25.1
Introduction
Patients and many physicians still often misperceive osteoporosis as a typically female disorder. However, the male:female gender ratio for osteoporotic fractures is only about 1:1.6 , and for hip fractures 1:2 . Still, gender and, in particular, estrogen deficiency following menopause is one of the strongest clinical risk factors for osteoporosis . Unfortunately, the so-called treatment gap, that is, the lack of recognition and treatment of osteoporosis, is even larger in men than in women . As a consequence, male osteoporosis represents an important public health issues, with considerable economic impact as well as a high personal burden for the affected men and their families. This chapter on osteoporosis in men will highlight recent advances as well as similarities and differences compared to postmenopausal osteoporosis.
25.2
Epidemiology
25.2.1
Prevalence of male osteoporosis
In US adults aged 50 years or older, the prevalence of osteoporosis [defined as a bone mineral density (BMD) T-score by dual-energy X-ray absorptiometry (DXA)] at either the lumbar spine or femoral neck from NHANES 2013–14 data was 16.5% in women and 5.1% in men, and for osteopenia (T-score between −1.0 and −2.5) 52.6% in women versus 35.6% in men . The prevalence of these conditions is lower in Blacks and highest in Asians in both genders (for osteoporosis in Asians in the United States: 38.8% in women versus 6.5% in men) .
Each standard deviation reduction in BMD is associated with about a twofold greater hazard of any fracture and a threefold greater hazard of hip fracture, and these hazard ratios may be even greater in older men than in postmenopausal women .
When low-trauma fractures and an increased FRAX score are considered as criteria (as proposed by the US National Bone Health Alliance and other societies), osteoporosis can be diagnosed in 29.9% of women and 16% of men aged 50 years and older . This prevalence increases from 5.5% in men versus 10.9% in women aged 50–59 years, to 46.3% in men and 77.1% in women aged 80 years and older .
25.2.2
Fracture incidence and burden of male osteoporosis
The burden of osteoporotic fractures in men is considerably less than in women but is still substantial. A systematic review estimated that of the 9 million fractures occurring in the year 2000 worldwide, 39% occurred in men, including 30% of hip, 39% of clinical vertebral fractures, 25% of humerus, and 20% of forearm fractures . In the United States, it has been estimated that in the year 2005, 29% of osteoporotic fractures occurred in men and 71% in women (2.45 female:male ratio) .
The lifetime risk of osteoporotic fractures after age 50 in certain high-risk Caucasian populations has been estimated at 20%–25% in men, compared to 45%–55% in women . Consequently, osteoporotic fractures in men account for around 30% of acute care costs (in the United States: 25% ), estimated in the United States at $ 4.1 billion, in Canada at CAD 489 million , and in Australia at AUD 646 million . In Taiwan the number of admissions and total medical costs for osteoporotic fractures are even greater in men compared to women .
The corollary of the lower risk of osteoporosis and fractures in men is that those men who do sustain a first osteoporotic fracture seem to have a greater relative and thus similar absolute risk of additional osteoporotic fractures as women . In other words, there is a gender difference in the risks of osteoporosis and a first fracture (lower in men), but the risk of refracture is similar in men and women.
25.2.2.1
Risk of mortality
The relative risk of mortality is increased after all osteoporotic fractures in the elderly but is highest following hip fractures, and is generally greater in men compared to women (although the gender difference in mortality after a hip fracture or any fracture is not statistically significant) . Older men also have more comorbidities than women, but comorbidities account for only a small proportion of the excess mortality after fractures . This can be regarded as a sign of greater underlying frailty in those men who do develop osteoporosis, because the excess mortality extends more than 10 years after the injury . Still, the greatest difference is the much higher risk of in-hospital and early mortality in men ( Fig. 25.1 ).
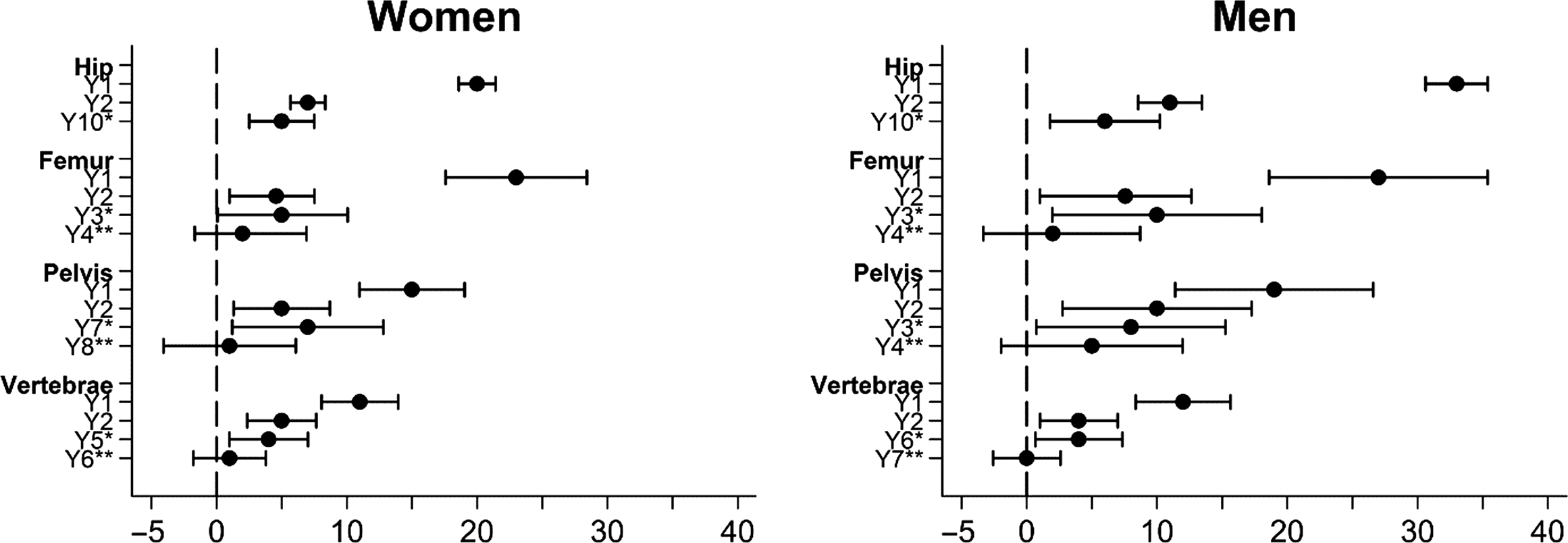
25.2.2.2
Secular trends
Important secular changes in fracture incidence have been observed in recent decades . The reasons for these secular changes are manifold and probably more related to socioeconomic and demographic changes than to direct influences, for example, medication use .
Many studies in Western populations suggest that while the incidence of fractures overall and especially peripheral fractures may have increased, particularly in older women, there has been a modest decrease in age-standardized hip fracture incidence over the last decade, from which men appear to have benefited less . A recent Danish study observed slightly decreasing clinical vertebral fracture rates in women versus increasing rates in men. The decline in major osteoporotic (i.e., hip, spine, wrist, and humerus) fractures was also less pronounced in men, with a decline in the female:male ratio from 2.93 to 2.72 between 1995 and 2010 . Unfortunately, refracture rates or postfracture mortality have remained unchanged .
25.2.3
Childhood fractures
From puberty through midlife the incidence of fractures is greater in men than in women. A peak in fracture incidence coincides with puberty and affects boys later and to a greater extent, with a predominance of upper limb and particularly distal forearm fractures ( Fig. 25.2 ) .
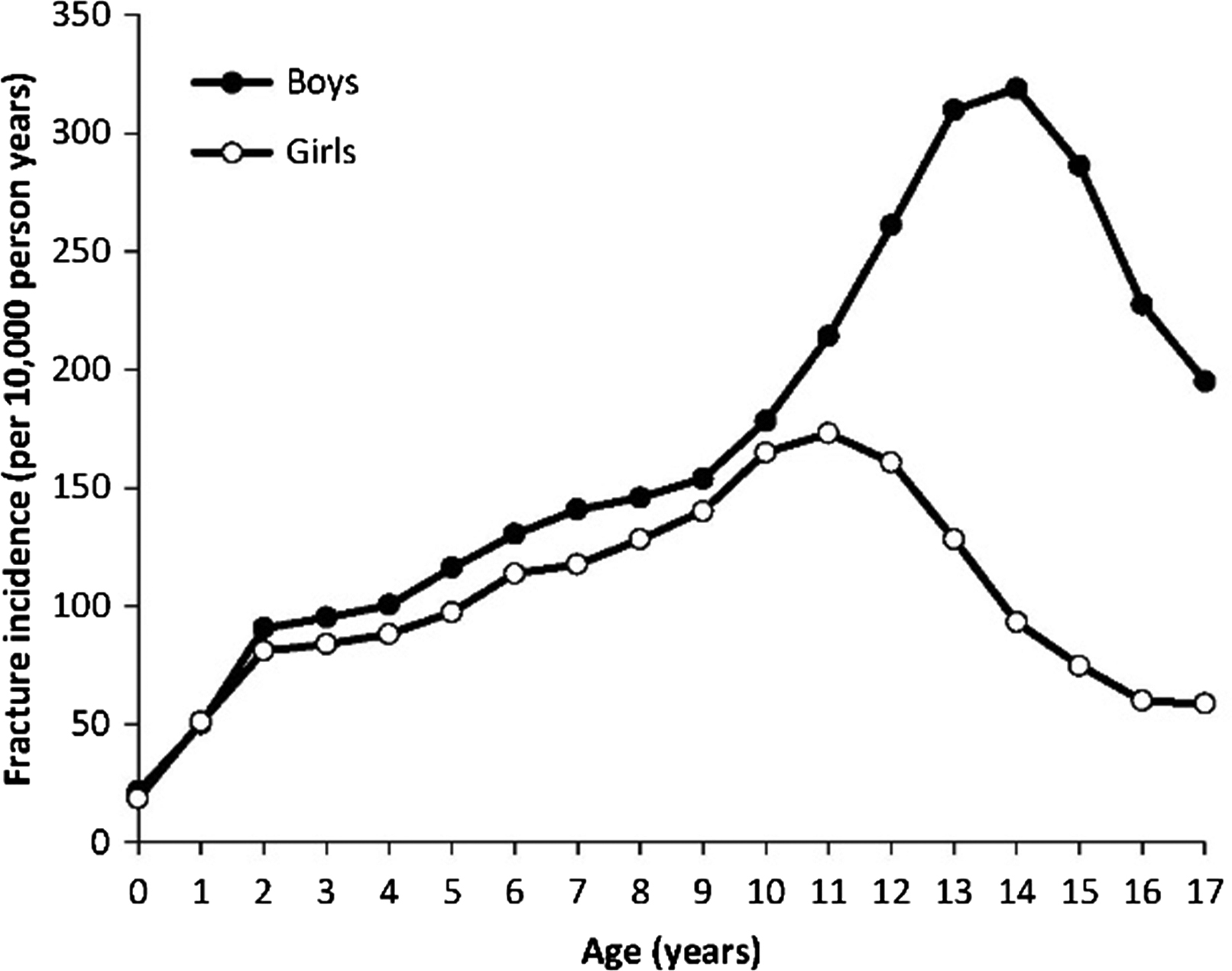
Multiple factors may contribute to this pattern, although the underlying reasons are not fully understood. Behavioral factors, that is, greater physical activity and enhanced risk taking and greater likelihood of injuries from sports or motor vehicle accidents are often cited . However, relative bone fragility may also be involved . During rapid outward cortical bone expansion during the growth spurt, a transient relative cortical bone deficit arises with lagging cortical thickness and density as well as greater porosity. During this phase, a mismatch between load and strength in boys may partly explain the peak in so-called greenstick or distal radius fractures typical for this age .
The prognostic importance of these childhood-to-midlife fractures for later osteoporotic fractures remains incompletely understood. One study suggested that a distal forearm fracture during childhood increased the risk of fragility fractures in older men but not women (possibly due to the overriding influence of menopause) . However, another study found no association in either gender . Perhaps a distinction should be made between high-trauma fractures associated with vigorous physical activity in childhood versus low-trauma fractures associated with underlying structural deficits in bone strength; the latter may track into adulthood .
25.2.4
Hip, vertebral, and peripheral bone fractures in older men
Around the age of 45–50 years, that is, coinciding with the menopausal transition in women, the gender predominance in fracture incidence reverses, with fragility fractures becoming much more common in women ( Fig. 25.3A ). However, there is clear heterogeneity in this sexually dimorphic incidence of fractures. Hip (as well as pelvis), vertebral and humeral fractures display an exponential age-related increase in both genders but more so in women ( Fig. 25.3B–D ). Depending on the type of fracture, the onset of the age-related rise in fracture incidence is delayed in men, from 5 to 10 years for hip and vertebral fractures to as much as 20–25 years later for humeral fractures.
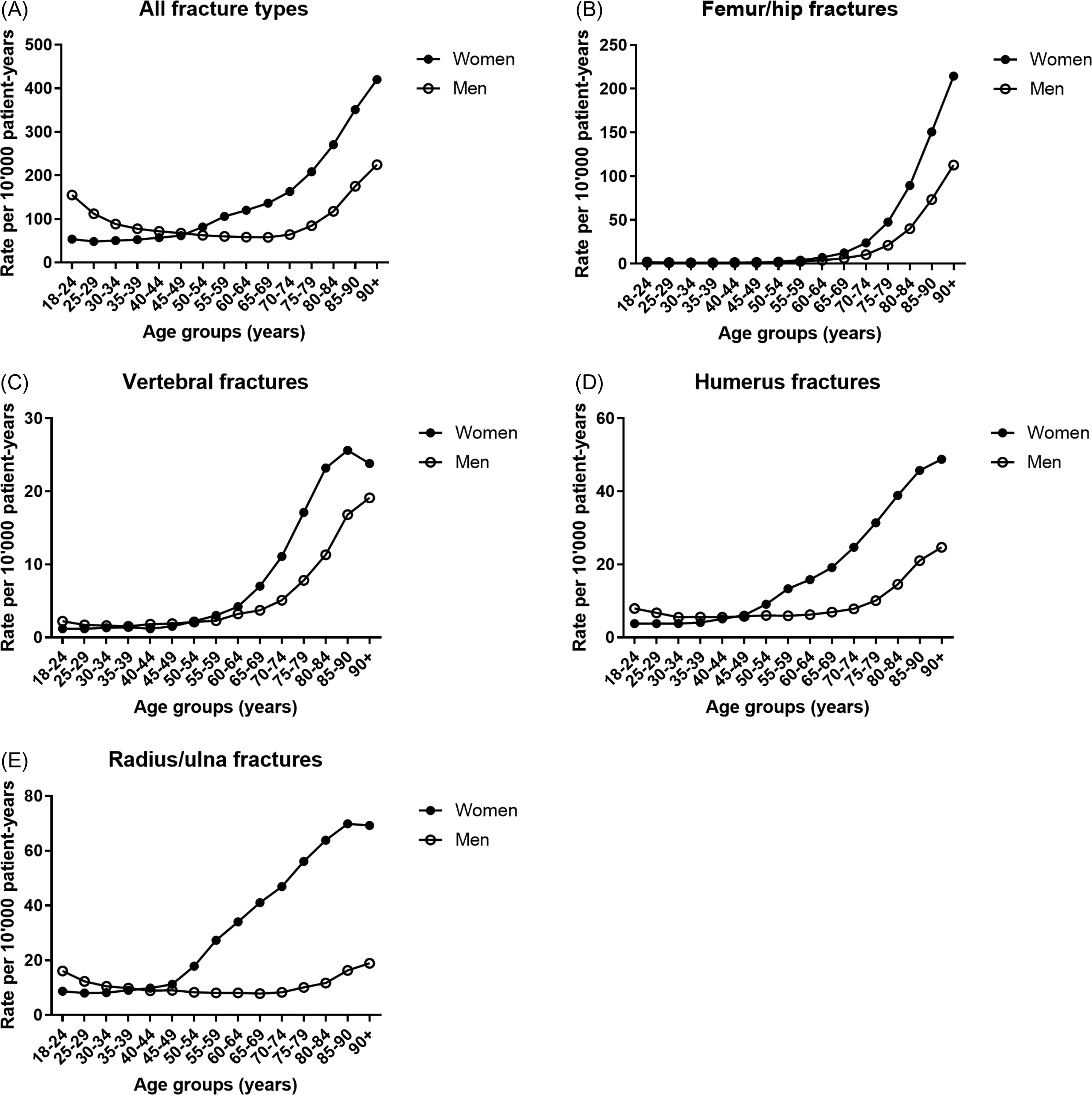
25.2.4.1
Hip fractures
A systematic review showed that the male:female ratio for hip fractures is remarkably constant around 1:2, despite greater than 10-fold variation in incidence between countries worldwide . The lifetime risk of hip fractures after age 50 may be as high as 4%–6% in men, compared to 8.5%–10% in women . A study using nationwide US emergency room data estimated that 19% of women and 12% of men who reach 85 years of age have sustained hip fractures, which rises to 30% of women and 20% of men who reach 90 years of age . Fractures of the hip have the greatest medical and economic impact in both genders, accounting for around half of direct medical costs and hospital days, and with the highest risk of transfer to a long-term care facility, which considerably inflates costs .
