Tumor type
Cytogenetic finding
Molecular trace
Reference
Ewing sarcoma
t(11;22)(q24;q12)
EWSR1-FLI1
Delattre et al. [100]
t(21;22)(q22;q12)
EWSR1-ERG
Sorensen et al. [240]
t(19;der(ins.inv(21;22)))
EWSR1-ERG
Maire et al. [241]
t(16;21) (p11;q22)
FUS-ERGa
Shing et al. [242]
t(7;22;)(p22;q12)
EWSR1-ETV1
Jeon et al. [243]
t(17;22)(q12;q12)
EWSR1-ETV4
Kaneko et al. [244]
t(2;22)(q33;q12)
EWSR1-FEV
Peter et al. [245]
t(6;22)(p21;q12)
EWSR1-POU5F1
Yamaguchi et al. [246]
t(1;22) (q36.1;q12)
EWSR1-PATZ1
Mastrangelo et al. [247]
t(2;22)(q31;q12)
EWSR1-SP3
Wang et al. [248]
t(20;22)(q13;q12)
EWSR1-NFATc2
Szukhai et al. [249]
t(2;16)(q35;p11)
FUS-FEV
Ng et al. [250]
t(15;19)(q14;p13.1)
BRD4-NUTa
Mertens et al. [251]
t(4;19)(q35;q13)
CIC-DUX4
Kawamura-Saito et al. [252]
Desmoplastic small round cell tumor
t(11;22)(p13;q12)
EWSR1-WT1
Ladanyi et al. [253]
Round cell, myxoid liposarcoma
t(12;16)(q13;p11)
FUS-DDIT3
Crozat et al. [254]
t(12;22)(q13;q12)
EWSR1-DDIT3
Panagopoulus et al. [255]
Epithelioid pleomorphic liposarcoma
t(12;16)(q13;p11)
FUS-DDIT3 (CHOP)
Cecco et al. [256]
Extraskeletal myxoid chondrosarcoma
t(9;22)(q22;q12)
EWSR1-CHN
Clark et al. [257]
t(9;17)(q22;q11)
hTAFII68-CHN
Attwooll et al. [258]
t(9;15) (q22;q21)
TCF12-CHN
Sjogren et al. [259]
t(3;9)(q12;q22)
TFG-CHN
Hisaoka et al. [260]
Clear cell sarcoma
t(12;22)(q13;q12)
t(2;22)(q33;q12)
EWS-ATF1
EWS-CREB1
Zucman et al. [85]
Antonescu et al. [261]
Angiomatoid fibrous histiocytoma
t(12;22)(q13;q12)
t(2;22)(q33;q12)
EWS-ATF1
EWS-CREB1
Hallor et al. [86]
Rossi et al. [262]
Synovial sarcoma
t(X;18)(p11.23;q11)
SS18-SSX1
Crew et al. [263]
t(X;18)(p11.21;q11)
SS18-SSX2
Clark et al. [264]
t(X;18)(p11;q11)
SS18-SSX4
Skytting et al. [265]
t(X;20)(p11;q13.3)
SS18L1-SSX1
Storlazzi et al. [266]
Alveolar rhabdomyosarcoma
t(2;13)(q35;q14)
PAX3-FOXO1
Galili et al. [267]
t(1;13)(p36;q14)
PAX7-FOXO1
Davis et al. [268]
t(2;2)(q35;p23)
PAX3-NCOA1
Wachtel et al. [194]
t(2;8)(q35;q13)
PAX3-NCOA2
Sumegi et al. [269]
Embryonal rhabdomyosarcoma
t(2;8) (q35; q13)
PAX3-NCOA2
t(6;8) (p21;q11.2)
t(8;11)
SRF-NCOA2
TEAD1-NCOA2
Mosquera et al. [272]
Dermatofibrosarcoma protuberans
t(17;22) (q22;q13)
COL1A1-PDGFB
Simon et al. [273]
Infantile fibrosarcoma/cellular mesoblastic nephroma
t(12;15)(p13;q25)
ETV6-NTRK3a
Knezevich et al. [96]
Alveolar soft part sarcoma
t(X;17)(p11;q25)
TFE3-ASPL a
Ladanyi et al. [274]
Low grade endometrial stromal sarcomab
t(7;17)(p15;q21)
JAZF1-SUZ12
Koontz et al. [275]
Inflammatory myofibroblastic tumor
t(1;2)(q25;p23)
TPM3-ALK a
Lawrence et al. [276]
t(2;19)(p23;p13)
TMP4-ALK
Lawrence et al. [276]
t(2;17)(p23;q23)
CLTC2-ALK
Bridge et al. [277]
t(2;2)(p23;q13)
RANBP2-ALK
Ma et al. [278]
Low-grade fibromyxoid sarcoma
t(7;16)(q33p11)
t(11;16)(p11;p11)
FUS-CREB3L2
FUS-CREB3L1
Storlazzi et al. [279]
Mertens et al. [280]
