22.1
Physical activity across the life span for osteoporosis and fracture prevention
Physical activity (PA) is vital for musculoskeletal health and an important component of osteoporosis and fracture prevention across the human life span . Effective prevention strategies need to begin early as antecedents of osteoporosis likely originate during skeletal growth . Rapid bone accrual around the pubertal growth spurt is particularly critical and offers an opportunity for high-impact, weight-bearing PA to optimize bone development . Later in life, regular PA, including exercises targeting muscle strengthening, posture, and balance, can prevent deterioration in muscle and bone strength, and contribute to functional mobility of older adults, thereby the reducing propensity to fall and fracture . It is important to note that the focus of exercise in osteoporosis and fracture prevention diverges from optimizing bone strength development during childhood and adolescence to fall and fracture prevention in older age ( Fig. 22.1 ).
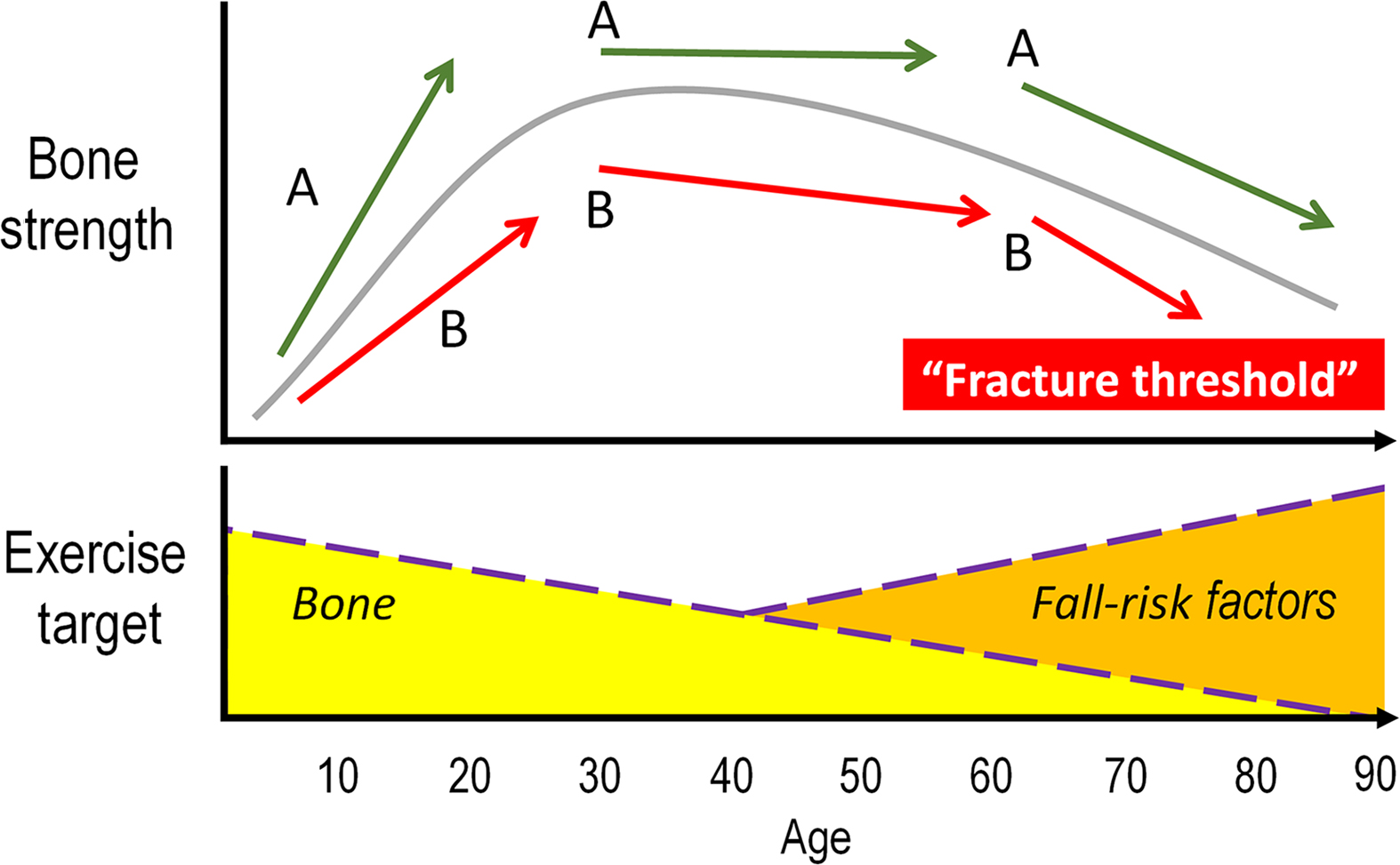
22.1.1
Antecedents of osteoporosis originate during skeletal growth
Over 40 years ago osteoporosis was recognized as a pediatric disease , indicating the critical role of optimal bone development during growth for later skeletal health . The 2 years preceding and surrounding the peak pubertal growth spurt are critical because youth accrue 40%–50% of adult bone mass, in both appendicular and axial skeleton, during these years . Importantly, a 10% increase in peak bone mass has been estimated to lower fracture risk by 50% or provide an additional 13 years before reaching the threshold for increased risk of osteoporosis and related fractures ( Fig. 22.1 ). Thus, late childhood and early pubertal years provide a critical period to optimize bone development and achieve the full genetic potential of skeletal health .
