52.1
The bone remodeling system
As transplantation osteoporosis results from alterations in the bone remodeling system, a review of normal bone remodeling is helpful to understanding the pathogenesis of this disease. The bone remodeling cycle is an orderly progression of events in which old, micro-damaged bone is replaced by new, mechanically stronger bone. The complete remodeling cycle at each remodeling site requires approximately 3–6 months. Bone remodeling occurs by two main processes, resorption followed by formation , which take place on both cancellous and cortical bone surfaces. Resorption begins with activation of macrophage precursors to form osteoclasts, giant multinucleated cells that excavate a cavity on the bone surface. Osteoclasts express receptors for receptor activator of nuclear factor-kappaB (RANK) ligand (RANKL), which is produced by osteoblasts and osteocytes, and for calcitonin, prostaglandins (PGs), calcium, and vitronectin (integrin α 1 β 3 ). Approximately 0.05 mm 3 of bone tissue is resorbed by each osteoclast, leaving small resorption pits on the bone surface called Howship’s lacunae in a process that takes approximately 2–3 weeks. A brief rest period called the reversal phase follows. Next, local mesenchymal bone marrow stem cells differentiate into osteoblasts that are attracted to the empty resorption pits where they accumulate as clusters of plump cuboidal cells along the bone surface. Osteoblasts produce the collagenous and noncollagenous proteins that constitute the matrix of the newly formed bone and are responsible for mineralization of the matrix or osteoid after a period of maturation that lasts approximately 20 days. Osteoblasts express receptors for several hormones [thyroid and parathyroid hormones (PTH), estrogens, vitamin D 3 ], cell adhesion molecules (integrins), and cytokines. RANKL, RANK, and osteoprotegerin (OPG) are three members of the tumor necrosis factor ligand and receptor-signaling family that are final mediators of bone resorption . RANKL is expressed in osteoblasts, osteocytes, and bone marrow stromal cells. When sufficient concentrations of macrophage colony-stimulating factor are present, RANKL binds to RANK, which is expressed on the surface of osteoclast lineage cells. This binding results in rapid differentiation of osteoclast precursors in bone marrow to mature osteoclasts, increased osteoclast activity, and reduced apoptosis of mature osteoclasts. RANKL is neutralized by binding to OPG, which is secreted by cells of the osteoblast lineage. Competitive binding of RANKL to either RANK or OPG regulates bone remodeling by increasing (RANK) or decreasing (OPG) osteoclastogenesis. Immunosuppressants exert their effects on bone remodeling by interacting with the RANK/RANKL/OPG system .
In healthy adults, declines in bone mass as a result of remodeling imbalance occur but are small. When bone remodeling becomes “uncoupled,” such that the rate of resorption exceeds the rate of formation, bone loss will occur. This typically happens when the rate of resorption is elevated beyond the capacity of the osteoblasts to restore the original amount of bone volume. However, depressed bone formation can also result in bone loss if resorbed bone, even normal amounts, cannot be replaced. It is very likely that transplantation-related bone loss results from both an increase in the rate of resorption and a decrease in the rate of bone formation .
52.2
Skeletal effects of immunosuppressive drugs
52.2.1
Glucocorticoids
Glucocorticoids (GCs) are a critical component of most posttransplant immunosuppression regimens. Prednisone or methylprednisolone are often prescribed in high doses (50–100 mg of prednisone or its equivalent daily) immediately after transplantation and during episodes of severe rejection, with gradual reduction over weeks to months. Total exposure varies with the organ transplanted, the number and management of rejection episodes, and the practice of individual transplantation programs. These medications are well recognized for their ability to cause osteoporosis.
The mechanisms by which GCs cause bone loss and fractures are outlined in Table 52.1 and have been summarized by several reviews . The predominant effect is an immediate and profound inhibition of bone formation. This results from decreased osteoblast recruitment, differentiation, and synthesis of type I collagen, as well as induction of apoptosis of osteoblasts and osteocytes . Biochemically, these effects are reflected in low levels of circulating osteocalcin, a major noncollagenous bone matrix protein secreted by osteoblasts. Indirect effects of GCs include inhibition of growth hormone secretion and decreased production or bioactivity of certain skeletal growth factors (insulin-like growth factor-1, PGE 2 , and transforming growth factor), actions that also reduce bone formation.
Inhibit bone formation—most important effect |
---|
30% reduction in amount of bone replaced in each remodeling cycle
|
Stimulate bone resorption—effects are minor, limited to first 6–12 months |
|
GCs also increase bone resorption. Their stimulatory effects on resorption are not as profound as their inhibitory effects on formation and are also transitory, generally limited to the first 6–12 months. GCs directly increase osteoclast activity by decreasing osteoblast expression of OPG and increasing expression of RANKL. In turn, osteoclast maturation is increased and osteoclast apoptosis is decreased. GCs indirectly increase resorption through impaired calcium transport across cell membranes, which results in reduced intestinal calcium absorption, increased urinary calcium losses, and negative calcium balance. Secondary hyperparathyroidism may result, although it is unlikely that this plays a major role in the pathogenesis of GC-associated bone loss . GCs also cause hypogonadotropic hypogonadism and reduced secretion of adrenal androgens and estrogens, which may also result in increased bone resorption. These contrasting effects of GCs upon bone formation (decreased) and resorption (increased) prevent osteoblasts from replacing the increased amount of bone resorbed at each remodeling site, and rapid bone loss ensues. GC-induced myopathy may also contribute to bone loss and fractures by altering gravitational forces on the skeleton, reducing weight-bearing activity and mobility, and by increasing the propensity for falls.
While GCs result in significant bone loss in individuals of all races, ages, and both genders, postmenopausal, Caucasian women are at greatest risk for fracture . In these women, GC-related bone loss is superimposed upon that already sustained because of aging and estrogen deficiency. In general, bone loss is most rapid during the first 12 months after transplantation and is directly related to dose and duration of therapy. Areas of the skeleton rich in cancellous bone (ribs, vertebrae, and distal ends of long bones) and the cortical rim of the vertebral body are most severely affected and are the most common sites for fracture.