In the case of the desmoplastic small round cell tumor (SRCDT ), the EWS gene is bound to the WT1 gene. At first, WT1 was known to be an altered tumor suppressor gene in Wilms tumor (nephroblastoma); in fact, EWS-WT1 is the first example of a constant rearrangement of a tumor suppressor gene. The chimeric transcript EWS-WT1 has been found in 97 % of cases that were studied. This is very helpful for diagnosis [82]; it also suggests the chimeric protein is important for the tumor development. It is, as in many other sarcomas, an aberrant transcription factor that regulates the expression of genes that partially coincide with usual WT1 targets. One of them is PDGFA, a fibroblastic growth factor, which is probably involved in the characteristic fibrosis of this neoplasia [83]. BAIAP3 is another factor that regulates the exocytosis process, and thus the secretion of growing factors [84].
EWS binds to ATF1 in the clear cell sarcoma (soft tissue malignant melanoma) [85] and in the angiomatoid fibrous histiocytoma [86]. EWS binds to a DNA-binding domain of a transcription factor, as in the Ewing tumor. Contrary to ATF1, EWS-ATF1 fusion works as a transcriptional activator, probably altering the regulation of genes usually controlled by ATF1. The chimeric RNA is detected both in fragments of frozen tumor and formalin-fixed paraffin-embedded material [87].
EWS-CHN fusion , generated from a t(9;22), is observed in the extraskeletal myxoid chondrosarcoma, curiously, not from myxoid chondrosarcoma of osseous origin [88]. CHN codifies a nuclear receptor having a binding domain to DNA. The fusion protein has an EWS amino-terminal domain bound to the whole CHN reading frame; this yields a nuclear receptor more active than the native one. This receptor is involved in the control of cellular proliferation by modulating the response to diverse growth factors. There are some infrequent variants of this fusion. Genomics studies have shown that this tumor has a high expression on PPARG. There are some specific inhibitors against this molecule, which could become a therapeutic target [89].
In addition, a gene analogous to EWS, FUS, is involved with CHOP (DDIT3) in gene fusion in 90 % of cases comprising round cell/myxoid liposarcoma (FUS-CHOP) [90]. CHOP (DDIT3) is a transcription factor. In TLS-CHOP (FUS-DDIT3), TLS binding domain to RNA is replaced by CHOP binding domain to DNA. The relation between myxoid liposarcoma and round cells is confirmed by detecting FUS-CHOP fusions in tumors made up, as a whole or in part, of round cells [90]. Approximately 5 % of cases show EWS-CHOP fusions, wherein EWS has an analogous role to FUS. Thus, proteins having the ability to bind to RNA, FUS, and EWS seem to be functionally similar, while the component providing the binding domain to DNA, CHOP, is specific in this neoplasia.