Traditionally, osteoporosis prevention strategies, including treatments for pediatric bone disorders, target bone mineral accrual to maximize peak bone mass by the end of skeletal maturation . From a functional perspective, however, bones should not be as heavy as possible. Instead, they should be light and as strong as necessary . The term peak bone strength is reported synonymous with peak bone mass in pediatric literature . Bone strength, though, integrates multiple factors, including bone mass, size, structure, and composition, and is a reflection of bone’s resistance to loading. As bone changes in size, structure, tissue composition, and mass due to growth, aging, or as an adaptive response to PA (i.e., loading stimulus) , it is important to estimate bone strength and complement this information with determinants, such as bone mass and size.
22.2
Imaging bone strength
Medical imaging tools offer noninvasive assessment and monitoring of bone properties, including estimation of bone strength across the human life span . Bone strength can be defined as bone’s resistance to fracture (i.e., failure load) for a given condition (e.g., impact arising from a fall) . The strength of a long bone depends on the amount and spatial distribution of bone mass (e.g., bone size, volume fraction, geometry, and internal architecture) and tissue properties (e.g., material density and composition of the organic matrix) . The clinical assessment of bone relies on dual-energy X-ray absorptiometry (DXA) imaging of areal bone mineral density (aBMD, g/cm 2 ), which is defined as bone mineral content (g) divided by the projected area of the scanned region . Due to the planar limitation of DXA, aBMD is influenced by bone size, resulting in (artificially) higher aBMD in individuals with bigger bones . To capture changes in bone size, structure, and (volumetric) density (BMD, g/cm 3 ), imaging tools need to measure bone in three dimensions. Computed tomography (CT) and peripheral quantitative CT (pQCT) scanners along with magnetic resonance (MR) 1
1 MR imaging captures measures of bone volume fraction. As density is the product of bone volume fraction multiplied by material density (which typically remains constant around 1.8 g/cm 3 ), MR can be used to estimate density.
imaging are clinical research tools to quantify size, structure, and densities within imaged regions of trabecular and cortical bone. Scanners, such as high-resolution pQCT (HR-pQCT), provide detailed information of bone micro-architecture, such as cortical bone thickness and porosity, trabecular number and thickness at the peripheral skeleton. These imaging tools are important when quantifying or monitoring changes in bone size and shape (external modeling), density and porosity (internal remodeling), as well as bone strength due to growth or loading stimulus . To estimate bone strength (e.g., failure load, stiffness), CT or MR images can be used in conjunction with an engineering-based computational tool known as finite element (FE) modeling . FE modeling evaluates how a structure with a complex shape and varying tissue properties behaves when loaded . By dividing a complicated bone structure into a finite number of small elements, the behavior of each element can be described mathematically and evaluated computationally. Effectively, this permits quantification of bone strength (e.g., failure load) or behavior (e.g., stiffness) without physically deforming or breaking the structure . Fig. 22.2 illustrates example images of a child skeleton obtained by DXA, forearm, and wrist bones by pQCT, details of distal radius micro-architecture by HR-pQCT along with FE estimates of strain energy distribution used to estimate bone failure load.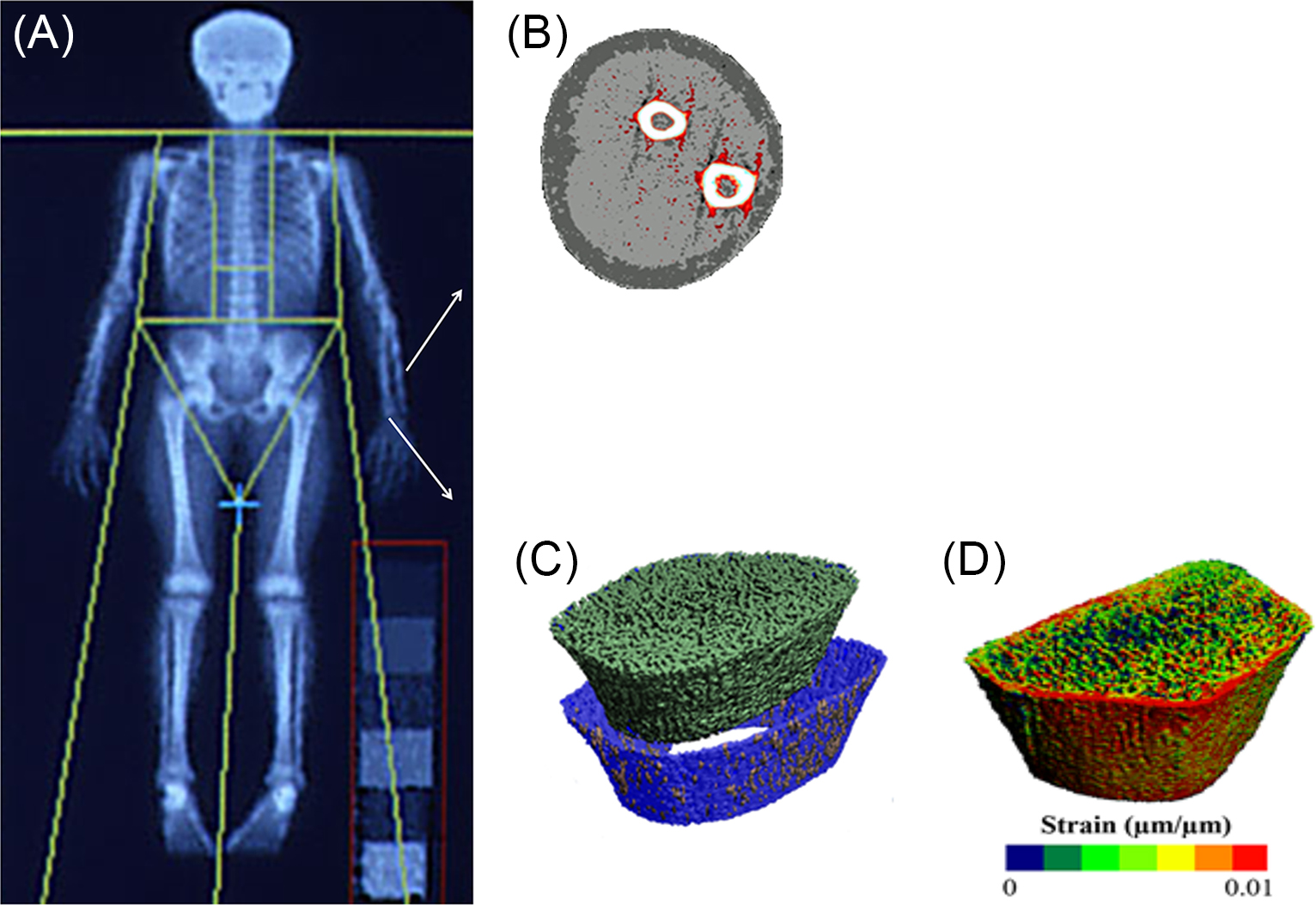