25.2.4.2
Vertebral fractures
In contrast to nonvertebral fractures, vertebral compression fractures (as well as rib fractures) in both genders often do not come to clinical attention . In the Osteoporosis Fracture in Men (MrOS) US study for example, less than 15% of radiographic incident vertebral fractures were clinically diagnosed . Still, these unrecognized fractures are associated with back pain and increased risk of subsequent fractures, which justifies screening for occult vertebral fractures. The latter can be accomplished either by vertebral fracture assessment (VFA) during DXA, by plain radiography, or they can be identified during computed tomography (CT) or magnetic resonance imaging of the chest or abdomen for other indications (see Chapter 63 : Vertebral fracture identification).
In noninstitutionalized US adults ≥40 years of age, the prevalence of vertebral fractures was similar in both genders at around 5%, and increased with age in both genders up to around 18%–21% in those aged 80 years and older . Notably, the use of different radiographic criteria to diagnose vertebral fractures (Genant semiquantitative vs modified algorithm-based qualitative technique, mABQ) has recently been revisited . The Genant semiquantitative method is still most commonly used , but the mABQ technique may have some advantages. Interestingly, women in the Canadian Multicentre Osteoporosis Study study had more prevalent and incident vertebral fractures relative to men when using the mABQ technique but not with the Genant method .
25.2.4.3
Wrist fractures
Radius and ulna fractures show no to only a slight age-related increase in men ( Fig. 25.3E ) . This implies that those men who do sustain wrist fractures likely have severe osteoporosis. The latter also explains why the risk of a subsequent hip fracture is greater after a prior distal forearm fracture compared to a prevalent vertebral fracture in men, while the opposite is true in women . In the MrOS study, wrist fractures were associated with low BMD, history of fractures after age 50, use of selective serotonin-reuptake inhibitors, and inability to perform grip strength testing . Interestingly, significant associations of muscle mass and strength or power with radius as well as tibia bone size have been observed in men, suggesting a role for muscle–bone interactions (see Section 25.3.1 ).
25.2.4.4
Rib and other fractures
Rib fractures are slightly more common in men . Overall, fractures other than the classical major osteoporotic fractures and in particular rib fractures are the commonest incident clinical fractures in men, and they have been shown to have all the characteristics of osteoporotic fractures in men .
In summary, efforts for secondary fracture prevention in men should focus not only on hip and vertebral fractures (which have the greatest personal and health economic consequences) but also on nonhip/nonvertebral fractures, because these are either the most important in number (rib fractures) or they are rare but carry a poor prognosis (wrist fractures).
25.3
Why are osteoporosis and fractures less common in men?
25.3.1
Extraskeletal factors
25.3.1.1
Risk of falls
In older men, fractures occur because of a trauma (most commonly minor, e.g., a fall), which exerts a force that exceeds the biomechanical competence of bone. Thus both skeletal fragility (discussed in Section 25.3.2 ), risk of falls and defense mechanisms (e.g., soft tissue padding around the hip) determine fracture risk (see Chapter 26 : Falls as risk factors for fracture). Indeed, most fractures occur in people who do not have a baseline T-score of ≤−2.5 (as much as 73% of female and 94% of male fracture cases, according to a recent analysis from the Dubbo Osteoporosis Epidemiology Study ).
Older men have a lower risk of falls than women , in part due to differences in the prevalence of certain falls risk factors (e.g., urinary incontinence, which is more common in women) . Still, risk factors for falls such as obesity or functional impairment clearly increase the risk of fractures in men . Notably, low testosterone (T) has been associated with risk of incident falls in older men . However, T treatment did not improve physical functioning in hypogonadal men in the Physical Function Trial of the Testosterone Trials, may possibly be associated with adverse cardiovascular events, and has not been shown to reduce the risk of falls .
25.3.1.2
Trochanteric soft tissue
Trochanter soft tissue thickness is associated with fracture protection in women but studies in men are somewhat contradictory . Possible reasons why trochanteric soft tissue thickness may be protective in women but not men include (1) lower amount of soft tissue around the hips in men, (2) less fat versus muscle tissue (with differences in stiffness and attenuation force), and (3) a correlation between soft tissue thickness and BMD that is positive in women but negative in men .
25.3.1.3
Muscle–bone interactions
As mentioned earlier ( Section 25.2.4.3 ), there are clear correlations between muscle and bone in men (as in women), both during peak bone mass (PBM) acquisition and subsequent bone loss . Male bone mineral content (BMC) is associated not only to appendicular muscle mass but also to type II (fast-twitch) muscle fiber atrophy . However, the question of whether the risk of falls and/or fractures is lower in men compared to women because men have greater muscle mass and strength remains unanswered and difficult to address. Notably, current definitions of sarcopenia adopt gender-specific cut points for muscle mass and strength, resulting in virtually identical prevalences of sarcopenia in older men and women (even though the average 85-year-old man may have the same average grip strength as a 30-year-old woman) .
Sarcopenia (defined as low muscle mass) has been found to predict fractures in the presence of low BMD, in two out of five studies in one metaanalysis, but only in men . However, several other studies found no association of appendicular muscle mass with fracture risk that was independent of BMD in men . Nowadays, there is increasing emphasis on the role of muscle strength and functional performance rather than muscle mass per se (see Chapter 35 : Bone, muscle, and sarcopenia). Nevertheless, muscle and bone are strongly interconnected tissues , and development of greater muscle mass may contribute to greater PBM in younger men (see next).
25.3.2
Hierarchical determinants of male bone strength: size matters
25.3.2.1
Peak bone mass and bone mineral density across the life span
Bones have a complex three-dimensional (3D) organization at the macro-, micro-, and nanoscale, all of which influence bone strength. Despite these complexities, bone strength correlates well with BMD, which is a simple radiological two-dimensional projection measuring BMC over the bone area in the region of interest. The gender differences in facture risk correlate with differences in bone strength. This skeletal sexual dimorphism has origins in different phases of life: PBM acquisition, consolidation during midlife, and bone loss in old age ( Fig. 25.4 ).
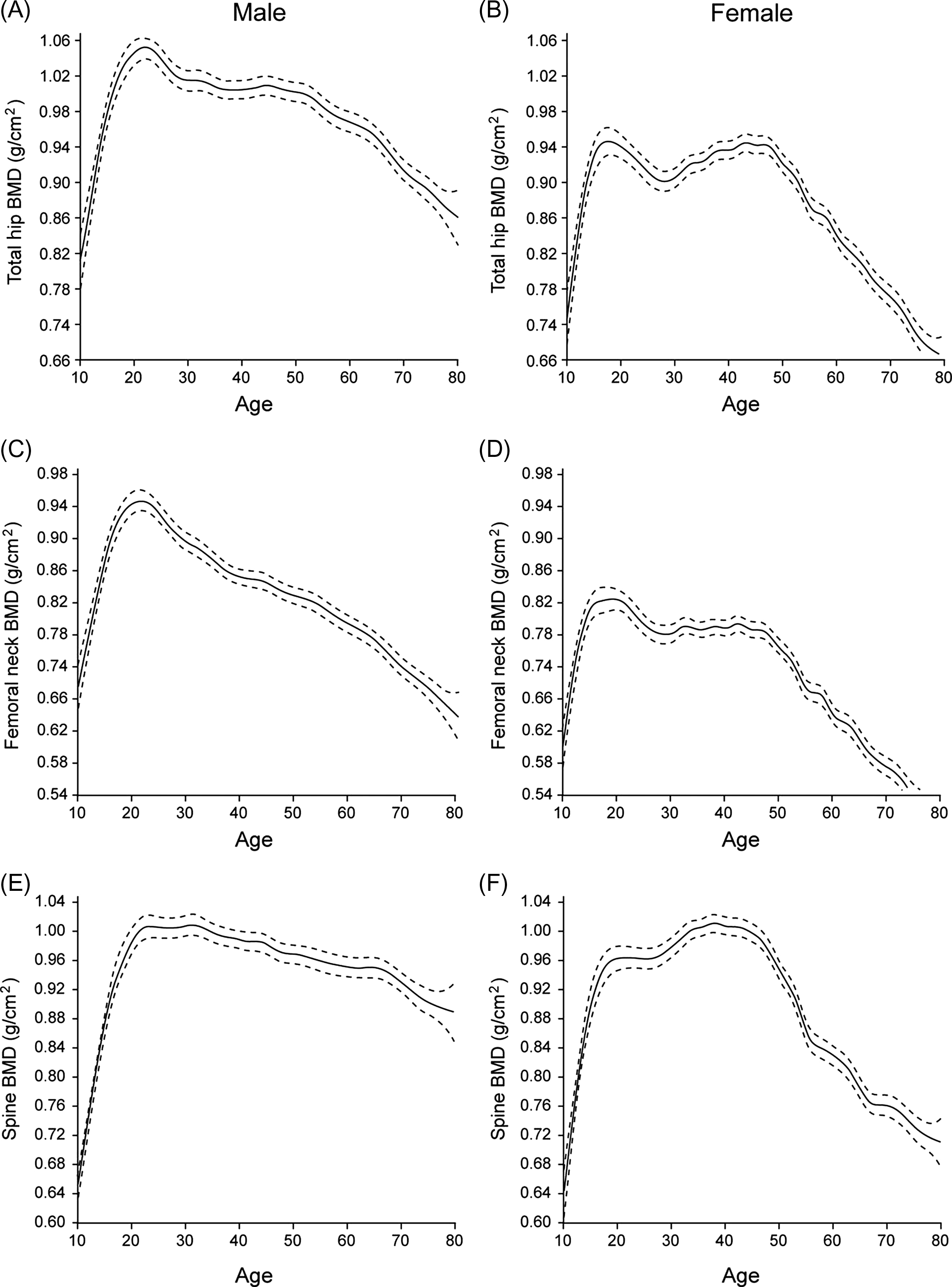
Evidently, the rise in fracture incidence in women around the age of menopause can be attributed to bone loss related to estrogen deficiency. However, PBM and midlife consolidation are still underrecognized but crucial determinants of fracture risk later in life, as well as gender differences therein. Simulations suggest that increases in PBM as small as 10%, if followed by parallel bone losses, could delay the onset of osteoporosis by more than a decade .
As shown for example in Fig. 25.4E–F , peak lumbar spine BMD is quite similar in men and women, but women experience a sharp decline after menopause, whereas the decline in men is more gradual . However, vertebral BMD measurements in men are prone to confounding by osteophytes (more than in women) , and quantitative CT (QCT) is better suited to reveal spinal bone loss in men . Still, lumbar spine volumetric BMD (vBMD) loss by QCT is greater in postmenopausal women than in older men .
At the total hip and femoral neck, the rate of BMD decline after age 50 is also lower in men, but their BMD around that age is also slightly (~5%) higher despite an early decline in hip BMD after PBM in men ( Fig. 25.4A–D ) . Several studies show low PBM in sons of men with osteoporosis, particularly at the lumbar spine, lending credence to the notion that idiopathic male osteoporosis may result from “tracking” of PBM deficiency . In women, there is even more evidence that osteoporosis may be due not only to differences in bone loss but also to differences in how much PBM is attained before the onset of bone loss, depending on the site examined .
Second, there are important changes between the third and fifth decade of life. For example, one study indicated that 25% of PBM at the femur was lost by age 50 in men (although birth cohort effects and other confounders preclude firm effect estimates based on cross-sectional data) . However, there is no apparent loss in bone strength or increase in fracture risk during this period. Instead, early BMC/BMD loss after PBM likely reflects cortical consolidation, that is, shift from trabecular to cortical bone, with ongoing periosteal bone expansion, further increases in cortical bone thickness, which both contribute to increasing bone strength (see Section 25.3.2.2 ).
Young men aged 20–30 years have on average about 25% greater PBM, that is, whole-body BMC than women . However, this is entirely expected given that men have bigger bones because they are on average 8% taller (because in 3D, 1.08³≈1.25) . Previous DXA-based studies already showed that there are site-specific differences in the timing of PBM acquisition, but gender differences in BMC are largely determined by greater bone area in men. For example, Henry et al. showed that PBM was reached age 21–22 in men versus 23–28 years in women . Peak BMC was greater in men at the femoral neck (+28%), ultradistal radius (+44%), and lumbar spine (+12%) . Differences in areal BMD (aBMD) are however much smaller (5%–15%), and they become negligible when vBMD is calculated or when BMD is adjusted for greater bone size in men . Notably, vBMD at the spine appears to be lower in men, but this negative effect is entirely offset by greater vertebral body size .
Trabecular bone score (TBS), a novel texture-based measure derived from spine DXA images, is lower in women (at least when TBS-v2 is used ), although the meaning of this difference is unclear.
25.3.2.2
Gender differences in cortical bone
The main structural determinant of the greater bone strength in men is the fact that at any age, they have much wider bones, that is, greater cortical bone diameter compared to women, even following adjustment for gender differences in height ( Fig. 25.5 ). This has important biomechanical advantages because cortical bone carries the greatest loads, and cortical bone strength scales with bone diameter to the fourth power, independent of cortical thickness (see Chapter 13 : The mechanical behavior of bone). Thus it is not surprising that low bone width predicts fractures in men independent of BMD . Techniques such as (peripheral or central) QCT and, more recently, high-resolution peripheral QCT (HR-pQCT) have allowed to better understand the structural determinants of bone strength across the life span, and gender differences therein . Additional finite element analysis allows noninvasive simulation of bone strength, strength-to-load ratio (e.g., in a sideways fall on the hip configuration), although this requires assumptions regarding gender differences in material properties or nanostructure (see Section 25.3.2.4 ).
