drug resistance if the therapeutic agent is not stable or functional in an acidic or hypoxic environment. The function of immune cells that infiltrate the tumor microenvironment is also influenced by the low pH and oxygen tension.30,31 Murine T or natural killer (NK) cells cultured under pH, glucose and oxygen tension conditions similar to those found in tumors showed marked decreases in interleukin-2-induced T-cell proliferation. Caldwell et al.32 showed that normoxia was conducive to better T-cell survival, but hypoxic conditions enhanced lytic function. Specifically, T cells grown at oxygen concentrations of 2.5% favored CD8+ T-cell lytic activity measured by a chromium-release assay. Secretion of IL-2, interferon-γ and vascular endothelial growth factor (VEGF) per cell was greater compared to growth at oxygen tensions of 20%. The combination of acidic and hypoxic conditions was not studied by these investigators.
Interstitial Pressure
IP is the result of oncotic pressure in the interstitial space, oncotic and hydrostatic pressures in the microvascular space, the reflection coefficient, and the hydraulic conductivity of the vascular wall and interstitial space (e.g., how well water can move through these tissues). IP can be measured in vivo in a number of ways, including implantable capsules, the wick-in-needle technique, and micropuncture techniques.33,34 The data for IP in normal subcutaneous tissue and muscle show a range of values in the literature from -2 to 4 mm Hg.35,36 IP was first noted to be elevated in animal tumors in 1950 by Young et al.37 In 1990, the first IP studies in humans were published.38, 39, 40, 41 These data indicated that many human tumor nodules have IPs much greater than in normal tissues ranging from median values of 4.7 mm Hg in non-Hodgkin’s lymphoma to 33 mm Hg in metastatic melanoma. In patients with cervical carcinoma, IP was correlated directly with the oxygenation status of the tumor and inversely with tumor response to radiation therapy.42 Other studies have failed to show a correlation between IP and oxygenation.42,43 Trends in IP profiles and response to treatment in patients with melanoma and lymphoma have been reported.39 In the 14 melanoma nodules examined, 11 progressed on a variety of immunotherapies. Ten of the progressing nodules showed an increase in IP over time. Three of three responding nodules showed a decrease in IP over time. All seven non-Hodgkin’s lymphoma nodules had a low IP at the start of treatment. Five of these nodules showed a complete response to chemotherapy or radiation therapy, and one was observed off treatment. The only lymphoma nodule that did not respond to treatment showed a significant increase in IP from 1 to 30 mm Hg at the time of tumor progression. Figure 7-1 depicts the difference in penetration of methylene blue tracer in a high versus low IP tumor in a murine model.
IP in animal tumor models is in equilibrium with microvascular pressure.44 These authors studied tissue-isolated R-3230AC mammary carcinomas grown in rats. Pressures in superficial postcapillary venules surrounding the tumor were equal to IP in the center and periphery of the tumor. The coupling between the microvasculature and IP derives from the intrinsic leakiness of tumor vessels. By using intravital microscopy to characterize the vascular status of implanted tumors and micropipette techniques to measure IP, the appearance of vascular loops and sprouts in developing tumors correlated with the appearance of elevated IP.45 Thus, angiogenesis
is linked to increased IP. There is also a time-dependence between pressure changes in the microvascular and interstitial spaces. Fluctuations in arterial pressure are transmitted to the tumor interstitium within seconds (11 ± 6), whereas cessation of blood flow causes only a gradual decline in IP that takes 1,500 ± 900 seconds.46 The difference in time scales reflects transcapillary events in the former and percolation of fluid out of the interstitium in the latter. Another characteristic of IP is that it is transmitted uniformly through tumor nodules but decreases to normal tissue IP within 0.5 mm of the boundary of the tumor.47
is linked to increased IP. There is also a time-dependence between pressure changes in the microvascular and interstitial spaces. Fluctuations in arterial pressure are transmitted to the tumor interstitium within seconds (11 ± 6), whereas cessation of blood flow causes only a gradual decline in IP that takes 1,500 ± 900 seconds.46 The difference in time scales reflects transcapillary events in the former and percolation of fluid out of the interstitium in the latter. Another characteristic of IP is that it is transmitted uniformly through tumor nodules but decreases to normal tissue IP within 0.5 mm of the boundary of the tumor.47
![]() FIGURE 7-1 C57BL/6 mice with established subcutaneous MCA-38 colon carcinoma (A), or MC38C13 non-Hodgkin’s lymphoma (B). Both tumors were implanted and allowed to grow for approximately 20 days to a diameter of 2.5 cm. IP was 24 mm Hg in MCA-38 and 5 mm Hg in MC38C13 (mean of five measurements). Methylene blue (MB) was injected via tail vein, and mice were euthanized 30 minutes later. The uptake of MB is uniform in the low IP tumor (B), but it can only diffuse from the periphery in MCA-38 (A). This model was chosen to study tumor size and IP values comparable to many metastatic human tumors. (Please see Color Insert.) |
A model examining pressure, flow, and resistance relationships in the arterial and venous circulations of tumors and the surrounding tissue was developed by Zlotecki et al.48 They used their model to predict the effect of epinephrine, norepinephrine, hydralazine, nitroglycerin, and angiotensin on the tumor vasculature and sought to validate the model using laboratory observations and published data. The major conclusions from this analysis were that arterial control of blood flow in tumors is minimal, and the steal phenomenon (serial and parallel paths for blood flow) predominates. Vasoconstriction increased mean arterial pressures, tumor blood flow and IP, whereas vasodilators had the opposite effect. The model also suggested that elevated IP did not cause vascular collapse. Based on these data, one can infer that a cancer treatment that decreased mean arterial pressure would result in decreased IP. Most cancer treatments do not decrease blood pressure with the exception of high-dose interleukin-2.49 Many of the recently developed VEGF-targeted agents (bevacizumab, sorafenib, and sunitinib) increase blood pressure,50, 51, 52 but may decrease IP by other effects on the vasculature.