Recent trends favor more rapid lowering of GC doses after transplantation or rejection episodes, and the use of alternative drugs to treat rejection . However, significant bone loss persists even in patients who have received lower doses of steroids, although it may be less rapid than previously documented . It is also important to note that even rather small doses of GCs are associated with increased fracture risk. In a large retrospective general practice database study, doses of prednisolone as low as 2.5 mg daily were associated with a significant 55% increase in the relative risk of spine fractures; doses of 2.5–7.5 mg daily were associated with a 260% increase in the risk of spine fracture and a 77% increase in the risk of hip fracture . Thus, even with the lower doses of GCs used today in many programs, there is still sufficient exposure in the first year to cause significant bone loss.
52.2.2
Cyclosporines
Cyclosporine A (CsA) is now a standard component of many immunosuppressant regimens. CsA is a small fungal cyclic peptide, the activity of which depends upon the formation of a heterodimer with its cycloplasmic receptor, cyclophilin. This CsA–cyclophilin heterodimer then binds to calcineurin and inhibits the phosphatase activity of calcineurin through interaction with distinct domains on the calcineurin subunit . Calcineurin may regulate both osteoblast and osteoclast differentiation . Although the gene for calcineurin, which is integral to the immunosuppressive action of CsA, has been identified in osteoclasts and extracted from whole rat bone, it does not appear to be altered by CsA administration .
Effects of CsA on bone and mineral metabolism that may contribute to bone loss after organ transplantation have been suggested by multiple animal studies ( Table 52.2 ) . When administered to rodents, albeit in doses higher than those currently used to prevent allograft rejection, CsA caused rapid and severe cancellous bone loss . Histomorphometry revealed a marked increase in bone resorption. Bone formation is increased in CsA-treated animals, in contrast to the effects of GCs, although insufficiently to compensate for the increase in resorption. The stimulatory effects of CsA on osteoclast formation are likely mediated via T lymphocytes . CsA also increases gene expression of osteocalcin and of bone-resorbing cytokines, such as interleukin (IL)-1 and IL-6 . CsA-induced bone loss may be promoted by PTH . Drugs that inhibit bone resorption, including estrogen, raloxifene, calcitonin, and alendronate, prevent or attenuate CsA-induced bone loss in rats . Similarly, 1,25-dihydroxyvitamin D [1,25(OH) 2 D] and PGE 2 also prevent bone loss in CsA-treated rats . Testosterone, however, does not appear to ameliorate bone loss .
Increase expression of bone-resorbing cytokines |
Increase expression of osteocalcin |
Increase bone resorption |
Increase bone formation |
Cause rapid, severe cancellous bone loss |
Effects mediated by T lymphocytes |
PTH may have permissive effect |
Bone loss prevented by antiresorptive agents, 1,25-dihydroxyvitamin D |
In contrast to findings from animal data, studies examining the effects of CsA on the human skeleton have yielded conflicting results. Several have shown that kidney transplant patients receiving CsA in a steroid-free regimen did not lose bone . However, a small study of kidney transplant recipients detected no difference in bone loss between those who received CsA monotherapy and those who received azathioprine plus prednisolone and a prospective study found that cumulative CsA dose was associated with bone loss in the 2 years following transplant, independent of the effect of steroids . In a study of patients on GC-sparing regimens, less than 6 months of GCs after transplant, 27% sustained a fracture, similar to rates in patients on conventional GC-containing regimens .
52.2.3
Tacrolimus (FK506)
FK506, a macrolide that binds to an immunophilin FK-binding protein, blocks T-cell activation in a similar manner to CsA. FK506 has been shown to cause bone loss in the rat model with biochemical and histomorphometric alterations similar to those observed with CsA ( Table 52.2 ) . In humans treated with FK506, rapid bone loss has been documented after both cardiac and liver transplantation . Some studies suggest that FK506 may cause less bone loss than CsA in humans , likely because lower doses of GCs are required for immunosuppression. Whether FK506 confers any benefit over CsA with regard to fracture incidence is not known.
52.2.4
Rapamycin (sirolimus)
Rapamycin is a macrocyclic lactone structurally similar to FK506 and binds to the same binding protein. However, rapamycin induces immunosuppression by inhibiting the response to IL-2, whereas FK506 inhibits secretion of IL-2. When combined with low-dose CsA, rapamycin was bone sparing in rat studies . In an open-label study, markers of bone turnover (N-telopeptide and osteocalcin) were higher in kidney transplant recipients who received CsA compared to rapamycin; unfortunately, bone mineral density (BMD) was not measured, so whether this was associated with a reduced rate of bone loss could not be determined .
52.2.5
Azathioprine, mycophenolate mofetil, and other drugs
Short-term administration of azathioprine is associated with decreases in serum osteocalcin but not with bone loss in animal models . No adverse effects of azathioprine administration alone on bone mass have been reported in human subjects. In the past, azathioprine was frequently used in combination with prednisone and CsA or FK506 to prevent organ rejection. However, it has largely been supplanted by mycophenolate mofetil, which does not have deleterious effects on bone in the rat . The skeletal effects of other immunosuppressant agents such as mizoribine, deoxyspergualin, brequinar sodium, liflunomide, and azaspirane are unclear. A combination of immunosuppressive agents in lower doses likely provides the best strategy for achieving adequate immunosuppression while protecting the skeleton.
52.3
Effects of vitamin D on immunity and graft rejection
52.3.1
Immune effects of vitamin D
Among the many skeletal and extraskeletal sequelae of vitamin D deficiency, the role of vitamin D in regulation of immune function may be of particular consequence among organ transplant patients . This relationship is discussed in detail in a review . Vitamin D potentiates the innate immune system, and studies have shown it to be protective against bacterial infections and tuberculosis . When 1,25(OH) 2 D is produced by monocytes and macrophages in sufficient quantities, it has intracellular antimicrobial effects. In the local environment, it can also interact with and govern the cytokine profiles of activated T and B lymphocytes . The ability of monocytes and macrophages to synthesize 1,25(OH) 2 D is dependent on availability of adequate serum concentrations of 25-OHD (calcidiol). This ability will increase in response to vitamin D supplementation . Conversely in the setting of insufficient 25-OHD, there will be a decrease in local production of 1,25(OH) 2 D and reduced activity against ingested microbes . The antimicrobial actions of 1,25(OH) 2 D also occur in barrier epithelial cells of the skin , gastrointestinal tract , and lungs . Animals treated with calcitriol were able to resist infection with Candida albicans and herpes simplex virus-1 , two common opportunistic infections in transplant patients. In humans the prevalence of upper respiratory tract infections was greater in individuals with lower 25-OHD levels .