22.3
Physical activity, exercise, and measurement of bone loading stimulus
Mechanical loading from PA is key for skeletal health . Several systematic reviews have evaluated the role of PA and exercise on bone development . Two of these reviews focused on bone structure and strength adaptation to PA and targeted exercise interventions . Prior to discussing this evidence, we define PA and exercise and discuss related measurement tools in relation to the mechanostat model, a prevailing theoretical model of bone adaptation to loading stimulus .
PA is defined as any bodily movement produced by skeletal muscles that requires energy expenditure . PA includes activities such as playing, working, active transportation, household chores, and recreational activities . Exercise is a subcategory of PA, defined as planned, structured, and purposeful activity with a goal to improve or maintain one or more components of physical fitness .
Habitual PA measurement has traditionally relied on subjective estimations assessed via questionnaires , including those developed to estimate exposure to bone loading . Recently, wearable devices, such as accelerometers, have become a popular tool to objectively record PA, even in population-based epidemiological studies . Accelerometer recordings are often used to estimate the intensity of daily activity, typically categorized as light, moderate, or vigorous PA (VPA). These intensities have been validated to correspond to energy expenditure (oxygen consumption), making them particularly relevant for cardiovascular and metabolic health . Quantification of energy expenditure, however, is less relevant for bone adaptation than exposure to dynamic, impact types of loading associated with bone functional adaptation . To estimate bone loading stimulus from accelerometer data, “raw” acceleration peaks (assessed in relation to acceleration due to gravity, g ) have been validated against ground reaction forces in children and adults while performing activities such as jumping on a force platform , making these outcomes better suited for assessment of functional loading adaptation of bone.
22.3.1
Mechanostat model of functional loading adaptation
Genetic and environmental factors contribute to morphological features of bone . Adequate hormonal milieu, growth factors, and nutrition are required for skeletal adaptation to a loading stimulus . The mechanostat model explains bone adaptation to loading stimulus from a functional standpoint. This model involves a feedback loop that serves to maintain bone strain 2
2 Here we describe the mechanostat model in relation to strain magnitude. Strain rate (i.e., rate at which bone deformation occurs, with respect to time) as well as number of cycles of high strain and rest periods between the cycles are also reported to influence bone (re)modeling in animal experiments .