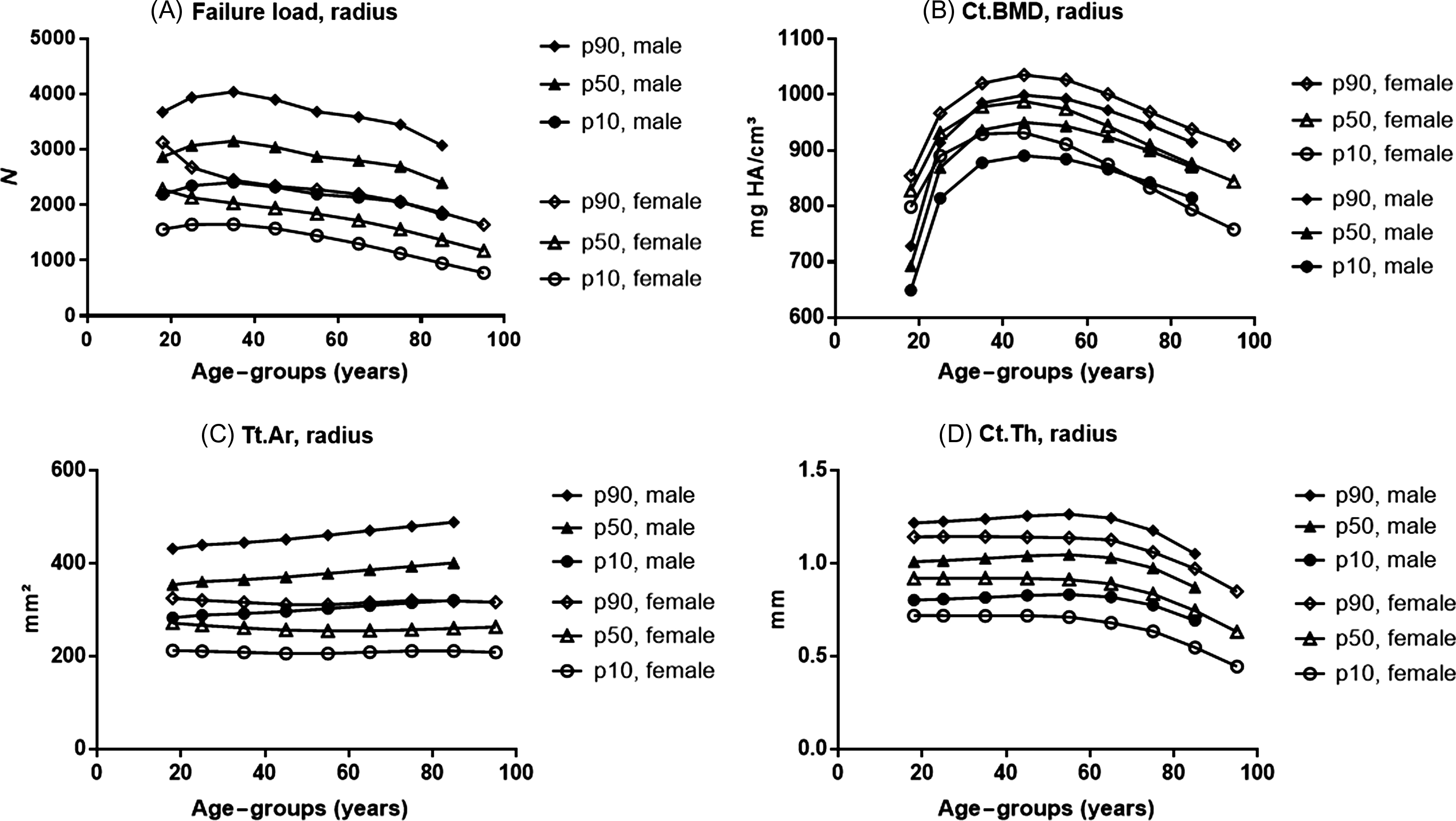
Sexual dimorphism in cortical bone arises during puberty, when periosteal bone formation rate and outward radial bone expansion are greater in boys . This leads to wider cortices and greater cross-sectional total area ( Fig. 25.5A ) in young men. Because the medullary cavity widens in parallel, cortical thickness is only slightly greater in young men around the age of PBM at the radius (see Fig. 25.5D ), and not significantly different at the tibia . Previously, it was believed that girls had greater endosteal apposition than boys, that is, contraction of the marrow area, but recent longitudinal studies show that both genders have endosteal expansion accompanying periosteal expansion, but less so in girls . Thus the most important effect of estrogens on the endosteal surface may be that they limit endosteal resorption, rather than their stimulation of endosteal bone formation.
All other cortical and trabecular parameters also show significant gender differences at PBM, except for total BMD. Cortical vBMD is similar or maybe even slightly lower in men around the age of PBM . Cortical porosity is also higher in boys than in girls during pubertal growth, which may contribute to the observed higher incidence of distal radius fractures in growing boys (see Section 25.2.3 ).
We can conclude that 20- to 30-year-old men have wider, but not necessarily thicker and certainly not denser or more mineralized bones. Nevertheless, their greater bone diameter provides men at PBM with 48% and 38% greater estimated failure load at the radius and tibia, respectively .
A second important explanation for the greater bone strength in men lies in midlife: during this phase, they continue to accrue cortical bone mass, whereas women do not. Indeed, cortical thickness and especially cross-sectional area continue to increase throughout midlife in men, but not in women . Although sex steroids are believed to be involved during puberty and after menopause or in aging men, the drivers of changes during midlife in either sex are not fully understood.
Finally, structural bone decay is much greater in postmenopausal women compared to their age-matched male counterparts, on all bone surfaces. Cortical bone loss begins around the age of menopause in women, while in men, only mild decreases occur and mainly after age 75 . The greater loss of cortical thickness and cortical bone area in women has two reasons: endosteal resorption is greater in postmenopausal women, and in contrast to men, ongoing periosteal expansion is unable to compensate sufficiently.
All data confirm that medullary area expansion (i.e., endocortical bone resorption) is less pronounced in men versus postmenopausal women. However, there are some discrepancies between studies as to whether periosteal expansion in aging men is greater, similar, or even lower than in women. Possible reasons for different conclusions between studies are the inclusion of different age-groups, the use of different time horizons for analysis, site-specific differences, for example, between the radius and the tibia , and the fact that most studies are cross-sectional or short-term rather than long-term longitudinal follow-up studies . For example, one longitudinal HR-pQCT study showed no significant difference in periosteal expansion rate according to menopausal status or gender at either the radius or tibia, but follow-up duration was only 3 years . Cross-sectional data from the InCHIANTI study suggested that at the tibia, men have greater periosteal expansion than women after PBM . In contrast, later longitudinal data from the same study showed that men have greater periosteal expansion from 20–30 to 50–60 years of age (while cortical bone area increases further), whereas women have greater periosteal expansion from 70 to 80 years of age onward (while their cortical bone area is nevertheless decreasing) .
Fewer studies have evaluated sexual dimorphism in hip and spine cortical and trabecular bone loss with aging. In a cross-sectional study, the estimated strength of vertebral body declined twofold faster with age in women. Both genders lost trabecular bone, but only men maintained their outer cortical shell, which became progressively more important as trabecular bone loss continued . A cross-sectional QCT study by Riggs et al. showed that cortical cross-sectional area increased between 20 and 90 years at the lumbar spine, femoral neck, distal radius, and distal tibia in both genders, but only at the distal radius was the rate of increase greater in men versus women .
Other studies confirm that cortical cross-sectional area increases in both genders but more so in men at the radius, but not at the tibia . One longitudinal study by Szulc et al. based on DXA imaging at the radius even showed that menopausal estrogen deficiency is associated with declining periosteal expansion . In contrast, Ahlborg et al. showed that postmenopausal estrogen deficiency was associated with accelerated bone turnover, endosteal bone loss and a compensatory increase in periosteal expansion at the radius, which partly maintained bone strength . In any case, data in men consistently show that the rate of periosteal expansion at the radius or tibia remains more or less constant between 20 and 80 years of age, while medullary area expands mostly after age 75 .
Longitudinal radius pQCT data from the European Male Ageing Study showed, in analogy with the Alhborg et al. findings, that the net outward displacement of the cortex was related to bone turnover markers (although not to sex steroid deficiency, which was uncommon in men). These findings support the hypothesis that periosteal expansion is driven by endosteal resorption to maintain bone strength . In line with these findings, changes in cortical bone were associated with changes in muscle area in the Hertfordshire cohort study . Furthermore, cortical bone area is greater in men from rural areas, more active men and those with greater lean body mass . Also in several studies, lower cortical vBMD was associated with (and compensated by) greater cross-sectional area and strength . However, Szulc et al. observed no association between prospective periosteal expansion at any site and baseline bone turnover markers (BTMs) in a smaller male population with a broader age range .
In the MrOS study, cross-sectional hip QCT data showed that in aging men, medullary expansion was compensated by periosteal expansion leading to maintained cortical thickness at the femur shaft. At the femoral neck however, cross-sectional area increased minimally with age, while medullary volume increased and cortical bone volume decreased with age . These findings emphasize the site-specific nature of cortical bone loss, even within the same bone. Longitudinal QCT data from the Age, Gene/Environment Susceptibility (AGES)–Reykjavik study showed that men, but not women, in the highest quartile of BTMs had higher femoral neck periosteal expansion . Thus bone resorption may drive periosteal expansion in the elderly, but the balance between endosteal resorption and periosteal expansion may be more beneficial in men.
As explained previously, the corollary of stronger, mainly cortical bone on average in men is that those men who do sustain, for example, hip fractures, have more marked structural bone decay compared to nonfracture male controls, than female fracture versus nonfracture cases .
Cortical porosity increases markedly with aging and more so in women (fourfold vs twofold increase in men), mainly due to increasing pore number (while average pore size shows only small to nonsignificant increases in both sexes) . It should be noted however that cortical porosity and thinning do not occur uniformly throughout the cortex, and that age- and gender-related differences are more evident in regions experiencing greater loads . Notably, a recent biomechanical investigation of male postmortem radii found that greater bone size was associated with worse cortical porosity . Whether cortical porosity is a cause or a consequence of periosteal expansion remains to be investigated in prospective studies.
In summary, the greater cortical bone strength in men is mainly related to greater periosteal expansion during puberty and midlife as well as protection against cortical thinning and porosity with aging. Since most fractures occur at peripheral, mainly cortical bone sites, these gender differences in cortical PBM acquisition and maintenance likely account for most of the sexual dimorphism in nonvertebral fracture risk.
25.3.2.3
Trabecular bone
Trabecular vBMD and bone volume is greater at PBM in men at the distal radius, and in some (but not all ) studies also at the tibia . Khosla et al. reported that young men had greater trabecular thickness but similar trabecular number and separation . Hansen et al. however found that both trabecular number and thickness were greater in men, at both the radius and tibia , whereas Macdonald et al. found greater trabecular number and thickness in men at the radius, but only greater trabecular number and not thickness at the tibia .
Trabecular PBM is significantly lower in men at the proximal femur and lumbar spine . As for cortical bone, these differences are not evident before puberty . Despite the lower trabecular vBMD or trabecular bone volume in the spine, the total trabecular BMC is greater at PBM in men because of their greater vertebral body size (i.e., the outer—cortical—bone shell) .
Trabecular bone loss is evident in both sexes early after PBM . Again, the underlying reason(s) for this early trabecular bone loss are incompletely understood. One possible explanation is that as a result of cortical consolidation, that is, increasing cortical bone strength, trabecular bone is resorbed because it is no longer loaded and thus—like a scaffold below the growth plate—the requirement for trabecular bone diminishes once the construction of cortical bone is complete .
The rate of decline of trabecular vBMD is greater in women at the lumbar spine and femoral neck , but the decline in trabecular bone volume is not different between genders at the distal radius and tibia . Nevertheless, men show greater declines in trabecular thickness , whereas women show significantly greater loss of trabecular number and greater increase in trabecular separation at the distal radius (but not at the distal tibia) . The latter reflects trabecular perforations, which have greater biomechanical disadvantages than trabecular thinning in men, providing a further structural explanation for the greater bone strength and lower fracture risk in men.
25.3.2.4
Bone material properties and nanostructure
Most studies suggest that material properties, for example, mineral/matrix ratio are not influenced by gender per se, although sexual dimorphism may result indirectly from differences in bone size, geometry, turnover, and tissue age . Studies reporting gender differences in bone material properties independent of bone structure, for example, viscoelasticity are few, small, and contradictory . Interestingly, some studies suggest that men with hip fractures have superior trabecular bone strength as well as greater reference point indentation values than women . However, bone material strength measured by nanoindentation in vivo does not differ between genders .
Despite clear age-related changes in osteocyte lacunar and canalicular porosity (the nanoporosity resulting from blood vascular channels), gender differences in bone’s nanoporosity remain unclear . One study reported that men had lower canal porosity and diameter at the femoral neck . A recent study in mice found no gender differences in wild-type mice, whereas conditional deletion of vascular endothelial growth factor in osteoblasts markedly increased vascular porosity, but only in males . Thus, although further investigation is needed because greater biomechanical properties in male bone may not to be entirely explained by superior cortical bone geometry , the latter is currently still the single most important determinant of greater male bone strength.
25.3.3
Sex steroid regulation of cortical and trabecular bone
25.3.3.1
Sex steroids or other factors
After having outlined the gender differences in bone strength and extraskeletal fracture risk factors across the life span in the previous section, the next question becomes “what causes these gender differences?” The most evident answer is androgens and estrogens, the sex steroid hormones, which bind with high affinity to their cognate nuclear receptors, the androgen receptor (AR) or the estrogen receptors alpha and beta (ERα and ERβ), respectively. However, it is important to emphasize that there are other potential, often overlooked and still poorly understood mediators of skeletal sexual dimorphism.
Lessons from bone health in transgenders
Several interesting conclusions can be drawn from studies on bone health in transgender persons. In one large cohort, low BMD (Z-score <−2.0) was found in 21.9% of trans women (male-to-female transgenders) and 4.3% of trans men before the start of any hormonal therapy , pointing to important behavioral aspects of gender. Trans women also have lower BMD at all sites, smaller cortical bone size as well as lower muscle mass and strength before the start of any cross-sex hormone therapy, in line with their lower involvement in athletic physical activities .