52.3.2
Role of vitamin D in allograft rejection
A role of vitamin D in the regulation of immune cell proliferation, differentiation, and responsiveness has been demonstrated . Evidence from animal studies suggests that administration of 1,25(OH) 2 D can prevent acute allograft rejection following liver , kidney , and heart transplantation. While data from human studies are limited, kidney transplant recipients supplemented with calcitriol had fewer episodes of acute cellular rejection , reduced GC requirements , and decreased expression of costimulatory and Human Leukocyte Antigen (HLA)-DR molecules, suggesting a possible mechanism for allograft survival . In one study, patients treated with calcitriol following heart transplantation had a reduction in their requirement for CsA . However, in a clinical trial of heart transplant recipients treated with calcitriol, Shane et al. did not observe a reduction in CsA or prednisone dose . In a retrospective study of cardiac transplant patients, those with lower preoperative 25-OHD had more moderate-to-severe rejection episodes in the first 2 months after transplant . In a clinical setting, patients supplemented with cholecalciferol had fewer rejection episodes . Future prospective studies are needed to elucidate the role of 1,25(OH) 2 D and of parent vitamin D in prevention of graft rejection and infection after transplantation.
52.4
Effect of transplantation on bone and mineral metabolism
52.4.1
Bone loss before transplantation
The majority of individuals with chronic diseases severe enough to warrant organ transplantation have already sustained considerable bone loss ( Table 52.3 ). Most candidates for organ transplantation have one or more accepted risk factors for osteoporosis, including loss of mobility and physical inactivity, poor nutrition, debilitation, and cachexia. These patients are commonly exposed to drugs known to cause bone loss, including GCs, heparin, loop diuretics, and anticonvulsants. Estrogen deficiency contributes to bone loss in postmenopausal women and many chronically ill premenopausal women. Similarly, hypogonadotropic hypogonadism is common in men with chronic illness. Peak bone mass may be low in patients who have had disease since childhood, as is the case with cystic fibrosis (CF) or congenital heart disease. For all of these reasons, when caring for organ transplant candidates it is essential to consider the possibility that bone mass may be reduced before transplantation. Particular issues related to transplantation of specific organs are outlined in the following sections.
Type of transplant | Prevalence before transplantation | |
---|---|---|
Osteoporosis a (%) | Fractures | |
Kidney b | 8–49 | Vertebral: 3%–21% |
Peripheral: 35% | ||
Heart | 4–10 | Vertebral: 18%–50% |
Liver | 8–43 | Vertebral: 20%–25% |
Lung | 30–67 | Vertebral: 14%–49% |
a Accepted definitions included bone mineral density (BMD) (by dual X-ray absorptiometry) of the spine and/or hip with Z score—2 or less or T score—2.5 or less.
b Definition of osteoporosis also included BMD of predominantly cortical sites such as the femoral shaft or proximal radius that are adversely affected by excessive PTH secretion.
52.4.2
Kidney and kidney–pancreas transplantation
52.4.2.1
Skeletal status before transplantation
Many factors contribute to the complex form of bone disease known as renal osteodystrophy in patients with severe chronic kidney disease (CKD) or end-stage renal disease (ESRD). These include disturbances in calcium and phosphate metabolism, decreased calcitriol synthesis, increased synthesis and secretion of PTH, metabolic acidosis, and defective bone mineralization . Some form of renal osteodystrophy is seen in almost all patients who undergo kidney transplantation. A given individual may have high bone turnover due to hyperparathyroidism with or without osteitis fibrosa, low turnover or adynamic bone disease, osteomalacia, or “mixed” renal osteodystrophy, a combination of one or more of the aforementioned lesions. Type I diabetes and systemic lupus erythematosus, diseases that commonly result in ESRD, can adversely affect the skeleton. Hypogonadism secondary to uremia is common. Several drugs used routinely in the management of patients with renal disease, such as loop diuretics and calcium-containing phosphate binders, can also affect bone and mineral metabolism. In addition, some kidney transplant candidates may have had previous exposure to GCs or CsA as therapy for immune complex nephritis or other diseases and thus may already have sustained significant bone loss prior to transplantation. Measurement of BMD by dual-energy X-ray absorptiometry (DXA) has important limitations in patients with ESRD and does not distinguish among the various types of renal osteodystrophy, and there is evidence that DXA does not discriminate between patients with and without fractures . However, in a study of patients with stage 3–5 CKD , DXA measurements at the lumbar spine (LS), total hip (TH), 1/3 radius, and ultradistal radius did discriminate fracture status. Out of all the DXA and microarchitectural variables measured, ultradistal radius DXA was best able to discriminate fracture status. Several cross-sectional studies have documented osteoporosis in a significant proportion of chronic dialysis patients ( Table 52.3 ) .
The risk of fracture in patients with ESRD is greatly elevated, estimated at 4.4–14 times greater than that of the general population . Vertebral fractures were present in 21% of Japanese hemodialysis patients . In one study, 34% of 68 hemodialysis patients had a history of previous fracture . In a prospective study the incidence of fractures was 0.1 fractures per dialysis year in patients with osteitis fibrosa and 0.2 fractures per dialysis year in patients with adynamic bone disease . In patients with moderate-to-severe CKD who do not yet require dialysis, hip fractures are twofold more common than in those with normal kidney function .
Risk factors for low BMD and fractures among patients with CKD and ESRD include Caucasian race, female gender, hyperparathyroidism, adynamic bone disease, secondary amenorrhea, type I diabetes, older age, duration of dialysis, peripheral vascular disease, prior kidney transplant , and diabetic nephropathy .