34.4.1 Alveolar Rhabdomyosarcoma
Alveolar rhabdomyosarcoma is associated to a characteristic translocation, t(2;13), and another less common one, t(1;13). This is the result of PAX3 and PAX7 gene fusion, respectively, with forkhead in RMS (FKHR) localized in 13q14 [91]. PAX genes are transcription factors regulated during the embryo development; they are essential for the genesis of some organs. PAX3 and PAX7, in particular, are expressed in the neural tube and are fundamental for its correct formation, and for the migration of myoblasts to upper and lower extremities. PAX3 could suppress myoblast differentiation; this could lead to the undifferentiated phenotype of this tumor. Some amplification has been detected concerning gene fusions in some tumors with PAX7-FKHR fusions; this suggests that translocation and amplification may be sequential mechanisms in the oncogenesis of this neoplasia. As for PAX3-FKHR fusion, an overexpression of transcriptional origin was detected on PAX3 non-associated to gene amplification [92]. These differences concerning PAX3 and PAX7 overexpression mechanisms are analogous to those observed at clinic level. PAX7-FKHR tumors tend to turn up in younger patients and are associated to a lower rate of metastasis and a better survival than those with PAX3-FKHR, even though they have a similar morphology [93].
34.4.2 Synovial Sarcoma
Synovial sarcoma is characterized due to t(X;18); it generates a fusion between SS18 (SYT) gene on chromosome 18 with one SSX gene: SSX1 and SSX2. They are located in two subregions of chromosome Xp11 (23 and 21, respectively); there are, though, rarer fusions. This fusion codifies an abnormal nuclear transcription factor which alters chromatin remodeling; this may induce some changes in the gene expression patterns. Transcripts are detected in almost all synovial sarcomas through RT-CPR. The synovial sarcoma is a clear example of correlation tumor phenotype and transcript type. Biphasic synovial sarcoma is associated with SYT-SSX1 fusions, which are present both in epithelial and fusocellular elements, while monophasic synovial sarcoma is usually present with SYT-SSX2. In addition, patients at clinic level with SYT-SSX2 show a relatively low risk of relapses, while tumors with SYT-SSX1 variant show a high proliferative rate and a worse prognosis [52, 94]. However, the prognostic value of these fusions is still under discussion [95].
Translocation t(17;22) in dermatofibrosarcoma protuberans and giant cell fibroblastoma generates a fusion involving COL1A1, a collagen gene and PDGFB, a protein-modifying gene with growing factor function. This fusion sets PDGFB under the control of COL1A1 promoter, thus removing all elements repressing PDGFB transcription. In the case of congenital fibrosarcoma, t(12;15) translocation unites ETV6 (TEL) gene with neurotrophin 3 receptor (NTRK3) [96]. Curiously, this fusion is also specifically seen in mesoblastic nephroma, acute myeloblastic leukemia, and secretory carcinoma of the breast, a rare variant of the infiltrating ductal carcinoma of the breast [97]. This phenomenon suggests that the same gene fusion may cause different neoplasms, and depends on the characteristics of the cell where this occurs. These two examples represent the exception confirming the general rule that gene fusions generate new ETV6-NTRK3 transcription factors in a chimeric kinase tyrosine, which may contribute to oncogenesis by deregulation of transduction pathways of signals generated by NTRK3, while PDGFB-COL1A1 probably acts as an autocrine growing factor.
34.5 Chimeric Proteins Resulting from Translocations: Structure and Function
34.5.1 Chimeric Protein Structure : ES Oncoprotein as an Example
Sarcomas are paradoxical examples of how a disease with a relatively low prevalence can bear prominent biological significance because of their molecular mechanisms of generation and progression. Fusion genes resulting from chromosomal translocations can be transcribed and translated into chimerical proteins. Let us first take Ewing sarcoma oncoproteins as an example [3, 98].
The ES family of tumors (ES) comprises morphologically heterogeneous tumors that are characterized by nonrandom chromosomal translocations involving the EWS gene and one of the several members of the ETS family of transcription factors [99]. The translocation t(11;22)(q24;q12) is the most common one, associated to 90 % of cases, and this high specificity suggests that the product of this rearrangement is involved in the formation of these malignancies. This translocation leads to in-frame fusion of EWS at 22q12 to FLI1 at 11q24 and the formation of EWS-FLI1 on der[22] comprising the 5’ end of EWS and the 3’ end of FLI1 [100]. The fusion gene encodes an oncoprotein consisting of the N-terminal domain of EWS and the C-terminal DNA-binding domain of transcription factors such as ETS family, activating transcription factor-1, Wilms’ tumor 1, and nuclear orphan receptors [101].