(i.e., deformation) within a customary range, defined by the mechanostat set points ( Fig. 22.3 ).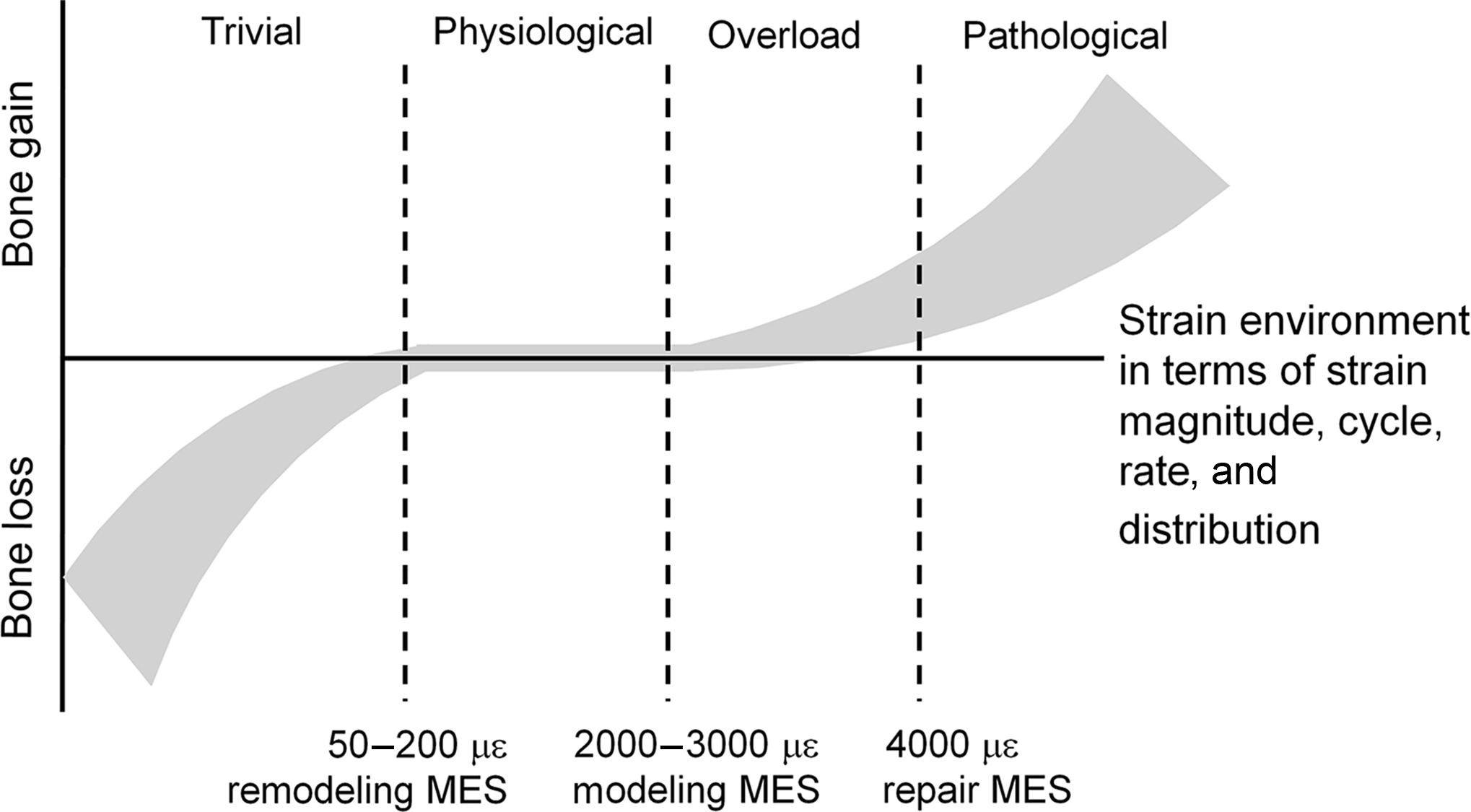
For example, a decline in bone strain (e.g., due to bed rest) increases bone (re)modeling with subsequent loss of bone mineral and alterations to bone structure (such as cortical wall thinning), thereby increasing bone strain back to the customary range. In contrast, initiation of a new unilateral activity (such as tennis training) exposes bone in the playing arm to an unusual strain environment. If bone strain exceeds the maximum effective set point for bone, (re)modeling and adaptation in bone mass and structure will occur (such as cortical wall thickening) . After bone adaptation the bone strain will subsequently return to the customary range . It is important to note that the strain set points in the mechanostat model are likely under genetic and hormonal control ( Fig. 22.4 ), which may contribute to superior loading adaptation in the growing versus mature skeleton .