52.4.2.2
Prevalence of osteoporosis in kidney transplant recipients
Low BMD measurements have been reported in many cross-sectional studies of patients who have undergone kidney transplantation ( Table 52.4 ) , although again the prognostic significance of low BMD in this group is unclear. For example, LS BMD was below the fracture threshold in 23% of 65 renal transplant recipients studied a mean of 4 years after transplantation ; female gender, postmenopausal status, and cumulative prednisone dose were independent predictors of low BMD. Similarly, LS BMD was more than 2 standard deviations (SD) below age- and sex-matched controls ( Z score ≤−2.0) in 41% of patients assessed between 6 and 195 months after renal transplantation and was directly related to increasing time since transplantation and circulating PTH concentration. In another study, advanced age, female gender, and diabetic nephropathy were associated with osteoporosis . LS and femoral neck (FN) bone density were more than 2 SDs below age- and sex-matched controls in 29% and 11%, respectively, of 70 kidney transplant recipients studied an average of 8 years after transplantation , and low BMD was particularly prevalent in women. In a study of male renal transplant recipients, 30% had osteoporosis at the hip or LS, 41% including the distal radius; only 17% had normal BMD. Bone resorption markers were elevated in half of the cohort . Other studies have shown similar results .
Type of transplant | Prevalence after transplantation | Bone loss: first posttransplant year | Fracture incidence | |
---|---|---|---|---|
Osteoporosis a (%) | Fractures | |||
Kidney b | 11–56 | Vertebral: 3%–29% | Spine: 4%–9% | Vertebral: 3%–10% |
Peripheral: 10%–50% | ||||
Peripheral: 11%–22% | Hip: 8% | |||
Heart | 25–50 | Vertebral: 22%–35% | Spine: 2.5%–8% | 10%–36% |
Hip: 6%–11% | ||||
Liver | 30–46 | Vertebral: 29%–47% | Spine: 0%–24% | Vertebral: 24%–65% |
Hip: 2%–4% | ||||
Lung | 57–73 | 42% | Spine: 1%–5% | 18%–37% |
Hip: 2%–5% | ||||
Bone marrow | 4–15 | 5% | Spine: 2%–9% | 1%–16% |
Hip: 6%–11% |
a Accepted definitions included bone mineral density (BMD) (by dual X-ray absorptiometry) of the spine and/or hip with Z score —2 or less or T score —2.5 or less.
b Definition of osteoporosis also included BMD of predominantly cortical sites such as the femoral shaft or proximal radius that are adversely affected by excessive parathyroid hormone (PTH) secretion.
52.4.2.3
Bone loss after kidney transplantation
Prospective longitudinal studies have documented high rates of bone loss after kidney transplantation ( Table 52.4 ), particularly during the first 6–18 months. Julian et al. were the first to report that LS BMD decreased by 7% at 6 months and by 9% at 18 months after transplantation . This pattern of bone loss, in which the rate is greatest during the first 6 months after transplantation and predominates at sites of cancellous bone, has been confirmed in several prospective studies . Estimates of bone loss at the LS vary between 3% and 10%. There may be a gender difference in the site at which bone is lost ; men lose more bone at the proximal femur than women in the first few months after transplantation. Since the early 2000s many kidney transplant programs have shifted to calcineurin inhibitor-based regimens, lowering GC doses or eliminating them entirely after only a few days of high-dose treatment . This shift in management has been associated with reduced rates of bone loss after kidney transplantation although this finding has not been uniform .
The pathogenesis of bone loss after renal transplantation is complex. The majority of studies have found that GC dose is directly associated with bone loss. Risk may be lower in men and premenopausal women, and higher in postmenopausal women. Some literature suggests that CsA plays a role in the pathogenesis of the high-turnover state often seen in renal transplant recipients by 1 year after renal transplantation.
52.4.2.4
Bone histology after kidney transplantation
Hyperparathyroidism is the most common lesion on bone biopsy prior to transplantation. However, by 6 months after transplantation, GC effects of osteoblast dysfunction and decreased mineral apposition predominate . Bone biopsy results are more heterogeneous in long-term transplant recipients and include osteoporosis, osteomalacia, and osteitis fibrosa. Reduced mineral apposition rate, longer mineralization lag time, and normal bone formation rate were commonly observed .
52.4.2.5
Fracture after kidney transplantation
Fractures are very common after renal transplantation ( Table 52.4 ) and typically involve appendicular sites (feet, ankles, long bones, hips) more than axial sites (spine, ribs) . In one study, nonvertebral fractures were far more prevalent in renal transplant recipients than in the normal population, five times more common in men aged 25–64 years, 18 times more common in women aged 25–44 years, and 34 times more common in women aged 45–64 years . Vertebral fractures have been reported in 3%–10% of nondiabetic patients after renal transplantation . In a cohort study of 101,039 subjects, kidney transplant patients had a 34% greater risk of hip fracture than those who remained on dialysis . Prevalent fractures, vertebral or appendicular, were identified in 24% of long-term kidney transplant subjects .
Fractures are common in patients who receive kidney or kidney–pancreas transplants for diabetic nephropathy . In a 3-year retrospective study of 35 kidney–pancreas recipients, approximately half had sustained from one to three symptomatic, nonvertebral fractures . Pretransplant diabetes was associated not only with a significant increase in fracture after transplantation in a nested case–control study of predominantly kidney but also heart, liver, and lung transplant recipients . This relationship persisted after controlling for several potential confounders, including GC use. However, data suggest that patients with type I diabetes who undergo kidney–pancreas transplants have a lower rate of fractures than those who undergo only kidney transplant; fracture risk was 36% lower in men who had kidney–pancreas transplants, although this relationship was not seen among women . Fractures are reduced in patients who have early corticosteroid withdrawal. In one study based upon the US Renal Data System, incidence of fractures requiring hospitalization was 31% lower among patients who only received steroids in the immediate posttransplant period .