Cross-sex estrogen therapy is associated with lower BTMs and increased BMD at the hip and spine in trans women, despite declining muscle and increasing fat mass . BMD increases at the total hip as well as at the lumbar spine in trans men of postmenopausal age . Thus long-term transgender hormone therapy can be considered safe from a skeletal perspective . In a recent metaanalysis, BMD did not change after 1–2 years of T in trans men, whereas a small increase at the lumbar spine was evident in trans women , confirming the primary importance of estrogens over androgens in either gender. Still, T treatment in younger trans men has been associated with increasing BTMs, gains in muscle mass and strength, less adiposity, and a small increase in trabecular vBMD at the distal radius and aBMD at the hip, in line with an anabolic effect of androgens on muscle and bone . BTMs also decrease in trans men ≥50 years of age (i.e., previously postmenopausal women), attesting to the antiresorptive effects of estrogens in both genders . After long-term T treatment, trans men have larger cortical bone expansion at the radius and tibia (which might in turn explain their lower trabecular vBMD) . In pubertal adolescents however, gonadotropin suppression and cross-sex hormone therapy has been associated with decreased bone formation markers and a transient decline in lumbar spine Z-scores . The use of different progestins may however influence body composition changes. Trans boys receiving proandrogenic lynestrenol gained lean body mass and grip strength. In contrast, the use of cyproterone acetate in trans girls decreases lean mass, grip strength; increases fat mass; and impedes their cortical bone expansion and trabecular PBM acquisition at the lumbar spine . The latter findings are in line with the effects of androgens on cortical and trabecular bone in growing mice (see later). Collectively, these findings demonstrate the powerful influence of sex steroids over chromosomal factors.
Insights from disorders of sexual development
Few studies are available on bone health in patients with disorders of sexual development. One study found that patients with gonadal dysgenesis (with either 46,XX or 46,XY chromosomal background) had inferior hip BMD compared to women with complete androgen insensitivity syndrome (due to mutations in the AR inducing resistance to androgens), suggesting that gonadal factors (most likely, estrogens) play a dominant role regardless of sex chromosomes . Women (46,XY) with complete androgen insensitivity syndrome have slightly reduced hip and spine BMD, which suggests a role of androgens on PBM acquisition .
Chromosomal determinants
Whether genetic factors play a role in skeletal sexual dimorphism remains controversial: one must then ask whether an autosomal gene-by-sex interaction is not a consequence of interactions with sex steroid signaling. The most recent genome-wide association study (GWAS) meta-analysis on this topic found no gene-by-sex interaction effect on adult BMD . However, a smaller GWAS suggested the existence of two gender-specific loci for pediatric BMD (near SPTB in females and IZUMO3 in males) , and earlier studies in mice have suggested that both genders, common as well as gender-specific genetic influences, regulate PBM . Several studies have identified regulators of bone metabolism only in one gender, which was apparently independent of sex steroids, for example, as recently shown for Krox20 in female mice . Larger human genetic studies on this topic are eagerly awaited.
Other factors in the reproductive axis
However, it has become clear that the gonads produce more than just sex steroids that directly regulate bone health, such as insulin-like peptide 3 and inhibin A (which is closely related to activins) . These links between bone and reproductive health require further investigation.
A possible direct role of follicle-stimulating hormone (FSH) on bone cells has attracted considerable attention and controversy . At least in men however, epidemiological and short-term interventional studies suggest that FSH does not influence bone turnover independently of sex steroids .
Many studies also suggest an association of sex hormone–binding globulin (SHBG) with male bone health, sometimes even independent of free sex steroid concentrations . Findings from the MrOS study showed that hip BMD loss was associated with low bioavailable E2 (bioE2, i.e., non-SHBG-bound E2), but men with the combination of low bioE2 and high SHBG had the fastest bone loss and the highest fracture risk . Longitudinal data from the Concord Health and Ageing in Men Project (CHAMP) showed that SHBG was the only sex steroid–related parameter independently associated with hip BMD loss and fracture risk . However, transgenic overexpression of human SHBG in mice (which naturally lack circulating SHBG) does not lead to a bone phenotype, and any speculative mechanism via purported SHBG receptors remains doubtful . All in all, SHBG concentrations may be more of a surrogate marker than a direct mediator in male osteoporosis. Although these studies support the free hormone hypothesis and determination of free sex steroid concentrations in clinical practice , T, E2, or SHBG measurements have limited clinical utility in the clinical evaluation of osteoporosis in older men .
Relation to age-related bone loss
By the end of the 1990s the important role of estrogens not only in postmenopausal but also in male osteoporosis as well as in osteoporosis in the elderly (then called “involutional” or “type II” osteoporosis) and even a possible roles in osteoporosis associated with secondary hyperparathyroidism or glucocorticoids was established . However, over the last decade, consensus has been reached that a revision of this model is needed, placing more emphasis on the role of generic aging mechanisms (e.g., oxidative stress and cellular senescence) in bone loss in the elderly of either sex . While sex steroids in part regulate bone cell apoptosis by regulating oxidative stress through nongenomic mechanisms (see later) , recent studies in animal models confirm that estrogen deficiency and cellular senescence act through independent mechanisms .
25.3.3.2
Role of androgens versus estrogens on peak bone mass
Androgens are converted into estrogens [e.g., T into estradiol (E2)] by the aromatase (CYP19A1) enzyme. Notably, circulating E2 is primarily of ovarian origin in premenopausal women, whereas peripheral aromatization is estimated to account for more than 85% of serum E2 in men . On average, total T slightly declines and E2 remains unchanged or slightly increases in elderly men, but because SHBG increases, free T and E2 decline . Older men have higher circulating E2 levels than postmenopausal women, but significant amounts of androgens and estrogens are synthetized locally within tissues via intracrine mechanisms, including within bone .
A large body of human observational studies, genetic evaluations, and randomized trials support a role for estrogens on male osteoporosis, while the evidence for an independent effect of androgens is much less clear . Given the strong correlation between T and E2 in men, the partly intracrine mechanism of action of sex steroids and the ethical challenges of performing interventional studies during PBM acquisition, studies in genetic animal models have been valuable to define whether the effects of sex steroids are mediated primarily via ERs or the AR. These studies clearly support an independent role for the AR in male periosteal bone expansion during puberty as well as for trabecular bone. Nevertheless, there are many limitations to the use of such animal models .
Insights from rodent models
Studies using T or dihydrotestosterone (DHT, which cannot be converted to E2) in AR knockout (ARKO) or AR-resistant (testicular feminization or Tfm) mice have shown that androgens via the AR stimulate cortical and trabecular PBM acquisition in males by increasing periosteal bone expansion as well as inhibiting trabecular (but not endosteal) bone resorption . Aromatase inhibition does not influence the effects of T on murine trabecular bone, muscle or fat mass . Selective AR modulators (SARMs) also prevent trabecular bone resorption and maintain periosteal bone formation in male rodent models . These findings support a direct role for the AR in these processes. Male mice with genetically inactivated steroid reductase 5α type 1 (which together with 5α-reductase type 3 converts T into DHT and is expressed at low levels in bone ) have reduced trabecular vBMD, reduced periosteal bone expansion, and lower muscle strength , again suggesting a role for the AR in these phenotypes. However, these effects may be developmental in origin, since pharmacological type 1 (nor type 2) 5α-reductase inhibition (finasteride or dutasteride) do not appear to influence the effects of T on muscle or bone in adult rodents or older men . Finally, a mouse model with a mutant AR resistant to SUMOylation (a posttranslational modification involving reversible attachment of Small Ubiquitin-Related MOdifier proteins) displayed a bone phenotype, while other androgen-sensitive organs were normal . These mice had compromised trabecular bone volume and periosteal expansion. Unexpectedly, this was accompanied by a reduced number of osteoblasts and lower trabecular bone formation (whereas the dominant effect of androgens on trabecular bone is antiresorptive).
Estrogens via the ERα in male mice are also required for optimal periosteal bone expansion and suppression of endocortical bone resorption . They increase trabecular bone volume by increasing bone formation and reducing bone resorption in an ERα-dependent manner ( Fig. 25.6 ). For trabecular bone, either androgen or estrogen signaling appears sufficient to compensate for loss of the other sex steroid, whereas for optimal cortical bone development, their combination appears indispensable . Indeed, aromatase inhibition blunts the effects of T on cortical but not on trabecular bone in male rodents . Similarly, male mice overexpressing aromatase either globally or specifically in the osteoblast lineage have increased bone mass . Conversely, male aromatase knockout (KO) mice have clear osteopenia in the trabecular and endocortical compartment, with increased bone resorption and decreased bone formation . Orchidectomy triggers further trabecular bone loss and reduced periosteal bone formation in young male aromatase-KO mice, in line with the role of androgens in these processes .
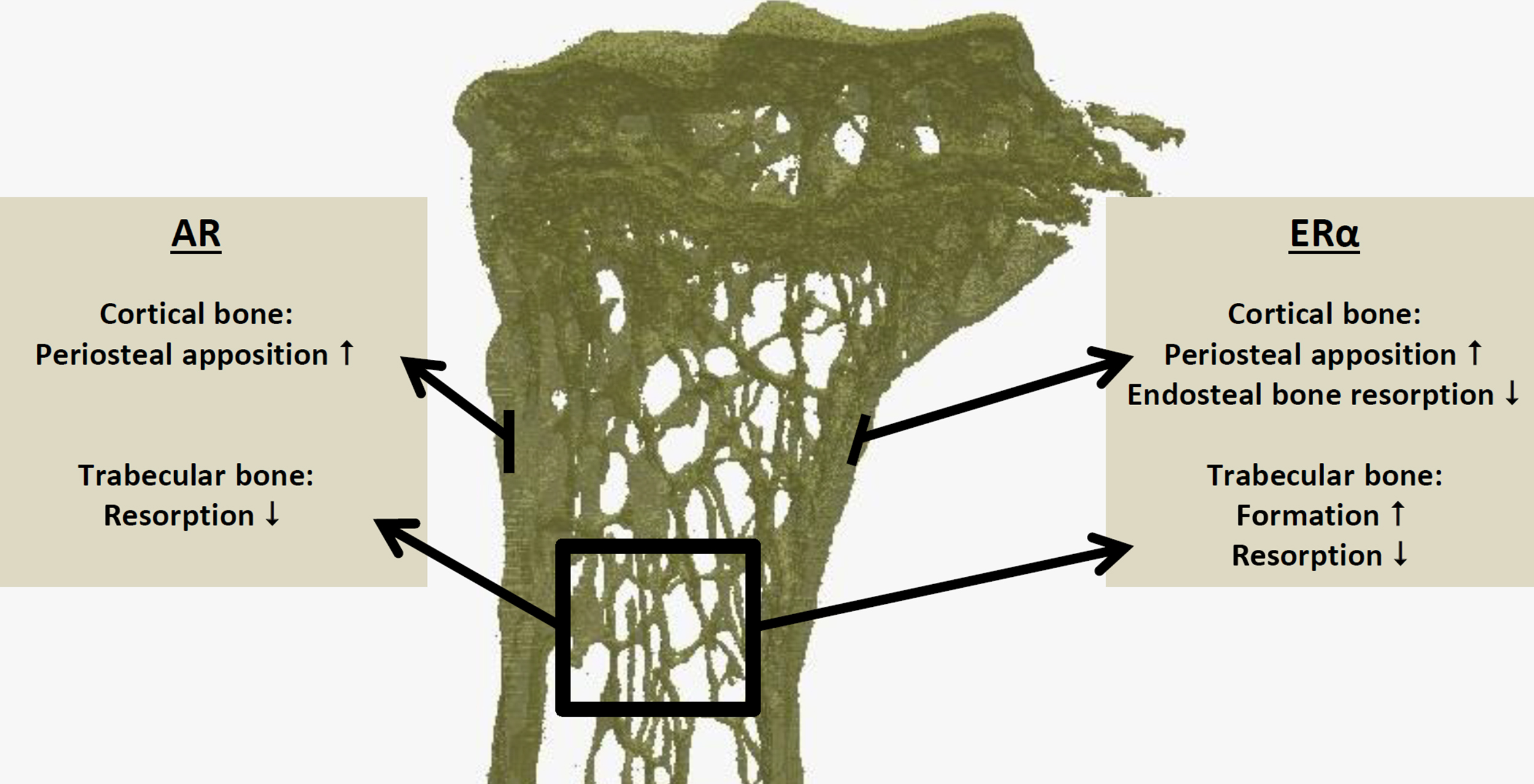
It should be noted that the effects in KO mice are developmental, that is, even a phenotype at an older age could be a consequence from in utero effects on, for example, the known imprinting of the gonadotropic axis ( Section 25.3.3.6 ). However, an elegant study comparing primary hypogonadal versus orchidectomized mice concluded that the perinatal T surge determined longitudinal growth and height difference in males but not their cortical or trabecular bone mass . Recently, Ohlsson’s group using inducible global ARKO mice showed that both pre- and postpubertal AR deletion trigger cortical and trabecular bone loss in adult male mice, while only prepubertal AR inactivation increased fat mass .
Human genetic evidence, epidemiological studies, and randomized trials
In line with the important role for estrogens in male osteoporosis are case reports of low bone mass in men with mutations abrogating aromatase or ERα activity . The ERαKO man showed low trabecular vBMD and endocortical bone loss, with thinner trabeculae and lower trabecular bone formation on bone biopsy . His periosteal circumference was however normal, which may be explained as a compensatory mechanism or by increased insulin-like growth factor-1 (IGF-1) levels. Longitudinal follow-up of a boy with congenital aromatase deficiency also showed that estrogen therapy increased periosteal bone expansion while lowering T to normal values .
Genetic polymorphisms influencing CYP19A1 activity and thus synthesis of estrogens as well as estrogen catabolic enzymes have been associated with BMD and fracture risk in men . In contrast, polyglutamine (CAG) repeats associated with reduced AR signaling are associated with higher (not lower) BMD because of hypothalamic–pituitary–gonadal feedback regulation and enhanced estrogen signaling . GWAS studies and metaanalyses clearly associate polymorphisms near ESR1 with bone density, calcaneal ultrasound parameters, cortical (and in one study, trabecular) vBMD as well as fractures in both genders . A recent Mendelian randomization study provided further evidence of a causal effect of circulating E2 on male BMD . Notably, the genome-wide significant association of variants near ESR1 with total body BMD represent one of the few age-specific factors, that is, with increasing influence at older ages .
Since the late 1990s a multitude of epidemiological studies found associations of BMD and fracture risk with circulating estrogen concentrations in men, especially bioavailable (or sometimes free) E2 . In contrast, many studies found no association with (total, free or bioavailable) T concentrations, with notable exceptions . In the MrOS Sweden and US cohorts, men with low bioE2 had increased fracture risk , particularly when this was combined with high SHBG and low bioT . More recently, low bioE2 has also been associated with vBMD and structural bone decay in older men . In a cross-sectional study, Khosla et al. reported that low bioE2 (<30 pM) was associated with cortical vBMD in elderly but not in young (eugonadal) men . Vandenput et al. reported that E2 was associated with cortical porosity in older men . However, since longitudinal bone loss is limited in older men, not all prospective studies may have been sufficiently powered to show significant associations . In the large MrOS study, bioE2 and bioT were positively and SHBG negatively associated with trabecular vBMD measured by QCT at the spine , whereas associations of these sex steroids with cortical and/or hip vBMD were not significant. Most recently, longitudinal HR-pQCT from the STRAMBO cohort showed that cortical and trabecular bone loss after 4 and 8 years were associated with low bioE2 and (to a lesser extent) free T concentrations, particularly when both sex steroids were compromised . Unfortunately, periosteal expansion was not examined in this study.
Significant bone loss occurs in men treated with androgen deprivation therapy for prostate cancer, but this is of course also estrogen depriving . In fact, the selective ER modulators (SERMs), raloxifene and toremifene increase BMD , while toremifene also decreased bone turnover markers and reduced the risk of fractures in these men . In older men however, positive effects on bone turnover have only been noted in men with low circulating E2 levels . In contrast, SARMs have not been shown to prevent bone loss in men , and pure antiandrogens for prostate cancer have not been associated with bone loss. However, this issue deserves further careful investigation, since enzalutamide treatment in mice reduces trabecular bone in the spine of male mice .