EWS protein is predicted to be an RNA-binding protein containing the transcriptional activation domain(s) in the N-terminal domain and the RNA recognition motif and three arginine-glycine-glycine repeats (RGG boxes 1–3) in its C-terminal domain [102, 103]. The transcriptional potency of the N-terminal domain of EWS observed in its various tumorigenic fusion proteins suggests that EWS may function as a transcription factor. In the EWS-FLI1 fusion protein, the RNA-binding motif containing the C-terminal half of EWS is replaced by the DNA-binding domain (DBD) of the FLI1 protein. EWS belongs to a subgroup of RNA-binding proteins called the TET family, which also includes liposarcoma/fusion protein (TLS/FUS) and human TATA-binding protein-associated factor (hTAFII68) [90, 104]. They all contain a distinctive conserved 87-amino-acid RNA recognition motif/ribonucleoprotein consensus sequence (RRM/RNP-CS) motif commonly found in most RNA-binding proteins and possess a number of R-G-G (arginine-glycine-glycine) repeats that also seem to facilitate binding to RNA. A zinc finger domain with four cysteines is present in their C-terminal domain. In addition, mammalian TET family members share glutamine-rich N-terminal domains consisting of a series of degenerate repeats. In Fig. 34.1, we present a schematic view of EWS protein domains.


Fig. 34.1
Schematic view of the structural features of a prototypic sarcoma chimeric protein, and the native proteins it arises from. EWS-FLI1 type 1 protein is associated with ES. Primary and secondary structures, as well as functional regions of native EWS, FLI1, their breakpoints, and the resulting fusion protein are depicted. EWS N-terminal domain contain amino acid residues 1–264, referred to as the EWS-activation domain (EAD). It contains 31 degenerate hexapeptide repeats (DHR) with the consensus sequence SYGQQS and 7 other Tyr residues. The C-terminal domain begins with the IQ domain, a region susceptible of binding calmodulin and being phosphorylated. Next, it has a distinctive conserved 87-amino-acid RNA recognition motif/ ribonucleoprotein consensus sequence motif (RRM/RNP domain) commonly found in most RNA-binding proteins, and possess a number of RGG (arginine-glycine-glycine) repeats that also seem to facilitate binding to RNA. A zinc finger domain with four cysteines (C2-C2 Zn finger-like) is also present close to the C-terminal end. FLI1 is a transcription factor, with a naturally occurring transactivating region in the N-terminal end. It encloses a highly conserved, sequence-specific, ETS-type DNA-binding domain. FLI1 also has contains a C-terminal end transactivating domain (CTA). The chromosomal t(11;22)(q24;q22) translocation in ES results in the fusion of the N-terminal domain of EWS and the DNA-binding domain of FLI1, generating the oncoprotein EWS-FLI1. The transactivating domain of EWS is retained, while the RNA-binding domain is lost, replaced by the sequence-specific DNA-binding domain of the FLI1 protein. The type 1 chimeric protein is represented entirely schematically. Types 2 or 3 can be deduced from type 1 by the insertion of 84 or 22 additional amino acids from EWS or FLI1, respectively.
Three key features indicate that the EWS protein is encoded by a housekeeping gene; it is expressed ubiquitously, its expression is stable throughout the cell cycle, and its mRNA has a long half-life [90]. Although more remains to be known about the role of native EWS, it is a nuclear protein that appears to be recruited to promoter regions where it associates with other factors to act as a promoter-specific transactivator [105, 106]. It also functions as a transcriptional coactivator in a cell type-specific and promoter-specific manner. It requires CBP/p300 for hepatocyte nuclear factor 4 (HNF4)-mediated transcriptional activation [107, 108]. Although EWS fusion proteins function as sequence-specific transcription factors, the role of native EWS protein and the regulatory mechanism controlling the coactivator function of EWS are largely unknown.