52.4.2.6
Bone turnover and mineral metabolism after kidney transplantation
After kidney transplantation, several fairly consistent changes in biochemical indices of mineral metabolism and bone turnover occur . PTH concentrations, which are usually high before transplantation, typically remain elevated and may never completely normalize . Because of residual parathyroid hyperplasia, elevated PTH, hypercalcemia, and hypophosphatemia are common during the first few months after transplant. Thereafter, calcium levels tend to decrease into the normal range. Data suggest that posttransplant hypophosphatemia may also be related to persistent elevations in fibroblast growth factor-23 . In most patients, these biochemical abnormalities are mild and resolve within the first year. In long-term transplant recipients, persistent elevations in PTH may be associated with reduced hip BMD . Calcitriol levels may remain low for 18 months after transplantation . Calcitriol production by the transplanted kidney may be inadequate to suppress PTH secretion by hyperplastic parathyroid tissue , and treatment with calcitriol may prevent hyperparathyroidism after renal transplantation . Vitamin D deficiency is common and severe in patients after kidney transplantation . Transplant patients have significantly lower levels of 25-OHD than age-matched controls . In one study, one-third of patients had undetectable 25-OHD values and mean 25-OHD was 10 ng/mL .
52.4.3
Cardiac transplantation
52.4.3.1
Skeletal status before transplantation
Several risk factors common in patients with end-stage heart disease may predispose to bone loss prior to transplantation, including use of tobacco, alcohol, and loop diuretics; physical inactivity; hypogonadism; and anorexia, which may contribute to dietary calcium deficiency. Hepatic congestion and prerenal azotemia may cause mild secondary hyperparathyroidism and further contribute to abnormal mineral metabolism. Although many patients awaiting cardiac transplantation may have normal bone density, approximately 4%–10% fulfill World Health Organization (WHO) criteria for osteoporosis ( Table 52.3 ) , and one study reported a prevalence of 23% .
52.4.3.2
Prevalence of osteoporosis in heart transplant recipients
Osteoporosis and fractures cause significant morbidity after cardiac transplantation. In cross-sectional studies the prevalence of vertebral fractures ( Table 52.4 ) ranges between 18% and 50%. Moderate-to-severe bone loss occurs in a substantial proportion of subjects at both LS and the FN . In a cross-sectional study, osteopenia or osteoporosis ( T score <−1.0) were found in 26% of long-term cardiac transplant recipients at the LS and 66% at the FN . Adults who receive cardiac transplants as adolescents have significantly lower BMD at LS, FN, and distal radius than age-matched controls, likely related to a failure to achieve peak bone mass .
52.4.3.3
Bone loss after heart transplantation
The pattern of bone loss after cardiac and kidney transplantation is similar. Longitudinal studies have documented rates of bone loss ranging from 2.5% to 11%, predominantly during the first 3–12 months after transplantation ( Table 52.4 ) . Bone loss at the hip is equal to or greater than bone loss at the LS . Further, while bone loss at the LS slows or stops after the first 6 months, FN bone loss continues during the second half of the first year after transplantation . There are limited longitudinal data on the pattern of bone loss after the first year. Our data suggest that the rate of bone loss slows or stops in the majority of patients, with some recovery at the LS noted during the third year of observation . Bone loss also slows at the hip after the first year; however, in contrast to the spine, there has been no significant recovery by the fourth posttransplant year. The results of one study suggest that there may be less bone loss than suggested in literature from the 1980s and early 1990s . Together, these findings indicate that factors other than GCs are important determinants of bone loss after cardiac transplantation, since GCs affect the predominantly cancellous bone of the vertebrae to a greater extent than other sites, and more substantial and lasting losses occur at the hip, a site with more cortical bone.
52.4.3.4
Fracture after heart transplantation
Fragility fractures most often occur during the phase of rapid bone loss in the first year following transplant ( Table 52.3 ). In one prospective observational study, 36% of patients (54% of the women and 29% of the men) sustained one or more fractures of the vertebrae, ribs, and hip in the first year despite daily supplementation with calcium (1000 mg) and vitamin D (400 IU) . The majority of fractures occurred during the first 6 months; mean time to first fracture was 4 months. Increased fracture risk tended to be associated with female gender and lower BMD prior to transplant. In men the rate of bone loss after transplantation rather than the pretransplant bone density was associated with fracture risk. Fractures were not predicted by pretransplant BMD or any other demographic or biochemical parameter. Many patients who had fractures had normal BMD prior to transplant . Similarly, two European studies of cardiac transplant recipients reported an incidence of vertebral fracture during the first 3 years of 30%–33% . The risk of a vertebral fracture was higher in those patients who had LS T scores below −1.0 (hazard ratio 3.1) .
In a more recent interventional study, patients who received only calcium and vitamin D had a vertebral fracture incidence during the first year posttransplant of only 14% . Similarly, in a prospective study of untreated patients, only 12% had fractures , suggesting fracture rates may be lower than in the past. Despite these results, clinical experience suggests that fractures remain a common and potentially devastating complication of heart transplantation. A complete bone evaluation, including BMD measurements before or immediately after transplantation, and consideration of treatment to prevent bone loss and fractures are warranted in all patients regardless of age, sex, or pretransplant bone density.
52.4.3.5
Bone turnover and mineral metabolism after heart transplantation
We reported that severe vitamin D deficiency was extremely common among heart and liver transplant recipients at the time of transplantation; 91% of patients had vitamin D insufficiency (25-OHD 20 to <32 ng/mL), 55% had deficiency (25-OHD 10 to <20 ng/mL), and 16% had severe deficiency (25-OHD <10 ng/mL) . After cardiac transplantation, there is a sustained increase in serum creatinine and decrease in 1,25(OH) 2 D . Serum testosterone concentrations decrease in men and may recover by the sixth posttransplant month . The etiology of low testosterone is multifactorial. Testosterone levels are lowest in the first month following transplant and reflect suppression of the hypothalamic–pituitary–gonadal axis by prednisone . Low testosterone at 1 and 2 years after transplantation may result from of primary gonadal failure . There is a rapid increase in markers of bone resorption (hydroxyproline and pyridinium cross-link excretion) during the first 3 months with return to baseline levels by the sixth month . In contrast, serum osteocalcin falls precipitously. This biochemical pattern suggests that the early posttransplant period is associated with uncoupling of formation from resorption, with restitution of coupling when GC doses are lowered. This period of uncoupling is associated with the most rapid bone loss and highest fracture incidence. Later in the posttransplant period, there is also evidence for a high bone turnover state perhaps due to CsA, characterized by elevations in both serum osteocalcin and urinary excretion of resorption markers . Increased bone turnover may partially reflect secondary hyperparathyroidism related to renal impairment . CsA-induced renal insufficiency may be an important cause of altered bone metabolism in long-term cardiac transplant recipients.