The most convincing evidence comes from randomized clinical investigations using aromatase inhibitors with or without gonadotropin suppression. Khosla’s group reported that E2 appeared to be the major regulator of bone turnover in men , whereas the effect of androgens was not significant in stringent two-way ANOVA . However, both T and E2 were associated with the bone formation marker osteocalcin . Leder et al. however found that both androgens and estrogens appeared to regulate bone resorption markers . Nevertheless, aromatase inhibition, while increasing T, reduced BMD . Treatment with DHT, which suppresses E2, also reduced BMD in a clinical trial . Recently, Finkelstein et al. reconfirmed that both androgens and estrogens seem to regulate bone turnover in younger men. However, only estrogen deficiency was associated with bone loss at the spine and cortical bone loss at peripheral sites, detected after 16 weeks by HR-pQCT . No trabecular bone loss was seen at peripheral sites after 16 weeks in this trial.
In summary, these findings provide compelling evidence that estrogens are the primary regulators of bone loss in men, and that androgens alone cannot compensate for the loss of estrogens. However, the bone turnover marker findings leave open the possibility that androgen signaling plays a role specifically in inhibiting trabecular bone resorption, as seen in rodent models . Furthermore, these and other studies have not been designed to examine the question of whether androgens are involved in the gender difference in periosteal bone expansion in humans .
25.3.3.3
Role of estrogen receptor beta
Estrogens can also activate ERβ (encoded by the ESR2 gene) and GPER1 (G protein–coupled ER1, formerly known as G protein–coupled receptor 30, GPR30) . One study reported increased trabecular bone volume and cortical thickness in male GPER1-KO mice , which was not seen in females . GPER1 has been reported as being expressed in osteoblasts, osteoclasts, osteocytes, and chondrocytes. However, a GPER1 agonist recently failed to limit bone growth in mice or murine bone explants , and cell-specific KO models are lacking to confirm a direct role of GPER1 in skeletal biology.
Several studies suggest a role for ERβ on bone loss as well as on the growth plate in female mice, with an inhibitory effect on ERα . However, male ERβKO mice show no bone phenotype and male ERαβKO mice show no difference compared to ERαKO alone . In human bone biopsies, cortical bone appears to express mainly ERα, while ERβ stains intensely only in trabecular bone . In line with these findings, Nicks et al. have shown that conditional deletion of ERβ in osteoblasts markedly increased trabecular (but not cortical) bone volume in female (but not male) mice . Thus ERβ appears to regulate trabecular bone in female while it appears to be redundant in male animal models.
Still, any effect in humans remains to be confirmed. One recent study suggested that failure of ovarian development and concomitant osteoporosis in a 16-year-old girl was associated with a heterozygous, dominant-negative ESR2 mutation . However, this patient was also estrogen deficient and improved her BMD Z-scores with estrogen replacement therapy, arguing against a direct involvement of ERβ in bone. Previous candidate gene studies have also suggested a role for ERβ in osteoporosis , but more recent GWAS metaanalysis have not confirmed this. All in all, ERα appears to play a dominant role in estrogen signaling.
25.3.3.4
Nonclassical actions of sex steroid receptors
Following their classical ligand-induced activation, sex steroid nuclear receptors translocate into the nucleus, recruit coactivator proteins, and regulate gene transcription by direct binding as homodimers to androgen or estrogen response elements (AREs/EREs).
The role of coactivators in the skeletal response to sex steroids has received little attention. Female steroid receptor coactivator (SRC)-1 and SRC-2 KO mice display partial skeletal resistance to E2 . Male SRC-1-KO mice show trabecular osteopenia and have been reported as resistant to DHT although not to E2 .
Several alternative mechanisms of action have been identified for ERα and, to a lesser extent, for AR:
- 1.
heterodimerization with other transcription factors, resulting in either reduced DNA binding (hijacking) or indirect DNA binding (tethering) resulting in increased or decreased transactivation;
- 2.
ligand-independent activation (mainly via phosphorylation of part of the nuclear receptor called activation function 1, AF-1); and
- 3.
rapid nongenomic (nonnuclear) effects, for example, by signaling of ERα at the cell membrane .
Both the full-size 66 kDa ERα as well as its splice variants ERα46 and ERα36 (which lack AF-1) have been localized in plasma membranes ( Fig. 25.7 ). Nongenomic signaling can be mediated by cytoplasmatic kinases such as extracellular signal-regulated kinases (ERKs), which phosphorylates other transcription factors to either induce (e.g., ELK-1) or repress [e.g., c-Jun/c-Fos (AP-1)] their transactivational properties . These mechanisms have been investigated as a means to selectively exploit the beneficial effects of estrogens in postmenopausal osteoporosis while avoiding some of its unwanted reproductive and possibly cardiovascular side effects.
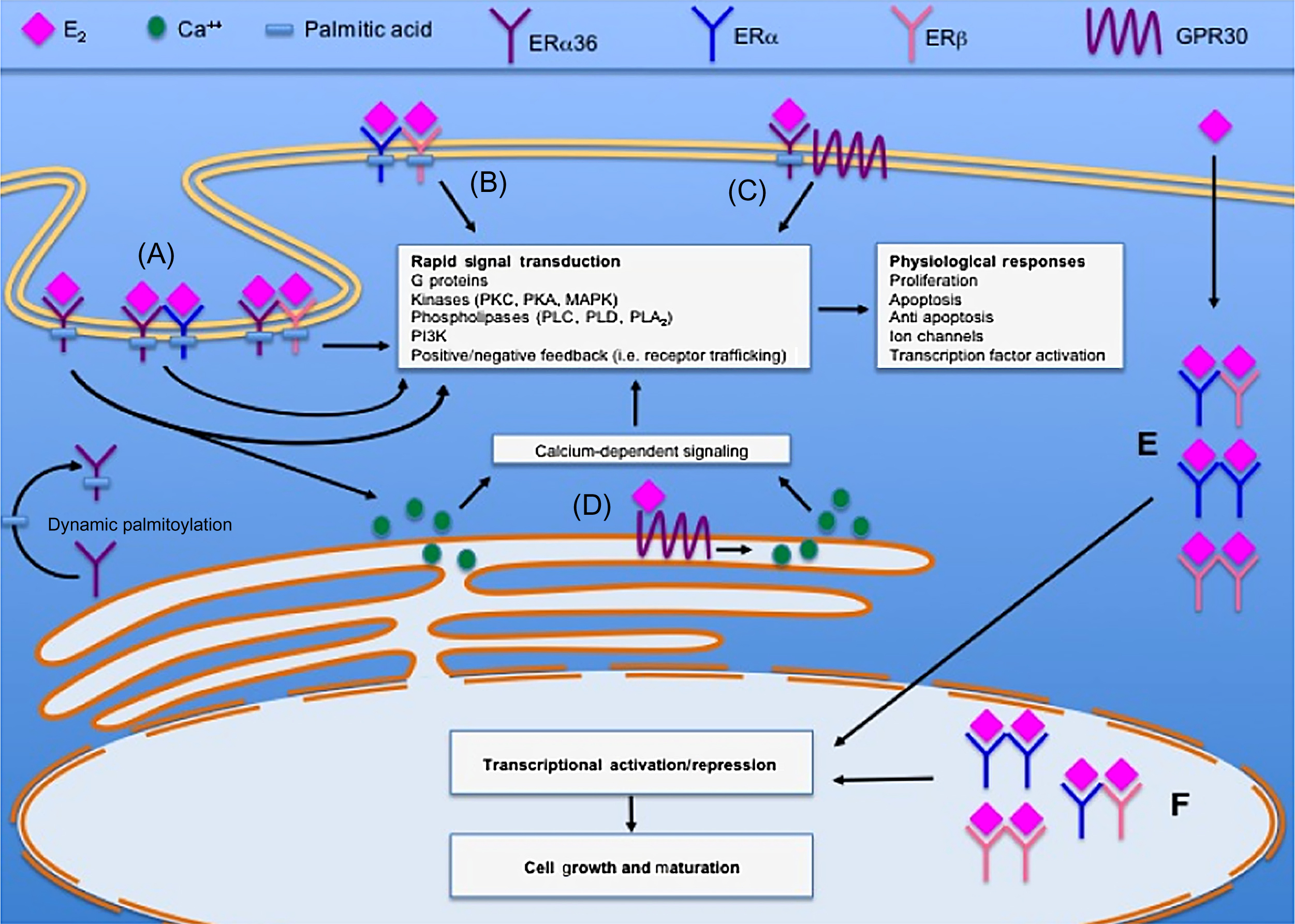
Indirect DNA binding via other transcription factors
Both AR and ERα can bind to other transcription factors and regulate activity on DNA response elements of the latter. For example, both androgens and estrogens prevent bone resorption by repressing interleukin-6 (IL-6) expression in osteoblasts and bone marrow stromal cells . Recently, it has been shown that soluble IL-6 receptor mediates the increase in osteoclasts and trabecular bone loss following ovariectomy, whereas IL-6 is not involved in cortical bone loss . ERα does so independent of EREs but via direct binding to nuclear factor kappa-B (NF-κB), which binds to an NF-κB binding site in the IL-6 promotor to repress its transcription . There is also mutually repressive interaction between ERα or AR and CCAAT enhancer-binding proteins (C/EBP), inhibiting the expression of, for example, IL-6 or IGF-1 .
Another example is the interaction of ERα or AR with RUNX2 (formerly CBFA1), an essential transcription factor in osteoblast and chondrocyte differentiation. A biphasic effect has been reported. In early-stage osteoblasts (when RUNX2 activity is low), E2-liganded ERα can bind to RUNX2, which results in greater transactivation via RUNX response elements, but less activity on EREs . In mature osteoblasts however (when RUNX2 activity is high), ERα binding to RUNX reduces activity of the latter . Similarly, heterodimerization between the AR and RUNX2 has been reported as repressing transactivation by the other protein . This mechanism may contribute, at least in part, to the antiresorptive effects of sex steroids exerted via osteoblast ( Section 25.3.3.5 ). This hypothesis requires confirmation but is supported by the finding that transgenic mice expressing a dominant-negative RUNX2 (loss-of-function model) resist ovariectomy-induced bone loss .
Nongenomic effects
As mentioned earlier, nongenomic (or nongenotropic) sex steroid signaling involves activation of cytoplasmatic kinases, which phosphorylate and regulate other transcription factors such as ELK-1, AP-1, C/EBPs, or cAMP response element-binding protein (CREB) . Following the initial discovery of this pathway, several therapeutic strategies have been designed to exploit nongenomic estrogen signaling.
Initially, a synthetic estrene compound was proposed as exploiting nongenotropic sex steroid signaling, but it was later found to act like a SERM with transcriptional activities . An estrogen dendrimer conjugate (EDC) however provides steric hindrance preventing nuclear and allowing only nonnuclear estrogen signaling . The group of Manolagas has shown that EDC was as efficient as an equimolar dose of E2 in preventing cortical (although not trabecular) bone loss in ovariectomized mice . Similarly, EDC maintained cortical thickness (but not trabecular bone) in orchidectomized mice .
In male mice with a mutant AR (absent second zinc finger and incapable of DNA binding), DHT treatment induced ERK-1/2 phosphorylation via a rapid nongenomic mechanism. In vivo, DHT suppressed cortical bone expansion in this model, opposite to the physiological effect of androgens .
Similarly, mice with a single knock-in ERα incapable of DNA binding (ERα[-/NERKI] mice) have been studied. A caveat of this model however, is increased circulating T in females (but not in males) . Female NERKI mice had cortical (but not trabecular) bone deficits and a paradoxical response to ovariectomy and E2 replacement . Male NERKI mice showed reductions in both cortical as well as trabecular vBMD .
The opposite mouse model has a knock-in mutated ERα lacking a palmitoylation site resulting in absent membrane localization (nuclear-only ERα, NOER mice), with markedly reduced rapid signal transduction. Female NOER mice display a strongly reduced vertebral trabecular bone response to estrogen, and a partly reduced appendicular cortical and trabecular estrogen response . Similarly, male NOER mice showed reduced cortical and trabecular thickness, with a diminished response to estrogens .
Collectively, these findings suggest that both genomic and nongenomic ERα signaling are required for optimal development of the male skeleton. However, the findings in NERKI and NOER mice are somewhat contradictory, and further investigation in novel models such as H2NES will be of interest .
Ligand-independent activation and the role of estrogen receptors alpha activation function 1
From an evolutionary perspective, ERα may have emerged as a ligand-independent transcription factor, whereas AR may have emerged only after androgens were produced . In vitro, ligand-independent activation is clearly evident for ERα in bone , while the AR is mainly if not entirely a ligand-dependent transcription factor. Also in vivo, ERE-luciferase mice show transcriptional activity in bone, even when treated with aromatase inhibitors .
Almeida et al. showed both in vitro and in vivo that the unliganded ERα potentiates Wnt signaling in osteoblasts, subsequent osteoblastogenesis, and periosteal bone formation, whereas E2 had the opposite effect, that is, restraining osteoblastogenesis and alkaline phosphatase activity . The osteogenic effects of mechanical loading on cortical bone also require ERα but are independent of estrogens . These findings show that the important anabolic effects of ERα on cortical bone may involve a ligand-independent mechanism.
Ligand-independent nuclear translocation of ERα may be triggered in a region of the N-terminal domain (NTD) called AF-1, by protein interactions or phosphorylation by several kinases (e.g., mitogen-activated protein kinase, protein kinase B (AKT), mitogen-activated protein kinase kinase (MEK1/2), and extracellular signal-regulated kinase (ERK-1/2)). Within the ligand-binding domain on the other hand, AF-2 is a ligand-dependent interaction surface for coregulator proteins or interactions with the NTD .
Several studies have dissected whether the effects of ERα require either AF-1 or AF-2. Female or male mice lacking AF-2 lack responsiveness to E2 (even though E2 binding to ERα is unaffected), demonstrating the essential role for AF-2 in ligand responsiveness . AF-1 deletion abrogates estrogen responsiveness only in trabecular bone and not in cortical bone in both female and male mice, with only weak effects on the uterus in females . Interestingly, the effects of EDC on cortical bone in male mice (see previous paragraph) also require AF-1 . Finally, AF-1 is required for the skeletal response to SERMs in female mice .
We can conclude that both AF-1 and AF-2 are required for the full effects of ERα, although tissue-specific ERαAF-1 or AF-2 modulators might retain some protective effects on the skeleton with less side effects on other tissues.
25.3.3.5
Target cells
ERα, ERβ as well as GPER1 (see Section 25.3.3.3 ) are expressed on osteoblasts, osteocytes, and chondrocytes as well as osteoclasts. The AR is also expressed on osteoblasts and even more so on osteocytes . Chondrocytes also express AR although its physiological role has not been proven in cell-specific KO models. The expression of AR by osteoclasts appears however to be negligible , with conditional deletion models suggesting no physiological relevance .