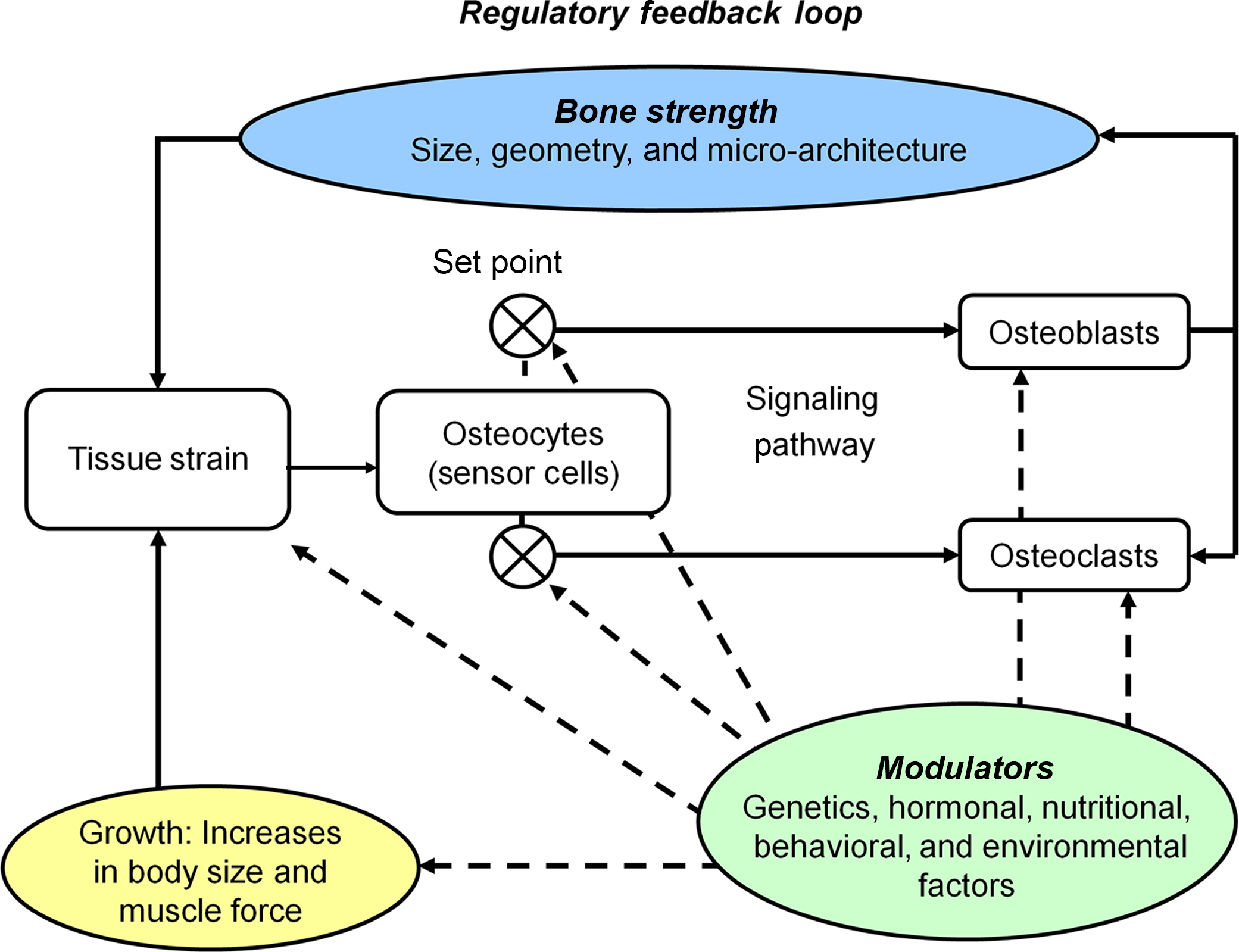
22.3.1.1
Estimating bone loading stimulus
The gold standard for defining bone strain is through strain gage instrumentation. Experimental data with strain gage instrumentation and noninvasive transcutaneous loading on animal bones has provided key information for understanding strain-related bone adaptation . Animal experiments have demonstrated bone adaptation to loading beyond simple strain set points, specifically highlighting the importance of dynamic strains, bone’s sensitivity to high strain rates, and unusual strain distributions as well as a requirement for only a few strain cycles with short periods of rest in-between cycles . Given that strain gage instrumentation is invasive, combined with the fact that an animal’s locomotive pattern or response to loading may not reflect a human’s response to loading, noninvasive means are needed to estimate bone strain. Medical imaging data together with computational tools (e.g., FE modeling described earlier, multibody musculoskeletal modeling) offers attractive and emerging noninvasive tools to assess how loading affects bone mechanical behavior . This information can improve understanding of bone adaptation to loading and guide exercise interventions as well as injury prevention strategies . For example, Troy’s group developed CT-based FE models of each participant’s radius to study how a specific loading/exercise task, with a targeted strain, would affect bone properties . Advancements aside, it is important to note that computational methods have a degree of uncertainty in estimating strain as they rely on several assumptions (e.g., appropriate relationship between CT/MR density and bone elastic modulus) . Accordingly, it is imperative that strain estimates from computational models are validated using cadaveric experimental testing . Validated models, combined with advancements in medical imaging and computational speed and capacity, will increase application of these tools in future clinical studies.