52.4.4
Liver transplantation
52.4.4.1
Skeletal status before transplantation
Multiple risk factors predispose patients with end-stage liver disease to low BMD before and increased fracture rates after transplantation . Many patients listed for liver transplantation have prevalent osteoporosis ( Table 52.3 ), as evidenced by low BMD and fragility fractures . Osteoporosis and abnormal mineral metabolism have been described in patients with liver failure from multiple etiologies, including alcoholic liver disease, hemochromatosis, steroid-treated autoimmune chronic active hepatitis, postnecrotic cirrhosis, and particularly in chronic cholestatic liver diseases such as biliary cirrhosis . In a study of 58 patients with cirrhotic end-stage liver disease referred for liver transplantation , 43% had osteoporosis (defined as Z score >2 SD below age-matched controls or presence of vertebral fractures). Serum 25-OHD, 1,25(OH) 2 D, intact PTH, testosterone (in male patients), and osteocalcin (a marker of bone formation) were lower and urinary hydroxyproline excretion (a marker of bone resorption) was higher in cirrhotic patients than controls. In another study of 56 liver transplant recipients, 23% had osteoporosis that antedated transplantation . In a larger study of 360 liver transplant candidates, 38% had osteoporosis and 39% had osteopenia .
Patients with primary biliary cirrhosis have low serum osteocalcin and low bone formation by histomorphometry . While decreased bone formation and increased bone resorption markers have been reported in patients with chronic liver disease , the utility of collagen-related markers of bone turnover has been questioned. In fibrotic liver diseases the synthesis of type I collagen is markedly increased. Guanabens et al. have found that collagen-related bone turnover markers appear to be influenced by liver, rather than bone, collagen metabolism and therefore do not reflect skeletal turnover in patients with liver disease . Serum osteocalcin and tartrate-resistant acid phosphatase may be more valid markers of bone remodeling activity in this clinical situation.
52.4.4.2
Bone loss and fracture after liver transplantation
Osteoporosis is prevalent after liver transplantation as well . The natural history of bone loss following liver and cardiac transplantation appears similar . Rates of bone loss and fracture vary considerably after liver transplantation ( Table 52.3 ). Very high rates were reported in studies published before 1995 , in which LS BMD fell by 2%–24%, primarily in the initial few months after liver transplantation. More recent studies have reported less bone loss. Keogh et al. reported that FN BMD fell by 8% and LS BMD by 2% after liver transplantation . Ninkovic et al. found only a 2.3% loss at the FN, with preservation of LS BMD 1 year after liver transplant . Floreani et al. found increases in BMD at 1 year . In general, bone loss appears to stop after 3–6 months with gradual improvement by the second and third posttransplant years. Eastell et al. have reported that bone mass recovers and bone histology normalizes with increasing survival time after transplantation , and other investigators have shown that there is improvement in BMD in long-term liver transplant recipients . This finding has not been uniform, however, and other studies have found continued losses rather than recovery .
Smallwood et al. reported in a cross-sectional study that lower bone mass following liver transplant was associated with older age, female gender, cholestatic liver disease, and higher prednisone dose . One retrospective study found that women who received cumulative GC doses that exceeded 3500 mg had lower FN BMD at 1 and 2 years following liver transplant than other patients . In a longitudinal study of 360 patients after liver transplant, Guichelaar et al. found higher rates of LS bone loss in patients with primary sclerosing cholangitis, current smokers, younger age, higher baseline BMD, shorter duration of liver disease, and ongoing cholestasis .
Fracture incidence is greatest in the first year after transplantation and ranges from 24% to 65%, the latter in a small study of women with primary biliary cirrhosis. The most common fracture sites are the vertebrae and ribs. Again, fracture rates appear to be considerably lower in more recent studies . Whether type of liver disease at baseline predicts fractures is controversial. Some authors report more bone loss and fractures in patients with primary sclerosing cholangitis and alcoholic cirrhosis . GC exposure and markers of bone turnover do not reliably predict bone loss or fracture risk. In prospective studies, older age and pretransplant BMD at the FN and LS were predictive of posttransplant fractures . Vertebral fractures prior to transplant may also predict posttransplant vertebral fractures . In patients who undergo repeat transplantations, those with primary biliary cirrhosis and with previous fragility fractures appear to be at increased risk. Risk of fractures may persist over the long term in patients with prolonged survival; in one study of patients who survived more than 15 years after liver transplantation, 49% had osteoporosis and 30% had sustained vertebral fractures .
52.4.4.3
Bone turnover and mineral metabolism after liver transplantation
There are few studies of calciotropic hormone levels and bone turnover markers after liver transplantation. Compston et al. reported a significant rise in serum intact PTH during the first 3 months after liver transplantation, although values did not exceed the upper limit of the normal range . Significant increases in PTH during the first 3–6 months after transplant have also been observed by other authors , although several early studies reported intact PTH levels in the normal range in liver transplant recipients . In our study that assessed 25-OHD at the time of transplantation, we found that liver transplant recipients had significantly lower vitamin D levels than heart transplant recipients, likely because of disease-related factors such as malabsorption, and impaired hepatic 25-hydroxylation of vitamin D . It is also possible that reduced hepatic production of vitamin D-binding protein could lead to an apparent decrease in total (bound and free) serum 25-OHD, but that free levels may be normal.
Bone turnover, measured by markers of bone formation and resorption (osteocalcin and carboxyterminal peptide of type I collagen), is higher in liver transplant recipients than in normal controls in most , though not all studies . OPG and RANKL levels are significantly elevated in the first 2 weeks following liver transplant .
As most liver transplant recipients receive both GCs and calcineurin inhibitors during the early posttransplant period, the independent role of each agent is difficult to assess. The mechanism of bone loss after liver transplantation was investigated in a histomorphometric study of 21 patients before and 3 months after transplantation. Before transplantation, a low turnover state was observed, with decreased osteon wall width and erosion depth. Postoperatively, a high-turnover state was observed, with increased formation rates and activation frequency, and a trend toward higher indices of resorption . High turnover may have been related to the concomitant increase in PTH concentrations or alternatively to calcineurin inhibitors. Together, the biochemical and histomorphometric data suggest therefore that low bone turnover observed in many patients with liver failure converts to a high-turnover state that persists indefinitely after liver transplantation.