The bone phenotype in KO animal models with (global) deletion of sex steroid receptors in all of their tissues tells us something about the overall physiological role of this receptor, but it does not exclude the possibility that the bone phenotype results indirectly from the effects of these receptors in other cells or extraskeletal tissues. Therefore conditional KO models have been used to investigate the direct target cells of the effects of ERα, ERβ, and AR on cortical and trabecular bone. These models are mostly based on cycling recombinase (Cre)-LoxP technology, in which expression of a Cre driven by a cell-specific promotor can excise a crucial part of a target gene flanked by two LoxP-sites within a specific cell lineage and all descendant cells . The opposite approach involves cell-specific gene overexpression, sometimes in combination with a global deletion genetic background . Despite several caveats and hard to explain differences in results, these models have considerably advanced our knowledge regarding the target cells of sex steroid effects on male and female cortical and trabecular bone over the last 15 years .
Target cells of estrogen receptors alpha and beta on male versus female cortical and trabecular bone
The findings of different cell-specific ERα or ERβ KO or overexpression models are summarized in Table 25.1 . It should be noted that theoretically, conditional deletion at one stage of maturation may affect all descendant cells, for example, deletion at the osteoprogenitor stage may still produce a phenotype that relies on effects mediated by osteocytes.
Target cells | Cortical bone | Trabecular bone | Ref. |
---|---|---|---|
ERα | |||
Osteoclasts | |||
Late (Ctsk-Cre) | Female= | Female ↓ | |
Male= | Male= | “ | |
Precursors (LysM-Cre) | Female= | Female ↓ | |
Male= | Male= | ||
Precursors (LysM-Cre) | Female= | ||
Osteoblasts | |||
Osteoprogenitor cells (Prx1-Cre) | Female ↓ | Female= | |
Male: transient ↓ | Male= | “ | |
Osteoprogenitor cells (Osx-Cre) | Female ↓ | Female= | |
Male= | Male= | ||
Differentiating osteoblasts (Runx2-Cre) | Female ↓ | ||
Mature osteoblasts (Col1a1-Cre) | Female= | Female= | “ |
Male= | Male= | ||
Mature osteoblast overexpression (Col1a1-Cre, ERα constitutively active) | Female ↑ | ||
Mature osteoblasts (Osteocalcin-Cre) | Female ↓ | Female ↓ | |
Male= | Male ↓ | “ | |
Mature osteoblasts (Osteocalcin-Cre) | Female ↓ | Female ↓ | |
Male ↑ | Male ↑ | ||
Osteocytes | |||
Dmp1-Cre | Female= | Female ↓ E2 response | |
Male= | Male ↓ | ||
Dmp1-Cre | Female= | Female ↓ | |
Lymphocytes | |||
T-cells (Lck-Cre) | Female= | Female= | |
B-cells (CD19-Cre) | Female= | Female= | |
Neuronal cells | |||
Nestin-Cre | Female ↑ | Female ↑ | |
POMC-Cre | Female ↑ | Female= | |
Hypothalamic VMN silencing | Female= | Female= | “ |
Nkx2-1Cre, Kiss1-Cre | Female ↑↑ | Female ↑↑ | |
ERβ | |||
Osteoblasts | |||
Osteoprogenitor cells (Prx1-Cre) | Female= | Female ↑ |
Some discrepancies between these studies are immediately evident. For example, two independent groups showed that cell-specific deletion of ERα in either early or late osteoclasts recapitulates the trabecular but not the cortical bone loss following estrogen deficiency in female mice. Another study did not confirm this, although the investigation was much less thorough . In any case, the available evidence consistently shows no direct role of osteoclast ERα in males.
Regarding the osteoblast lineage, Almeida et al. convincingly showed that ERα in osteoprogenitors but not mature osteoblasts mediates cortical bone accrual in female but not male mice, although they did observe a transient reduction in cortical bone expansion in males . However, the validity of their Col1a1-Cre has been questioned by others, who showed using Osteocalcin-Cre that ERα in mature osteoblasts regulates female cortical bone, and using Runx2-Cre, Osteocalcin-Cre, or Dmp1-Cre that trabecular bone was also compromised in female mice. It should be noted however that the same Osteocalcin-Cre and Dmp1-Cre were each used in two studies producing conflicting results, which were in part attributed to differences in genetic background and the ERα flox/flox model used . Nevertheless, a constitutively active ERα overexpression model using Col1a1-Cre further supports a role for ERα in suppressing trabecular bone resorption in female mice, by decreasing IL-6 and increasing osteoprotegerin (OPG) expression in osteoblasts . Likewise, osteoblast-specific aromatase overexpression enhanced cortical and trabecular bone as well as OPG, while reducing osteoclast numbers in female mice . Although this was not accompanied by systemic disturbances, theoretically this phenotype could result from local effects of E2 on other cell types.
In male mice on the other hand, one group showed that ERα in the osteoblast lineage influences neither cortical nor trabecular bone . In contrast, Määttä et al. and Windahl et al. provided evidence suggesting that trabecular bone formation is stimulated by ERα in osteoblasts and osteocytes, respectively. Melville et al. further illustrate the pitfalls of the Osteocalcin-Cre model, which has expression in chondrocytes. This likely explains their observed bone length phenotype, which may explain their paradoxical increase in cortical and trabecular bone mass in male mice .
It is important to emphasize the possible role of other bone cells than osteoclasts, osteoblasts, and osteocytes. Using a different approach of hematopoietic stem cell transplantations and reconstitution between wild-type and global ERαKO mice, Erben’s group suggested that bone lining cells are important regulators of RANKL-mediated bone resorption following estrogen deficiency . Ucer et al. recently suggested that bone marrow stromal cells contribute to bone resorption following estrogen deficiency via the osteoclastogenic cytokine SDF1 (stromal cell–derived factor 1, a.k.a. CXCL12) .
Findings from conditional KO models in other models, specifically cells in the immune or nervous system, are discussed in Section 25.3.3.7 . Notably, ERα appears to play a counterregulatory effect in the cells, opposite to its whole-organism effects.
In general, we can conclude that the effects of ERα on bone are cell-, gender-, and compartment-specific. ERα in osteoclasts mediates trabecular (but not cortical) bone loss in female (but not male) mice. ERα in the osteoblast lineage regulates cortical bone accrual in female mice, likely at the osteoprogenitor stage. In later stages of osteoblast differentiation, it may also contribute to trabecular bone formation in female and male mice. In any case, the target cell(s) for the effects of ERα on cortical bone in male mice remains enigmatic. ERα has been deleted in several other tissues, for example, muscle but any bone phenotype in these mice has not been reported. ERβ has a counterregulatory effect, that is, suppressing trabecular bone volume in female mice, possibly by the regulation of osteoprogenitor differentiation . Again, the effects in males remain unreported.
Target cells of androgen receptor on male versus female cortical and trabecular bone
The findings of different cell-specific ARKO or overexpression models are summarized in Table 25.2 .
Target cells | Cortical bone | Trabecular bone | Ref. |
---|---|---|---|
Osteoclasts | |||
Late (Ctsk-Cre) | Male= | Male= | |
Precursors (LysM-Cre) | Male= | Male= | |
Osteoblasts | |||
Osteoprogenitor cells (Prx1-Cre) | Male= | Male ↓ | |
Female= | Female ↓* | ||
Mature osteoblasts (Col1a1-Cre) | Male= | Male ↓ | |
Mature osteoblast overexpression (Col1a1-Tg) | Male ↓ | Male ↑ | |
Female ↓* | Female= | “ | |
Mature osteoblasts (Osteocalcin-Cre) | Male (↓) | Male ↓ | |
Mature osteoblasts (Osteocalcin-Cre) | Male= | Male ↓ | |
Female= | Female ↓ | ||
Osteocytes | |||
Dmp1-Cre | Male= | Male ↓ | |
Neuronal cells | |||
Thy1-CreERT2 | Male ↓ | Male ↓* | |
Satellite cells | |||
MyoD-Cre | Male= | Male ↓ |
Contrary to prior reports, AR expression in osteoclasts appears to be minimal if any, as well as functionally redundant . In contrast, AR is expressed in osteoblasts and even more so in osteocytes . ARKO in early and mature osteoblasts, and to a lesser extent in osteocytes, increases bone turnover and trabecular bone loss through increased resorption . Surprisingly, no changes in cortical bone were seen, except for a transient delay in periosteal expansion during puberty in one model . Interestingly, AR may also regulate mineralization by osteoblasts .
Wiren et al. created two different models with AR overexpression in osteoblasts . In line with the cell-specific KO models, they observed increased trabecular bone volume due to decreased resorption in male mice , with no change in periosteal circumference (although increased periosteal bone formation was noted at the calvaria in one model ). Furthermore, comparison between the two models supports the conclusion that mature osteoblasts and osteocytes mediate AR effects . Unexpectedly, cortical bone area was compromised in both of these osteoblast AR overexpression models, with reduced endosteal bone formation . Together with changes in bone length in the first model , these findings are difficult to interpret. However, later detailed examination led to the interesting hypothesis that androgens directly stimulate periosteal cells derived from neural crest rather than mesenchymal origin .
In any case, on the basis of these models, we can conclude that the AR in osteoblast-lineage cells is responsible for the antiresorptive effects on trabecular bone but not for its anabolic effect on periosteal bone formation in males. The target cells for the latter, most important effect of androgens on bone, thus remain unknown.
In search for extraskeletal target cells mediating the effects of androgens on bone, several other conditional ARKO models have been developed. Male mice with muscle-specific AR deletion also display trabecular but not cortical bone deficits, although only mice were not examined at different ages . Interestingly, AR deletion in extrahypothalamic neurons has recently been shown to accelerate age-related cortical thinning, as well as trabecular bone loss in the spine of male mice . Other cell-specific ARKO models have been developed including in fat cells or in other parts of the nervous system, including the hypothalamus , but the bone phenotype of the latter models is likely confounded by disrupted hypothalamic–pituitary–gonadal axis feedback.
Few studies have examined female cell-specific ARKO mice. Määttä et al. observed trabecular bone loss in female osteoblast-ARKO mice, while Ucer et al. noted reduced trabecular vBMD, trabecular number and increased trabecular separation. In contrast, Wiren et al. observed no trabecular phenotype in female osteoblast-specific AR-overexpressing mice . Although a direct effect of androgens on bone in females would be relevant in the context of, for example, polycystic ovary syndrome or trans men, the clinical importance of these findings remains uncertain .
25.3.3.6
Target genes
Ideally, a direct target gene can be defined as a gene, which is up- or downregulated following binding of AR or ERα in its promotor or enhancer regions within a few hours after ligand stimulation (typically, 3–6 hours), and is required for the physiological effect on bone in vivo . Considering how little is known about the target cells of sex steroids on bone, as well as the nongenomic mechanisms of their effects, it is not surprising that little is known with certainty about direct target genes.
For example, many studies have failed to explain altered bone turnover by changes in Rankl/Opg gene expression in the bones of conditional ARKO or ERαKO models discussed earlier. However, membrane and soluble RANKL have recently emerged as important regulators of bone formation and resorption . Mice lacking soluble RANKL display normal bone loss following ovariectomy, attesting to the importance of RANKL bound to the osteoblast membrane . Martin et al. showed that both E2 and DHT inhibit RUNX2-driven osteoblast secretion of RANKL and consequent osteoclastogenesis, by regulating membrane RANKL through a nongenomic, posttranslational, and trafficking mechanism . In addition, prolonged estrogen deficiency has been reported to upregulate Rankl expression by bone lining cells in vivo . Khosla’s group showed that estrogen deficiency increased the presence of RANKL on the surface of bone marrow stromal cells, B- and T-lymphocytes . Similarly, estrogen therapy and raloxifene suppress RANKL in peripheral blood mononuclear cells . However, by O’Brien et al. showed that osteocyte-derived RANKL is essential for ovariectomy-induced bone loss . In addition, RANKL released by B-lymphocytes (but not T-lymphocytes) contributes to ovariectomy-induced bone loss . Interestingly, bone marrow soluble RANKL concentrations increase following gonadectomy in both sexes, perhaps in part via increased shedding following upregulation of the matrix metalloproteinase-14 . The decoy RANKL receptor OPG-Fc mitigated not only trabecular and endocortical resorption but also the decline in periosteal bone formation after castration .
For ERα, it was originally proposed that estrogens induce osteoclast apoptosis via Fas ligand (FasL) in an autocrine manner . However, Krum et al. found that osteoclast survival was regulated in a paracrine fashion by ERα regulation of FasL in osteoblasts . They continued to show that FasL cleavage required matrix metalloproteinase 3, which is regulated by ERα . Others confirmed that conditional deletion of FasL in osteoblasts prolongs the life span of osteoclast and induces bone loss . However, others argue that the effects of the Fas/FasL system depend on the differentiation of osteoblasts , and that nongenomic effects are sufficient for the proapoptotic effects of E2 on osteoclasts .
In osteoblasts, ERα occupies unique genome-wide DNA binding sites (the so-called cistrome) and gene expression has been found <15% similar to other cells, for example, breast cancer cells . Although FoxA1 is a crucial pioneer factor in the latter cells, Krum’s group identified GATA4 as a key pioneer factor in osteoblasts . The alkaline phosphatase ( Alpl ) gene was identified as a direct transcriptional ERα target gene in these studies . Other target genes identified by chromatin immunoprecipitation include retinoblastoma-binding protein 1 , the cell adhesion molecule Svep1 , IGF-binding protein 4 , and Esr1 itself in a positive feedback loop . More recently, Ucer et al. reported that increased osteoclastogenesis in osteoblast-specific ERαKO mice (Prx1-Cre) was at least partly explained by upregulation of stromal-derived factor 1 (also known as CXCL12) .
Sex steroids have also been linked to Wnt/β-catenin signaling, with considerable sexual dimorphism in mouse models in which this pathway is modulated . Almeida et al. showed that ERα in osteoprogenitor cells potentiates Wnt signaling to stimulate proliferation and differentiation of periosteal cells, whereas estrogens inhibited it . Furthermore, estrogens as well as the SERM raloxifene downregulate circulating as well as skeletal mRNA expression of sclerostin and sclerostin domain-containing protein 1, another Wnt/bone morphogenetic protein inhibitor . One other previous study suggested that the stimulatory effects of androgens in osteoblast differentiation involve Wnt signaling .
In contrast to these molecular mechanisms identified mainly in osteoblasts in females, there is a paucity of evidence for molecular mechanisms in males, or for the effects of AR on bone.
25.3.3.7
Effects of sex steroids on bone via extraskeletal target tissues
Since cell-specific deletion of AR or ERs in bone cells does not fully explain their effects on cortical and trabecular bone in both genders, as outlined previously, several studies have examined whether these nuclear receptors regulate bone indirectly via other target cells or tissues. Although other target cells are under investigation, we will focus here on the most obvious candidates: muscle and fat, the nervous system, physical activity, the immune system, and the indirect effect via sex steroid regulation of the somatotropic axis.
Muscle
The role of muscle–bone interactions has gained increasing attention . This has led some critics to argue that the effects of androgens on bone can be explained entirely via the well-known effects of androgens on muscle . However, Laurent et al. have shown that while selective deletion of the AR in the satellite cell lineage induces trabecular bone loss, the antiresorptive effects of androgens are mediated independently of muscle AR . There is also evidence for a role of estrogens in muscle physiology in female rodents , but whether this has downstream consequences for bone remains a matter of conjecture. Furthermore, androgenic effects appear to explain the well-known muscle anabolic effects of T in men .