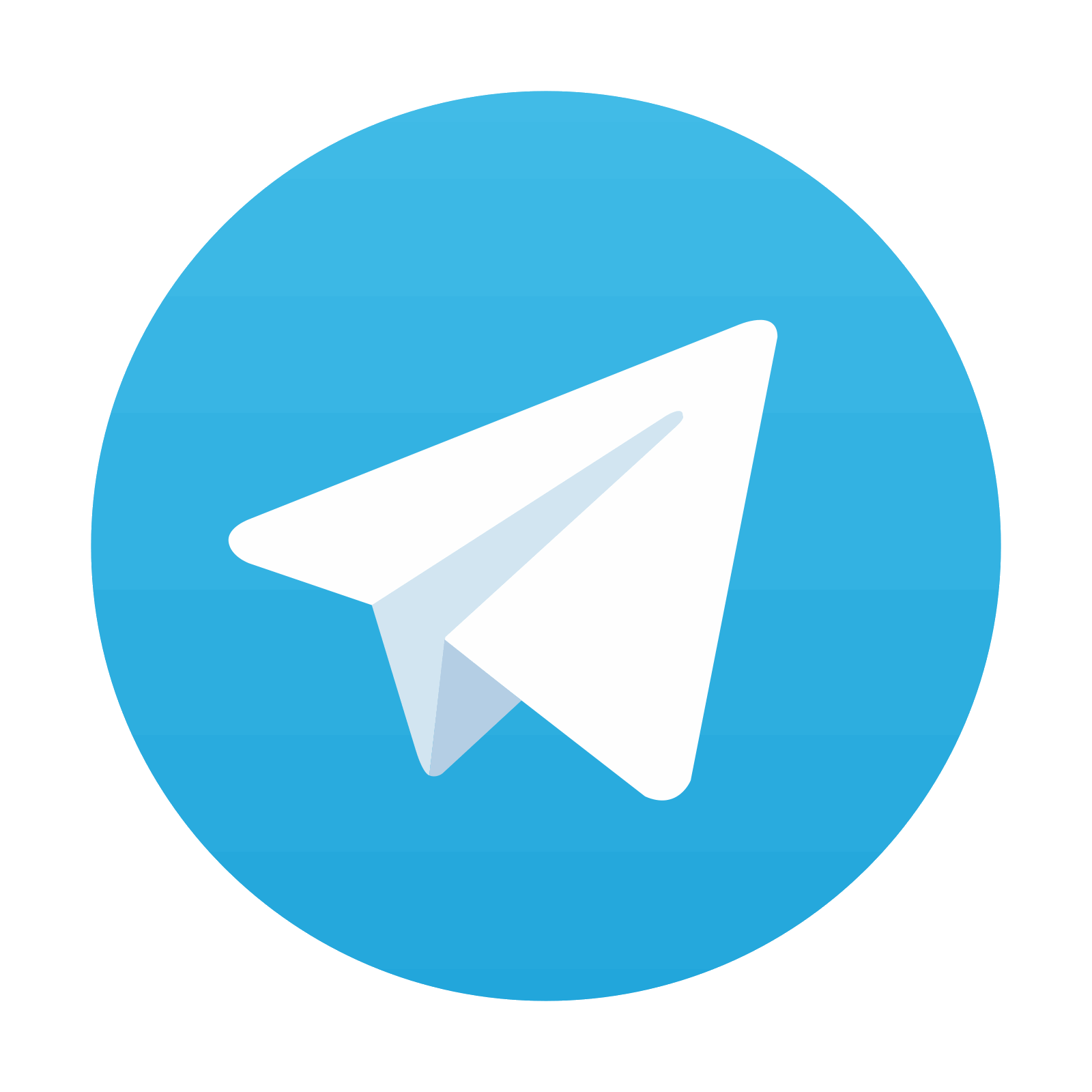
Stay updated, free articles. Join our Telegram channel
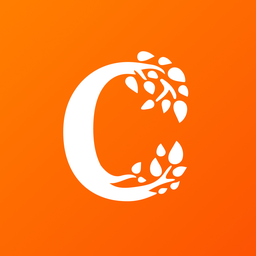
Full access? Get Clinical Tree
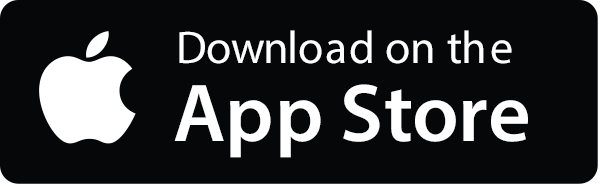
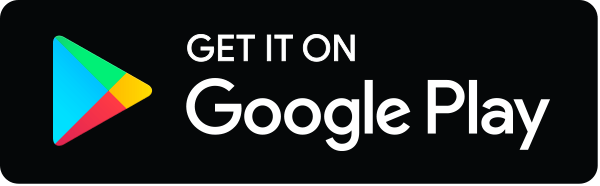