52.4.5
Lung transplantation
52.4.5.1
Skeletal status before lung transplantation
Bone loss and osteoporosis are extremely common among patients with end-stage lung disease, and may be related to many factors, including hypoxemia, tobacco use, and prior GC therapy ( Table 52.3 ) . CF, a disease that often results in lung transplantation, is associated with osteoporosis and fractures due to disease-specific factors such as pancreatic insufficiency, vitamin D deficiency, and calcium malabsorption and hypogonadism . A markedly increased rate of all fractures and severe kyphosis has been reported in adults with CF . We have observed that vitamin D deficiency is extremely common in CF patients, and bone density was significantly lower in vitamin D-deficient patients despite supplementation . In cross-sectional studies, low bone mass and osteoporosis were present in 45%–86% of candidates for lung transplantation; low BMD was related to higher GC exposure . Vertebral fracture prevalence was 29% in patients with emphysema and 25% in patients with CF . Low bone mass is also common in patients with primary pulmonary hypertension prior to lung transplantation; in a retrospective study, 72% had osteopenia at the LS and 61% at the FN; BMD at the FN correlated with pulmonary vascular resistance, functional measures, and walking distance . A cross-sectional study of patients with diffuse parenchymal lung disease presenting for lung transplantation found that 57% had osteopenia and 13% had osteoporosis. Low BMD was associated with lower body mass index, and Hispanic ethnicity .
52.4.5.2
Bone loss and fracture after lung transplantation
There are few prospective data on patients after lung transplantation and most studies are very small ( Table 52.3 ). A study of 12 patients demonstrated a mean 4% decrease in LS BMD during the first 6 months despite supplementation with calcium 1000 mg and vitamin D 400 IU daily . Two men sustained multiple vertebral fractures. In another study of 28 patients, both LS and FN BMD decreased by approximately 5% during the first 6–12 months after lung transplantation. Fractures developed in 18% . In a retrospective analysis of 33 lung transplant recipients who had survived at least 1 year posttransplant, BMD was markedly decreased and 42% had vertebral fractures . In a 10-year follow-up study of lung transplant recipients, only 28 (29%) patients survived. Of those, 11% had prevalent osteoporotic fractures . In our experience, as many as 37% of lung transplant recipients suffer fragility fractures and significant bone loss during the first posttransplant year despite antiresorptive therapy .
Risk factors for fracture and bone loss after transplant include female gender, low pretransplant LS BMD, pretransplant GC therapy, and high bone turnover after transplantation. Bone loss correlates with GC dose in some , but not all, studies . Bone turnover markers are elevated following lung transplant. Posttransplant bone biopsies of CF patients demonstrate increased osteoclastic and decreased osteoblastic activity .
52.4.6
Bone marrow transplantation
The prevalence and indications for bone marrow transplantation (BMT) are increasing in recent years. Due to myeloablative therapy (alkylating agents and/or total body irradiation) that patients receive prior to transplant, they commonly develop profound and frequently permanent hypogonadism, which can result in bone loss. After transplantation, patients may receive GCs, methotrexate, and/or CsA. Osteoporosis after allogenic BMT is related to several factors, including the effects of treatment and effects on the stromal cell compartment of the bone marrow . Kelly et al. first reported low BMD after BMT . Subsequently, several cross-sectional studies have confirmed low total body BMD or bone mineral content (by DXA) and low LS BMD (by computed tomography) in BMT recipients ( Table 52.4 ). In one study, only patients who were younger than 18 years at the time of transplantation were affected, perhaps because of a failure to achieve optimal bone mass and smaller bone size . Two studies have documented that bone mass is low in hypogonadal women after BMT and that hormone replacement therapy is associated with significant increases in BMD .
There are limited prospective data on bone loss after BMT. In a study of nine adults undergoing 6 months of high-dose GC and CsA therapy for graft-versus-host disease (GVHD), significant LS bone loss occurred . Ebeling et al. found that low BMD antedates BMT, particularly in subjects with prior GC exposure. Posttransplant bone loss was particularly severe in patients who underwent allogenic BMT, likely because of their increased propensity for GVHD . Valimaki et al. followed patients for 6 ( n =44) and 12 months ( n =36) after allogenic BMT. There was no discernable difference in rates of bone loss among patients who received calcium and vitamin D compared to those who received calcitonin. In an analysis of the combined treatment groups, BMD decreased by approximately 6% at the LS and 7% at the FN . Kang et al. found that BMD decreased by 2.2% at the LS and 6.2% at the FN during the first year after BMT . Similarly, Kashyap et al. found that LS BMD decreased by 3.0% and FN BMD by 11.6% during the first 12–14 months . While there appears to be little bone loss after the first year following BMT , the significant bone loss that occurs at the FN does not appear be regained .
Bone turnover markers demonstrate decreased formation and increased resorption during the first 3 months, a pattern consistent with uncoupling of formation from resorption, similar to changes seen after other types of organ transplantation. After 3 months, there is recovery of bone formation markers and elevated turnover persists during the latter half of the year . After autologous BMT, rates of FN bone loss are lower, about 4%, but the losses persist for as long as 2 years. In contrast, LS BMD may return to baseline .
Cellular or cytokine-mediated abnormalities in bone marrow function may affect bone turnover and BMD after BMT . Many factors reduce osteoblastic differentiation, including high-dose chemotherapy, total body irradiation, and treatment with GCs and/or CsA. Colony-forming units-fibroblasts (CFU-f) are reduced for up to 12 years following BMT . Long-term survivors after BMT may have persistent abnormalities in bone turnover and vitamin D .
Avascular necrosis (AVN) is a common complication of BMT, occurring in 10%–20% of allo-BMT survivors, at a median of 12 months posttransplant . The most important risk factor for the development of AVN is GC treatment of chronic GVHD. AVN may be related to decreased numbers of bone marrow CFU-f in vitro, but it does not appear to be related to BMD .