Fat
It has also become clear recently that sex steroids may regulate the commitment of mesenchymal progenitor cells toward either osteoblast or adipocyte differentiation. Indeed, bone loss in either androgen or estrogen deficiency as well as ARKO, ERαKO, or aromatase-KO models is accompanied by increased adiposity, both in classical fat depots as in bone marrow fat, as well as diabetes-like metabolic disturbances .
One clinical trial in postmenopausal women using magnetic resonance imaging found that estrogen quickly reduced vertebral bone marrow fat fraction, well before any bone mass changes were expected to occur . Also in the AGES–Reykjavik cross-sectional cohort, both T and E2 were associated with bone marrow fat fraction in the elderly . However, further work is needed to convincingly demonstrate that sex steroids’ regulation of bone metabolism requires their regulating of progenitor cells’ allocation to the osteoblast versus adipocyte lineage.
Nervous system
Both central and peripheral nervous systems have emerged in recent years as powerful regulators of bone mass, and both express AR and ERs.
Ohlsson et al. using a controversial Nestin-Cre showed that ERα deletion in nervous tissue increased cortical and trabecular bone volume in female mice , in line with other studies suggesting an opposite effect of central versus peripheral sex steroid regulation of bone mass. Further work showed that ERα deletion in pro-opiomelanocortin (POMC) neurons, but not in the ventromedial nuclei of the hypothalamus where it is also highly expressed, enhanced E2 responsiveness in female mice . Recently, both stereotactic and Cre-mediated ERα deletion has identified Kiss1 neurons in the arcuate nucleus of the hypothalamus as a powerful inhibitor of bone strength in female but not male mice .
We can conclude that ERα actions in the central nervous system inhibit cortical and trabecular bone formation in female mice, with findings in male rodent models eagerly awaited.
Physical activity
Sex steroids are important regulators of behavior and gender differences therein in many species, including humans, for example, the “steroid rage” effect of T abuse. Previous study clearly showed that female rodents display greater spontaneous locomotor activity than males, with lower activity in gonadectomized or ARKO mice . Conversely, physical activity remained effective to restore the detrimental bone phenotype in ARKO mice . ERαKO in medial amygdala neurons led to marked weight gain due to reduced physical activity . Recent studies show that neuronal- or hypothalamic-ARKO mice are less physically active while selective deletion in extrahypothalamic regions did not influence physical activity . T may regulate physical activity behavior in these male mice in part via central dopaminergic pathways .
We can conclude that sex steroid deficiency reduces spontaneous physical activity in animal models, which may have downstream detrimental effects on bone. However, this hypothesis requires confirmation in humans.
Immune system
There is strong evidence linking estrogen-related bone loss to the immune system in females. Estrogens induce thymus atrophy while androgens inhibit bone marrow B lymphopoiesis in male mice , in part via the AR in osteoblasts . Mice lacking T-lymphocytes are protected from ovariectomy-induced bone loss, which is mediated at least in part by their release of tumor necrosis factor-α (TNF-α) . However, others have shown that some strains of mice lacking T-cells lose bone following ovariectomy , and ovariectomy-induced bone loss is mitigated in mice lacking RANKL in B-lymphocytes not T-lymphocytes . In postmenopausal women, estrogens suppress inflammatory gene expression, including NF-κB complex genes NFKB2 and v-rel avian reticuloendotheliosis viral oncogene homolog B (RELB) . Conversely, TNF-α blockade using etanercept mitigates bone resorption following acute estrogen deprivation . The gut microbiome, which regulates the immune system, has also emerged recently as a regulator of bone mass. Indeed, germ-free mice have increased bone mass, and probiotics can mitigate ovariectomy-induced bone loss (see Chapter 39 : Bone and the microbiome).
Chimeric wild-type and ERαKO hematopoietic stem cell reconstitution studies such as described previously have shown that nonhematopoietic cells are essential for the cortical and trabecular bone response to E2 in female mice, although these effects are almost halved in the absence of hematopoietic ERα . Another recent study from Ohlsson’s group adds to the evidence that T-cells are not direct target cells mediating this effect of ERα , leaving the likely possibility that B-lymphocytes are responsible in this regard.
Of note, both AR and ERα also regulate the immune system in males . Whether this contributes to their bone loss remains hitherto unknown.
Role of growth hormone and insulin-like growth factor-1
Both androgens and estrogens regulate the somatotropic axis. In older men, androgens appear to regulate growth hormone (GH) pulse frequency and duration, whereas aromatization regulates IGF-1 secretion .
The bone phenotype in many global ARKO, ERαKO or aromatase-KO animal models could theoretically be related in part to disturbances in the somatotropic axis (reviewed in Ref. ). Several studies have suggested that estrogens or androgens regulate osteoblast IGF-1, IGF-binding proteins (IGFBPs), or IGF-2 receptor; however, the evidence remains conflicting . Callewaert et al. established that skeletal sexual dimorphism was established in early puberty and requires downstream actions of the GH receptor, whereas androgens and estrogens independently regulated periosteal bone formation and expansion in late puberty . Many epidemiological studies have found associations of IGF-1 or IGFBPs with bone turnover, BMD or fracture risk in one, the other, or both genders . However, whether cross talk with the GH/IGF axis contributes to bone loss in postmenopausal women, older men, or following sex steroid deficiency remains largely unexplored .
25.4
Diagnosis and treatment of osteoporosis in men
25.4.1
Diagnosis
The work-up for osteoporosis can be considered a three-tiered process: (1) diagnosis, (2) exclusion of secondary causes, and (3) fracture risk assessment (see Chapter 61 : Evaluation of the osteoporosis patient). First, the diagnosis of osteoporosis needs to be established, either for secondary prevention in patients with prevalent fractures, or for primary prevention in men or women at risk for fractures because of low bone density, predisposing diseases, or other clinical risk factors . The diagnosis of osteoporosis can be made on clinical grounds following a low-trauma fracture in postmenopausal women or men aged 50 years or older (even when they have “just” osteopenia on DXA ), when other bone disorders (e.g., pathological fracture from malignant bone disease) have been excluded.
25.4.1.1
Laboratory testing
Like with any disease, evaluation starts with a clinical history and (focused) physical examination. Comorbidities, medications, smoking, and alcohol abuse are the commonest causes of secondary osteoporosis.
Guidelines in male osteoporosis further recommend a limited set of blood tests, which are important either to guide treatment choices (e.g., calcium, 25-hydroxyvitamin D, creatinine levels) and/or to identify treatable (possibly occult or subclinical) underlying causes of secondary osteoporosis (e.g., complete blood count, liver tests, phosphate, alkaline phosphatase levels, 24-hour urinary calcium/creatinine ratio to detect idiopathic hypercalciuria) . Hyperthyroidism (subclinical or overt) and monoclonal gammopathy are other fairly common biochemical abnormalities found in 2%–6% of osteoporotic men in clinical series .
Notably, clinic-based studies have reported high prevalences (~50%) of “secondary osteoporosis” in older men compared to ~30% in postmenopausal women , leading to the widespread idea that secondary osteoporosis is more common in men . However, this claim is likely unsubstantiated since there are few direct comparisons between genders. Referral bias is likely, since in fracture liaison services there is no evidence for a gender imbalance in secondary osteoporosis . In contrast, some secondary causes such as primary hyperparathyroidism are known to occur more frequently in women . Furthermore, in population-based studies, most of these tests are not more commonly abnormal in osteoporotic than nonosteoporotic men, with the possible exception of 25-hydroxyvitamin D and alkaline phosphatase . Lack of a clear definition of what constitutes “secondary” osteoporosis further complicates this issue .
There is some controversy regarding the usefulness of T measurements in male osteoporosis. In a clinical population, Ryan et al. found a high prevalence of hypogonadism (22.6%–25%) among osteoporotic men, while prior studies reported 8%–10% prevalences . In US veterans with spinal cord injuries, hypogonadism was even more common (52%) than hypovitaminosis D (50% prevalence) . In contrast, results from the MrOS studies suggest that T measurements have limited clinical utility in older men . These findings may not be generalizable to younger men (particularly <50 years of age) or those in which history and/or physical examination raises suspicion of secondary causes. Furthermore, guidelines suggest T replacement therapy only for symptomatic hypogonadism but not for osteoporosis per se, given the lack of proven antifracture efficacy of T therapy. Thus in asymptomatic men (particularly ≥70 years), a finding of male hypogonadism might not have therapeutic implications.
25.4.1.2
Dual-energy X-ray absorptiometry
For primary prevention a T-score ≤−2.5 at the hip or lumbar spine has been proposed to identify postmenopausal women at high risk of fractures, based on expert opinion at a WHO meeting . In premenopausal women or men below age 50 years, stricter criteria need to be applied, particularly in the absence of hip or vertebral fractures for example, to distinguish osteoporosis from low PBM . Indeed, low BMD has been observed in sons and daughters of older men or women with osteoporosis, and even continuous declines in hip BMD early after PBM may be physiological . Therefore osteoporosis cannot be diagnosed in men younger than 50 years based on BMD alone .
Previously, there was some controversy regarding the use of gender-specific T-scores in men, colloquially known as the “T-score debate” . However, the International Society of Clinical Densitometry (ISCD) and others now unequivocally recommend the use of DXA T-scores based on a single normative database (NHANES III) of 20- to 30-year-old White US women for comparison in other populations including in men . This threshold corresponds to a gender-specific T-score of −2.75 . However, the rationale to use female reference values in both genders is that for the same absolute BMD value (which implies a relative deficit in men, since they should have higher BMD), men have similar bone strength and fracture risk . This is in line with the fact that fracture risk is on average lower in men, but the relative risk of low BMD (particularly at the total hip site) is higher in men. Each standard deviation (SD) decrease in total hip BMD is associated with a 3.2-fold increased risk of hip fractures and 1.6-fold for nonvertebral fractures in older men, which indicates a greater hazard ratio than in women ( P ≤.01 for gender interaction) .
Unfortunately, a T-score of ≤−2.5 alone lacks sensitivity, but more so in men: in different prospective cohort studies, this criterion identified only 27%–34% of women and 6%–21% of men with osteoporotic fractures . However, the use of gender-specific T-scores in men would lower specificity and increase disease prevalence. Notably, a recent randomized trial showed that antiresorptive therapy reduced fracture risk and height loss in postmenopausal women with osteopenia . However, similar evidence in men remains lacking.
25.4.1.3
Screening for male osteoporosis
The ISCD recommends DXA screening in women aged 65 years and men aged 70 years and older, or at younger ages in those with additional risk factors such as low body weight, prior fracture, high-risk medication use or disease known to cause secondary osteoporosis . Cost-effectiveness modeling suggests that universal screening of men, even from ages 50 or 60 years and relying upon age, femoral neck BMD, and VFA would be cost-effective . Use of simple prescreening tools such as the Osteoporosis Self-Assessment Tool (OST) or Male Osteoporosis Screening Tool (MOST) would reduce the need for DXA, with a reported sensitivity of 83%–90% but with low specificity . Several recent trials have investigated the usefulness of screening for osteoporosis in postmenopausal women , but no similar trials are available in men. However, the US Preventive Services Task Force recommends against screening for osteoporosis in men not due to lack of screening trials, but primarily based on the absence of clinical trials showing reduced clinical fracture risk in men , which is inappropriate in our view as well as in the view of other experts .
25.4.1.4
Beyond bone mineral density: novel radiological methods
Apart from DXA BMD, several alternative techniques have been investigated in an attempt to improve fracture risk prediction. As noted earlier ( Section 25.3.2.4 ), studies on gender differences in bone material properties (e.g., using nanoindentation) are few, discordant, and do not currently support a role for “bone qualities” other than cortical and trabecular bone structure in gender differences in fracture risk.
Quantitative ultrasound measurements at the heel predict fractures in both genders independently of DXA but are not well validated for treatment and unsuitable for monitoring during treatment .
TBS, a texture-based measurement based on lumbar spine DXA images, can improve fracture prediction in both men and women . In clinical practice, it can be used to adjust FRAX scores (see next), although the effect is often small. In the Geelong Osteoporosis Study for example, TBS-adjusted FRAX was no better than unadjusted FRAX for fracture prediction in men .
Although not necessarily better for clinical fracture prediction than DXA, hip and/or spine QCT measures predict hip and spine fractures in both genders . Notably, men without or with incident hip fractures still have greater finite element analysis (FEA)-estimated proximal femoral strength than women without or with such fractures, respectively. However the difference between control and fracture cases appears greater in men . QCT reveals that the rate of structural bone decay and estimated strength loss is more than double in postmenopausal women compared to men, with the greatest difference in cortical bone . Interestingly, trabecular vBMD appears to be somewhat more predictive of fractures in men, possibly because it is more sensitive to changes . Vertebral and femoral strength estimated by FEA based on either DXA or central QCT also have predictive values for vertebral and hip fractures, but they are not significantly better than DXA in both genders . Hip QCT–derived measures like trochanteric vBMD and buckling ratio predict incident hip fractures in men independent of aBMD, but their predictive added value remains modest (e.g., in the MrOS study, area under the receiver–operator curve increased from 0.863 to 0.901, P <.005 for adding trabecular vBMD to a model with age and total hip aBMD) . Statistical shape and density models, cluster analysis or machine learning–based algorithms based on (HR-p)QCT might improve fracture predictions in the future, however, they require independent validation . QCT measurements are not available in most clinical settings, but methods exist to derive DXA-equivalent T-scores as well as FEA-derived bone strength from routine abdominal or pelvic CT scans . Efforts are needed to implement such strategies worldwide.
HR-pQCT has also been investigated for fracture risk assessment and selection of candidates for osteoporosis treatment in both genders. Both in MrOS and STRAMBO, HR-pQCT measures as well as estimated failure load predicted incident fractures in men, although their added predictive value (beyond DXA and clinical risk factors) is again modest (as in women) . Older men with accelerated bone loss (≥10% hip aBMD loss) have worse cortical and trabecular bone parameters on HR-pQCT at the radius or tibia . A recent metaanalysis confirmed the independent predictive value of HR-pQCT for fractures in older men and women (particularly FEA-derived failure load, or models combining cortical and trabecular indices), although pooled gender-specific data have not been reported .
We can conclude that novel techniques to evaluate fracture risk appear promising in both genders. Currently however, DXA is still preferable because these novel methods are not widely available, may have modest additional predictive value at best, and have not been validated in clinical trials to select candidates for the treatment of osteoporosis.
25.4.1.5
Bone turnover markers
Findings on the sexual dimorphism in BTMs depend on the type of BTM analyzed. Some BTMs such as N-terminal propeptide of type I procollagen (PINP) tend to be higher in men 20–30 years of age, similar around age 50, and higher in postmenopausal women . However, Khosla et al. reported that osteocalcin and serum N-Telopeptides of Type I Collagen (NTX) were higher in men before age 50 but similar after that age . BTMs are significantly but weakly associated with aBMD or vBMD loss. The few available studies are conflicting regarding independent associations of BTMs with fracture risk in men; hence, the evidence base is insufficient to recommend them for this purpose, as is still the case in women . Both in the prospective MrOS and MINOS studies, high bone turnover was associated with accelerated bone loss but not independently with fracture risk . In the STRAMBO study, BTMs were more strongly associated with bone microarchitecture in men after the age of 70 years . Lower BTMs have however also been associated with relative bone deficits, for example, in men with metabolic syndrome and/or insulin resistance . In the AGES–Reykjavik study, BTMs more closely correlated to cortical than trabecular bone loss in men . These findings are in line with the fact that >80% of the skeleton comprises cortical bone , and an important study using postmortem femur bone samples showing that most bone loss in postmenopausal women is cortical . Unfortunately, comparable high-quality biopsy studies on cortical versus trabecular bone loss at the femur in men remain lacking (see next paragraph).