22.4
Bone strength adaptation to exercise in growing bone—systematic reviews
Several systematic reviews and two meta-analyses have evaluated the evidence relating PA and exercise to bone development . Two reviews focused on bone structure and strength adaptation to weight-bearing PA and exercise interventions, including impact-type activities (e.g., jumping) . A narrative synthesis of 14 interventions and 23 observational studies by Tan et al. concluded that bone strength adaptations to PA depended on skeletal maturity, sex, and study quality rating . For example, for weight-bearing PA intervention studies with a strong rating, the majority (3/5) reported 3%–4% greater increase in bone strength in the intervention group. In contrast, for intervention groups with a moderate rating, a minority (3/9) reported a significant bone strength gain . Increases in bone size (total bone area), cortical thickness and mass accompanied a significant bone strength gain . The meta-analysis of randomized controlled trials (RCTs) indicated a small effect of exercise on bone strength in pre- and early-pubertal boys only . Per-protocol analyses of individual trials in children and youth suggested that interventions incorporating regular weight-bearing exercise result in 1%–8% bone strength gain at the loaded skeletal sites . Both systematic reviews indicated a need for well-designed, long-term RCTs with adequate sample sizes to quantify the effects of exercise on bone strength and its structural determinants .
22.5
Bone strength adaptation to exercise in growing bone—recent interventions
After publication of these systematic reviews, a small number of exercise trials in children and youth assessing bone strength and structural adaptation have been reported . Daly et al. assessed the efficacy of a cluster RCT with over 700 boys and girls in 29 elementary schools, randomized to standard physical education (PE) classes or a specialist-taught PE program that emphasized vigorous exercises and games combined with muscle strengthening . After 4 years, girls in the intervention group had 5%–14% greater gain in cortical area and thickness at the tibia and radius shafts, while their male peers had a 5% greater gain in cortical thickness at the tibia shaft only. The greater gain in cortical thickness for the intervention group was due to lesser endocortical expansion, challenging the notion that periosteal apposition is the predominant response of bone to loading in pre- and early puberty . This cluster RCT was the first pediatric intervention monitoring bone structure and strength measures along with objectively estimated PA intensity of the intervention, providing important guidance for future trials.
Taken together, these findings may have clinical importance as advanced imaging studies suggests that bone micro-architecture, particularly cortical bone thickness, has a critical role in predicting fracture risk in children and older adults . Development of a thick cortex during growth may provide a protection against age-related cortical thinning (endocortical trabecularization) at fracture-prone long bone ends, such as the proximal femur or distal radius . However, the details of effective exercise protocols (e.g., type, frequency, or duration of bone loading activities) and optimal timing for bone adaptation rely on observational data and experimental studies using animal models . We discuss the related observational evidence in the following sections.
22.6
Maturity, sex- and site-specific adaptation—observational evidence
Observational studies, such as unilateral comparisons between bones of dominant (loaded) and nondominant (less loaded) arms, have provided compelling evidence of sex- and site-specificity affecting bone adaptation to loading. In addition, unilateral comparisons have suggested that the growing skeleton exhibits greater adaptation to exercise stimuli when compared to mature skeleton . This was demonstrated by two-to-four times greater unilateral benefits in bone mass, size, and strength in women who had started tennis playing prior, rather than after, menarche ( Fig. 22.5 ). Larger total bone area suggested greater periosteal bone formation and an increase in cortical thickness, which likely explained greater bone strength in young starters . Bone adaptation also appeared greater in the loaded sites of male than female players ( Fig. 22.5 ). Specific rationale for greater adaptation in men may be related to males having greater loading stimuli (strain magnitude and rate) due to larger body and muscle size or hormonal differences between sexes influencing bone adaptation.