52.4.7
Evaluation and management of osteoporosis in patients awaiting transplantation
52.4.7.1
Evaluation
As there are now substantial data documenting the high prevalence of bone disease in candidates for all types of transplantation, all patients should be evaluated for bone disease before transplantation so that potentially treatable abnormalities of bone and mineral metabolism may be addressed and the bone health optimized preoperatively ( Table 52.5 ). An assessment of risk factors for osteoporosis should be conducted, including history of adult low-trauma fractures, medical conditions (thyrotoxicosis, renal disease, rheumatological, and intestinal disorders), unhealthy lifestyle choices (physical inactivity, dietary calcium and vitamin D deficiency, excessive caffeine and alcohol intake, tobacco use), exposure to certain drugs (diphenylhydantoin, lithium, loop diuretics, GCs, prolonged high-dose heparin, thyroid hormone), and a family history of osteoporosis. Men should be evaluated for hypogonadism. On physical exam, physicians should look for signs of hypogonadism, thyrotoxicosis, and Cushing syndrome. Risk factors for falling (impaired vision, hearing, balance, and muscle strength, psychotropic drugs) should also be assessed.
In all candidates |
Assess risk factors for osteoporosis, including menstrual/menopausal history in women, history of low-trauma fractures |
Measure bone densitometry (BMD) of spine and hip by DXA |
Obtain thoracic and lumbar spine radiographs |
If BMD testing reveals osteoporosis or if there are prevalent vertebral or nonvertebral fractures |
Serum electrolytes, BUN creatinine, calcium, PTH, 25-hydroxyvitamin D, thyroid function tests (see text) |
In men, serum total and/or free testosterone, with follow-up FSH, and LH if testosterone is low |
Urine for calcium and creatinine |
It is critical to measure BMD of the spine and hip before transplantation. Radiographs of the thoracic and LS should also be performed to evaluate the presence of prevalent fractures, which are associated with an increased risk of future fracture. If the BMD is normal and supplementation with calcium and vitamin D is planned, numerous biochemical tests are unnecessary. However, if the pretransplant BMD is low, a thorough biochemical evaluation can alert the physician to the etiology of low bone mass and guide appropriate targeted therapy. For patients with low pretransplant BMD, the biochemical evaluation should include a chemistry panel (serum electrolytes, creatinine, calcium, phosphorus, alkaline phosphatase), thyroid function tests, intact PTH, and serum 25-OHD. To assess bone turnover, markers of bone formation (serum osteocalcin and bone-specific alkaline phosphatase) and resorption (C- or N-telopeptide excretion) can also be measured. In men, total and free testosterone, follicle-stimulating hormone, and luteinizing hormone concentrations should be obtained.
While patients are on the waiting list for transplantation, rehabilitation therapy should be prescribed, as tolerated, to maximize conditioning and physical fitness. All transplant candidates should receive the recommended daily allowance (RDA) of vitamin D (400–800 IU), or as necessary to maintain the serum 25-OHD level above 30 ng/mL (80 nmol/mL), and elemental calcium (1000–1200 mg, from combined dietary sources and supplements). Calcium citrate is preferred as many of these patients take proton pump inhibitors before or after transplantation, which can reduce intestinal calcium absorption. Hypogonadal men should also be offered testosterone replacement. Generally accepted guidelines for gonadal hormone replacement should apply to these patients.
Although pretransplant BMD does not reliably predict fracture in individual patients, low pretransplant BMD likely increases fracture risk, particularly in postmenopausal women. Individuals awaiting transplantation who meet WHO criteria for the diagnosis of osteoporosis ( T score <−2.5), osteopenia, or low bone mass ( T score between −1.0 and −2.5) should be evaluated and treated similarly to others with, or at risk, for osteoporosis ( Table 52.5 ).
Patients who are found to have osteoporosis before transplantation should receive treatment, typically antiresorptive therapy with a bisphosphonate. Teriparatide or abaloparatide can be considered for patients with very low BMD or fractures if they do not have hyperparathyroidism. The pretransplant waiting period is often long enough (1–2 years) for significant improvement in BMD before transplantation. Patients with renal osteodystrophy should be managed in accordance with accepted clinical guidelines . A discussion of this topic is beyond the scope of this chapter.
After transplantation, monitoring serum and urine indices of mineral metabolism is useful to detect developing conditions that may contribute to bone loss (vitamin D deficiency or renal insufficiency with secondary hyperparathyroidism). However, if pharmacologic doses of vitamin D or its active 1-hydroxylated metabolites are used, serum (and urinary) calcium must be monitored frequently in order to detect hypercalcemia or hypercalciuria. Measurement of BMD should be performed annually for the first 2 years, with continued long-term annual measurements for patients who remain on GCs. Transiliac crest bone biopsy largely remains a research tool. However, histomorphometric studies would be very helpful in confirming theories of the pathogenesis of transplantation osteoporosis. In kidney transplant recipients, bone biopsy provides valuable clinical information, particularly regarding the presence of adynamic bone disease, prior to or during bisphosphonate treatment.
52.5
Prevention and treatment of transplantation osteoporosis
The major principles of transplantation osteoporosis that have been demonstrated consistently after kidney, liver, heart, lung, and BMT should be used to guide therapy of patients. They are as follows:
- •
Rates of bone loss are most rapid immediately after transplantation.
- •
Fractures also occur very early in the posttransplant period, sometimes within only a few weeks of grafting.
- •
Fragility fractures develop in patients with both low and normal pretransplant BMD.
- •
Prevention of rapid bone loss during the first few months after transplantation is likely a more effective strategy to reduce morbidity from fractures than initiation of therapy after fractures have occurred.
- •
Preventive strategies should be instituted immediately after transplantation both in patients with normal pretransplant BMD and those with low BMD who have not been treated previously ( Table 52.6 ).
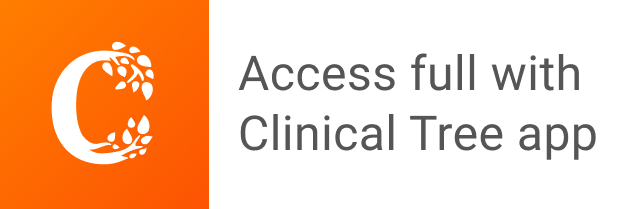