25.4.1.6
Bone biopsy
Bone biopsy studies in male osteoporosis are few. One study found that trabecular bone volume, trabecular thickness, and osteoid thickness were lower in osteoporotic than healthy control men, while remodeling rate and erosion depth were similar, thus suggesting osteoblast dysfunction . Bone biopsy reveals poorer trabecular microstructure in osteoporotic men with compared to those without vertebral fractures . Given its invasive nature, limited additive clinical value, and lack of availability of rigorous high-quality bone histomorphometry in most clinical settings, bone biopsy is not recommended in the work-up of idiopathic male osteoporosis, unless a specific alternative diagnosis is suspected, for example, malignancy, mastocytosis , and osteomalacia.
25.4.2
Treatment of male osteoporosis
25.4.2.1
Fracture risk assessment
Since osteoporosis treatment can be considered synonymous with prevention of fractures, fracture risk assessment is the cornerstone based on which treatment candidates are selected.
As in women, men with previous hip or vertebral fractures have the highest risk of sustaining additional fracture and should be considered candidates for treatment . Many trials in male osteoporosis have selected men based on a T-score ≤−2.5 (of note: often a male-specific T-score!) and showed benefits in terms of BMD and fracture risk reduction (as outlined later) that were similar to results obtained in postmenopausal women. However, the T-score ≤−2.5 cutoff alone has high specificity but low sensitivity (as outlined previously). It is therefore reasonable to consider treatment in men aged 50 years or older with prior hip or vertebral fractures, central T-scores ≤−2.5, or in those with high fracture risk based on low BMD and/or clinical risk factors. This approach, which is similar to that in postmenopausal women, is also in line with current guidelines on male osteoporosis .
The latter approach of fracture risk assessment, including clinical risk factors, is the most controversial criterion to select candidates for treatment. Several tools have been developed for clinical fracture risk prediction, with or without incorporation of BMD results. Simpler tools such as OST and MOST have been used mainly to prescreen candidates for further DXA testing . The FRAX, Garvan and (in the United Kingdom) QFracture algorithms are the most commonly used fracture risk assessment algorithms. Some studies suggest that the use of FRAX or Garvan without BMD underestimates fracture risk or does not identify a large group of men in whom treatment might be considered based on T-scores ≤−2.5 . Although FRAX without BMD performed reasonably well in Norwegian men , another large population-based study found limited predictive ability of FRAX without BMD in men .
A small retrospective validation study suggested that although FRAX and Garvan had similar predictive ability in women, only Garvan was a good predictor in men . Furthermore, miscalibration and underestimation of fractures may be an issue when applying any tool in geographically diverse regions . In the large MrOS study, both FRAX and Garvan performed well and in the Manitoba BMD registry, FRAX was a strong and consistent predictor of major osteoporotic fractures and hip fractures in both genders . Major difference between the FRAX and Garvan calculators are that the latter incorporates falls risk and predicts not only the four “major” but also any osteoporotic fracture. QFracture (developed in UK primary care) predicts 3-year risk of hip or major osteoporotic fracture, but it is more complex. Findings from the MrOS study suggest that other risk factors for hip fractures in men should also be considered clinically, including height loss, use of tricyclic antidepressants, history of myocardial infarction or angina, Parkinson’s disease, and cognitive impairment . A major advantage of fracture risk assessment tools is that they can be used for shared decision-making with patients, and that they can allow recommendations for screening and treatment of men at absolute fracture risk thresholds similar to those in women. Although we believe that calculators should not replace clinical judgment, current guidelines recommend the use of FRAX, Garvan or other validated fracture risk assessment tools to identify men at high risk of fracture who might benefit from treatment . However, no randomized trials have shown fracture risk reductions in men recruited solely based on high fracture risk, which represents a major gap in current evidence.
25.4.2.2
Nonpharmacological measures
Encouragement of healthy lifestyle measures such as physical activity, smoking cessation, curtailing alcohol abuse, as well as interventions to prevent falls, are essential in both genders, not only to reduce the risk of fractures but also because of myriad other beneficial health outcomes. Smoking and alcohol abuse are among the commonest secondary causes of osteoporosis in men . Both current and former smoking (even “moderately”) has been independently associated with higher fracture risk in men, through associations with poor BMD and microstructure . One metaanalysis found higher risk ratios of smoking on fractures in men than in women , possibly because men have lower absolute fracture risk or because men smoke more heavily. Identification and treatment of other secondary causes may also have other health benefits (see Section 25.4.1.1 ).
Physical exercise has clear benefits to reduce the risk of falls . Exercise interventions have also been shown to increase BMD in men , including several group sports . However other studies were negative , which may be due in part due to the local osteogenic effects of exercise on certain regions of bones such as the femur or tibia . Aerobic exercise appears not to improve BMD , while resistance training and/or impact loading may be effective . Overall, the optimal physical exercise intervention and any protection against fractures require further study .
25.4.2.3
Calcium and vitamin D
In observational studies, vitamin D deficiency is associated with secondary hyperparathyroidism, higher bone turnover, lower BMD, higher risk of falls, and higher fracture risk (particularly hip fractures), in men as it is in women . Although 1,25-dihydroxyvitamin D levels have also been associated with skeletal outcomes in men , findings are more consistent and reliable with 25-hydroxyvitamin D . As noted earlier, the detrimental effects of sex steroid deficiency on male bone health were reinforced in the presence of vitamin D deficiency .
Recommendations for calcium intake and 25-hydroxyvitamin D levels are generally similar for older men and women. However, evidence in men remains scanty . One randomized trial found no effect of calcium and vitamin D supplements on BMD loss in older men ( n =86, with high baseline nutritional calcium intake, 1159 mg/day) . A 2×2 factorial trial found exercise but not vitamin D–fortified milk effective to increase femoral neck BMD and structure . However, in a larger trial, including n =176 men, calcium and vitamin D supplementation increased BMD at the hip and spine over 3 years, as it did in women . Only two men (both in the placebo group) sustained fractures in this trial. Calcium and vitamin D supplements have also been shown to mitigate BMD loss in trials on glucocorticoid-induced osteoporosis (which included men), in a study among army recruits and in patients initiating antiretroviral therapy for HIV (90% men) . However, as in women, benefits are most likely in elderly (particularly institutionalized) with pretreatment vitamin D deficiency and low calcium absorption.
In contrast, studies in the general population, including controversial reanalyses of the Women’s Health Initiative Trial and metaanalyses, have suggested possible cardiovascular safety concerns with calcium supplements. Although there is no convincing evidence for adverse cardiovascular events, this may merit further investigation in men, given that men are at higher risk of cardiovascular events. Several trials using intermittent megadoses of vitamin D have also reported a paradoxical increase in falls risk. Although similar trials in men are lacking, regular smaller doses are advisable.
Meanwhile, vitamin D supplementation in men with baseline low 25-hydroxyvitamin D levels, as well as sufficient calcium intake, preferably from dietary sources with supplements added as needed, is recommended in current guidelines . However, consensus remains lacking on the desirable reference range for 25-hydroxyvitamin D levels, partly due to assay variability . In the MrOS study, men in the lowest quartile of 25-hydroxyvitamin D (<20 ng/mL) had a higher risk of hip fractures .
25.4.2.4
Pharmacotherapeutics in male osteoporosis
The use of various bone-targeted drugs in male osteoporosis is discussed in detail in the respective Chapter 74, Chapter 75, Chapter 76, Chapter 77, Chapter 78, Chapter 79, Chapter 80, Chapter 81 in Part X, Pharmacotherapeutics. The role of T therapy specifically is discussed in detail in Chapter 79 , Androgens in the treatment of osteoporosis.
It should be noted that most pivotal randomized trials of osteoporosis drugs are performed in postmenopausal women, whereas for male osteoporosis as well as glucocorticoid-induced osteoporosis, smaller trials with BMD as the primary endpoint are usually performed, because the US Food and Drug Administration only mandates such trials and accepts extrapolation of evidence. Indeed, it should be clear from this chapter that pathophysiologically, there is little reason to assume that osteoporosis is a different disease in postmenopausal women and men. The BMD outcomes of such trials are almost invariably similar as in the pivotal trials in postmenopausal women. The one exception of a somewhat larger, better powered trial in male osteoporosis ( n =1199) showed a reduction in morphometric vertebral fractures and height loss in men treated with i.v. zoledronic acid compared to placebo . This is in line with other trials also reporting lower vertebral fracture risk and height loss with oral alendronate , and even less nonvertebral fractures in a trial with risedronate . Unfortunately, in some countries, the lack of trials powered for fracture outcomes is abused by regulatory agencies to curtail reimbursement for novel pharmacotherapies in male osteoporosis, that is, gender discrimination. We hope that this work may contribute to global efforts to enhance the understanding of male osteoporosis as a disease that is similar to its female counterpart in almost all of its aspects.
In summary, osteoporosis remains underdiagnosed and undertreated in men, despite the fact that they contribute considerably to the burden of this disease. The risk of osteoporotic fractures is lower in men, in part due to the effects of androgens during PBM acquisition and lack of a menopause equivalent. However, osteoporotic fractures in men carry a higher risk of mortality and higher refracture risk. Preclinical studies have clearly identified independent effects of the AR on male bone, although the target cells and target genes involved in these effects remain incompletely understood. The effects of sex steroids on the male musculoskeletal system are pleiotropic since muscle, fat, neuronal tissues, physical activity, the immune system, and the GH/IGF-1 axis are all influenced by sex steroids too. Still, T and E2 have only a limited role in the diagnosis and treatment of male osteoporosis, which is very similar to the approach in postmenopausal women.
Acknowledgment
The authors would like to dedicate this chapter to the memory of the late prof. Dr. Steven Boonen, who contributed substantially to the previous version of this chapter.
References
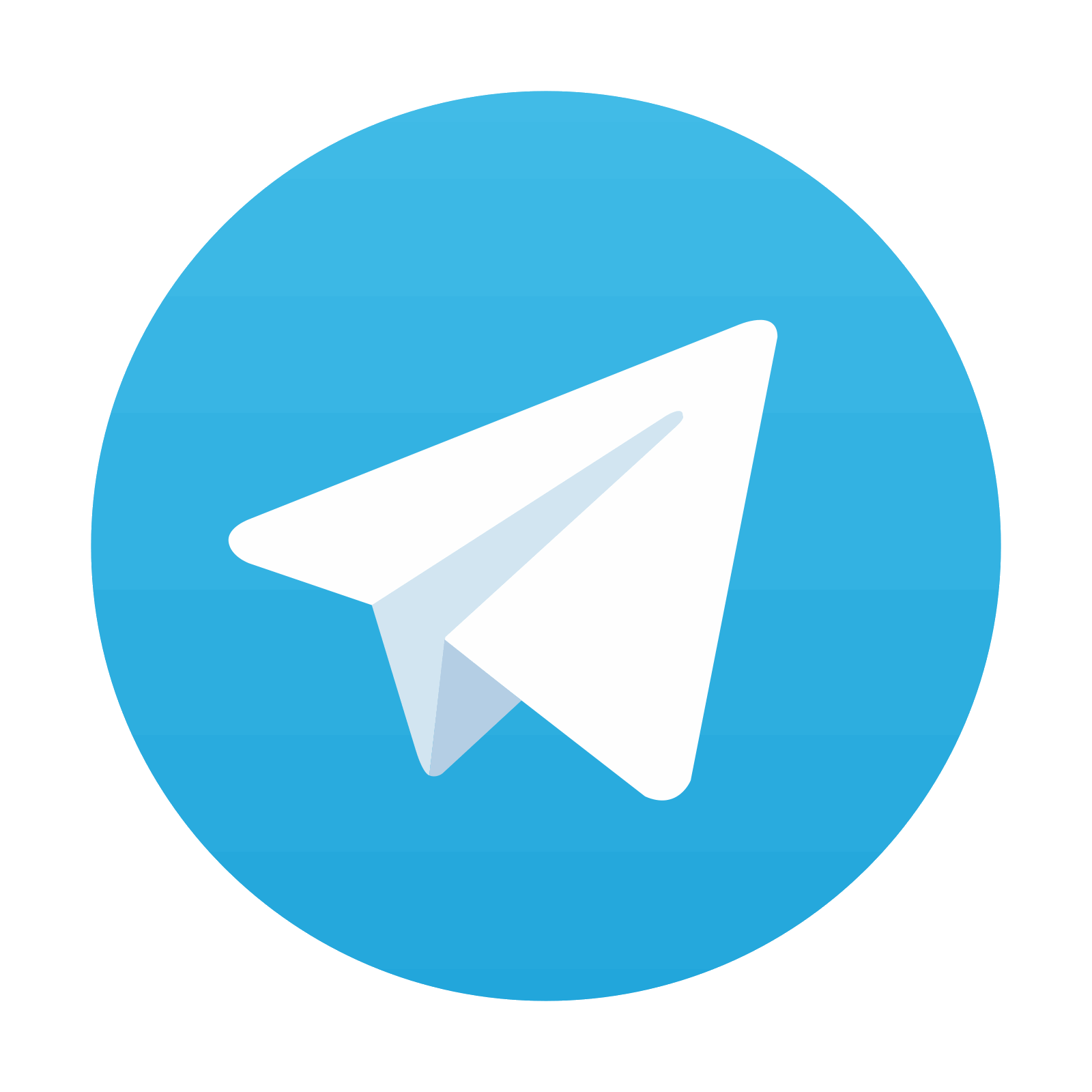
Stay updated, free articles. Join our Telegram channel
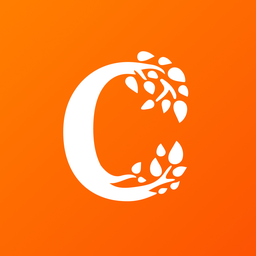
Full access? Get Clinical Tree
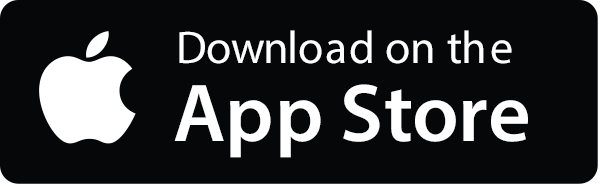
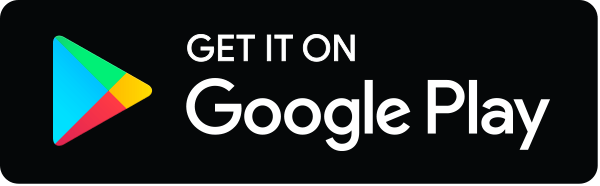
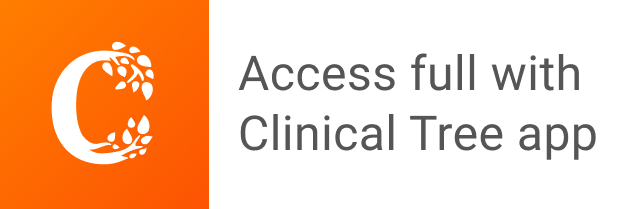