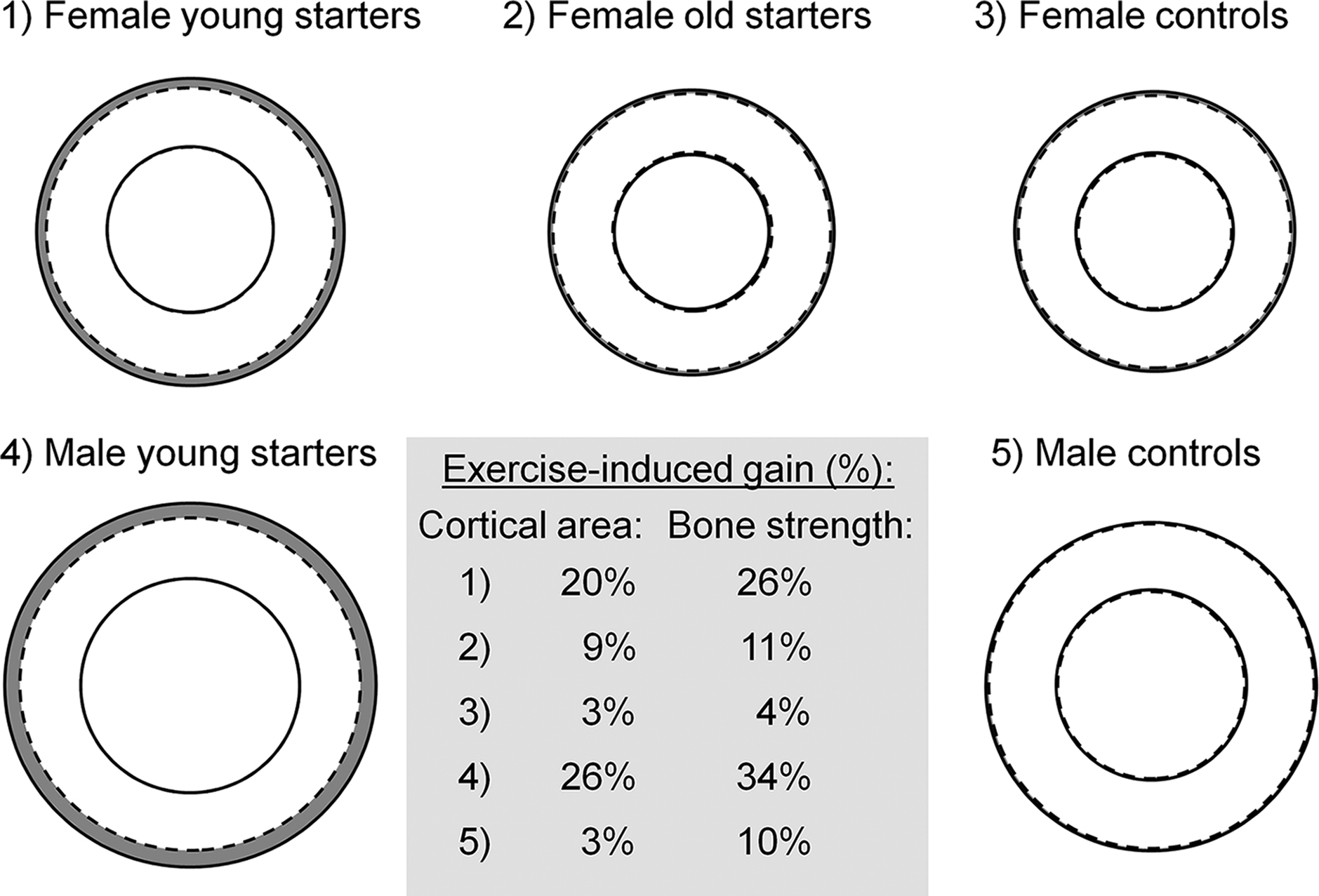
Unilateral loading studies have pinpointed a relatively short time period for optimal bone adaptation corresponding to early peripubertal years and pinpointed site-specific differences in bone adaptation within and between bones, regions, and surfaces . For example, a thicker cortex was due to a smaller medullary cavity in more mature female racquet sport players, suggesting either maintenance or formation of bone at the endosteal surface and an alternate mechanism to periosteal bone formation to increase cortical thickness. Importantly, in comparison to the periosteal surface, bone formation at the endosteal surface offered a similar contribution to compressive bone strength but a lower contribution to bending and torsional strength 3
3 Long bone resistance to bending and torsion is largely based upon how far cortical bone is away from the long axis of the bone (i.e., axis running along the medullary canal). As endosteal bone is closer than periosteal bone, endosteal formation will have less of an effect on bending and torsional strength than periosteal formation.
. Interestingly, a similar adaptation mechanism was observed in the 4-year specialist-taught PE program discussed earlier .Findings from athlete models, such as gymnastics, support observations from unilateral studies, reporting larger bone size, thicker cortices, denser trabecular bone, and greater estimated bone strength in bones exposed to dynamic gymnastic loading . A recent pQCT image-based FE study connected gymnastic loading during adolescence with symmetrical adaptations in bone geometry and strength at the distal radius, which improved resistance to loading from multiple directions . FE models of the proximal femur, derived using MR images, characterized region-specific adaptation in female athletes with a history of impact-type loading activities . The athlete groups had 11%–23% stronger bones (in terms of failure load), indicating better resistance to loading from a fall when compared to nonathletic controls or athletes with a history of repetitive, nonimpact loading activities (e.g., swimmers) .
22.6.1
Objectively recorded physical activity and bone adaptation—observational evidence
From a public health perspective, observational studies assessing the role of habitual PA are important since findings comparing athletes to nonathletic controls may not be broadly generalizable. For example, athlete comparisons are prone to self-selection bias as athletes may have engaged in the sport due to their stronger bones and muscles. Second, athletes’ exposure to the magnitude and duration of loading stimulus is beyond the habitual PA of the general population. Therefore observational evidence, particularly from longitudinal cohort studies, is valuable when defining PA guidelines and public health strategies for osteoporosis and bone fragility prevention.
Studies with accelerometer-recorded PA have identified habitual PA characteristics associated with bone strength. Daily minutes of moderate-to-vigorous PA (MVPA) or VPA were positively associated with estimated bone strength and size (i.e., total area, cortical bone thickness) at the weight-bearing tibia and femur in children, adolescents, and young adults . Janz et al. reported greater bone strength at the femoral neck, distal, and shaft sites of tibia in boys and girls who had accumulated the most MVPA, when compared to less-active peers . Alarmingly, authors noted that only a fraction of participants (<6% in girls) achieved high levels of MVPA throughout childhood and, by late adolescence, almost all girls were inactive (i.e., MVPA<60 min/day). These results indicate that skeletal benefits of PA are not being realized due to low levels of activity, especially among girls . HR-pQCT findings by Gabel et al. added to this evidence, pointing out 6% greater bone strength (failure load), 4%–6% larger total area, and ~13% greater cortical porosity at the distal tibia in adolescents at the upper (~60 min) versus lower (<30 min) quartiles of MVPA. Youth in the upper quartile had 4% greater trabecular bone volume fracture and 6% greater bone strength at the distal radius . We recently reported that adding 63 daily impacts could result in a 7% gain in tibia bone strength in children . Interestingly, our models with a 10-minute increase in daily VPA provided an equivalent 7% gain in bone strength, whereas a 10-minute increase in daily MVPA would result in only a 3% gain in bone strength at the tibia shaft . Similarly, adolescents in the upper quartile of short bouts (<5 min) of VPA (~33 VPA bouts) had 10% stronger distal tibia when compared to their peers in the lowest quartile (~9 VPA bouts/day). These findings suggest that objective estimates of bone impact counts and short VPA bouts (rather than MVPA) may offer a useful means for future trials striving to estimate the osteogenic stimulus in relation to bone strength adaptation in children and adolescents. To that end, the role of habitual PA on bone micro-architecture at the distal radius appears weak or nonexisting . It is important to note though that this may relate to the limitation of waist-worn accelerometers to capture loading at the upper extremity. Future studies should consider complementing waist-worn accelerometers with monitors estimating loading stimulus at upper extremity bones.
22.7
Maintenance of bone benefits from physical activity during childhood and youth
From a clinical perspective the importance of any bone benefits depends on their permanence. Accumulating evidence suggests that at least some of the bone strength and structural benefits acquired through PA during childhood and youth persist into adulthood and even into old age. The majority of studies examining the effects of PA during childhood and youth on adult bone mass have been limited to retrospective assessments of PA or earlier measured youth PA to adult bone mineral status. Follow-up data of retired athletes has provided evidence of both sustained and partially lost bone benefits after reduced or ceased activity, whereas exercise-induced bone gains have been reported to persist 1–8 years after exercise intervention in pre- or early-pubertal children .
A prospective follow-up of unilateral bone strength gain in the loaded arms of female tennis and squash players demonstrated a maintained benefit, particularly at the shaft sites, 5 years after cessation of their competitive careers . A controlled cross-sectional comparison of the unilateral differences at the humeral shafts of active and retired professional baseball players, in turn, indicated that about half of the bone size (total area) and one-third of the bone strength benefits were maintained lifelong ( Fig. 22.6 ). After throwing activities had ceased, however, the benefits in cortical thickness were gradually lost due to medullary expansion and endocortical trabecularization ( Fig. 22.6 ). Authors concluded that the old adage of “use it or lose it” does somewhat apply to the skeleton, but not entirely, and that PA during youth should be strongly encouraged for lifelong bone health .
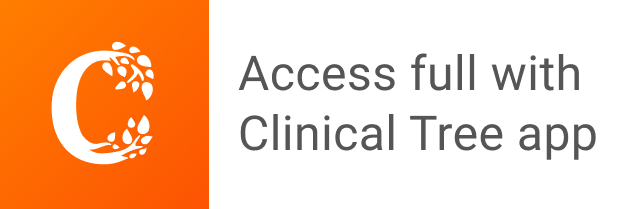