FLI1 is a member of the ETS family of transcription factors which activate specific target genes by binding to their cognate DNA sequences through their DNA-binding regions, usually located at their carboxyl termini [109, 110]. The replacement of the native transcription activation domain(s) of FLI1 by the N-terminal region of EWS converts the nontransforming activator, FLI1, into a transforming protein with new transcriptional activation potential. The ETS family of transcription factors is defined by a conserved ETS domain that recognizes a core DNA motif of GGAA/T [111]. This family of approximately 30 genes including FLI1, ERG, ETV1, E1AF, FEV, and ZSG controls a variety of cellular functions in cooperation with other transcription factors and cofactors. Target genes include oncogenes, tumor suppressor genes, and genes related to apoptosis, differentiation, angiogenesis, and invasion [111, 112]. Recently, Riggi et al. reported that EWS-FLI1 acts on chromatin remodeling playing a main role in Ewing sarcoma biology [71].
34.5.2 Protein–Protein Interactions Studies Provide Structural and Functional Insights into the Mechanisms of Action of Native Proteins in Sarcomas
We can next take a look inside the archetypical case of the ES chimera protein. In the EWS-FLI1 fusion protein, both the N-terminal domain of EWS and the DBD of FLI1 are necessary for the transforming activity of Ewing cells [101, 102]. To assess the contribution of the N-terminal domain of the EWS protein to the formation of human solid tumors, it is important to understand the normal function(s) of EWS. Although much is known about the oncogenic functions of chimeric EWS fusion proteins that result from chromosomal translocations, the cellular role of the normal EWS protein is not well characterized.
Bertolotti et al. investigated the putative role of EWS in RNA polymerase II (Pol II) transcription by comparing its activity with that of its structural homolog, hTAFII68 [105]. The structural homology between EWS and the transcription factor hTAFII68 (70 % similarity among the full-length proteins) strongly suggested that there may be a functional homology between these proteins. The authors demonstrated that a portion of EWS is able to associate with the basal transcription factor TFIID, which is composed of the TATA-binding protein (TBP) and TBP-associated factors (TAFIIs). In vitro binding studies revealed that both EWS and hTAFII68 interact with the same TFIID subunits, suggesting that the presence of EWS and that of hTAFII68 in the same TFIID complex may be mutually exclusive. Moreover, EWS is not exclusively associated with TFIID but, similarly to hTAFII68, is also associated with the Pol II complex. The subunits of Pol II that interact with EWS and hTAFII68 were identified, confirming the association with the polymerase. On the other hand, using Ewing cell nuclear extracts, they studied the association of EWS and the oncogenic fusion protein, EWS-FLI1, with different multiprotein complexes. These experiments suggest that EWS and EWS-FLI1 behave differently since EWS-FLI1 cannot stably associate with either TFIID or Pol II in Ewing cell nuclear extracts. These observations suggested that EWS and EWS-FLI1 may play different roles in Pol II transcription.
Employing yeast two-hybrid cloning to study protein–protein interactions, Petermann et al. isolated the seventh largest subunit of human RNA polymerase II (hsRPB7) as a protein that specifically interacts with the amino terminus of EWS [113]. This association was confirmed in nuclear extracts, where hsRPB7 was found to co-purify with EWS-FLI1 but not with FLI1. Overexpression of recombinant hsRPB7 specifically increased gene activation by EWS-chimeric transcription factors, whereas replacement of the EWS portion by hsRPB7 in the oncogenic fusion protein restored the transactivating potential of the chimera. Those results suggested that fusion to FLI1 causes as structural change of the amino-terminal EWS domain, making it accessible for interaction with hsRPB7. Presumably, the physical interaction of the amino terminus of EWS with hsRPB7 contributes to the transactivation function of EWS-FLI1 and, since hsRPB7 has characteristics of a regulatory subunit of Pol II, may influence promoter selectivity.
Most chimeric proteins in many sarcoma types function as aberrant transcription factors. One step further in the characterization of the RNA-binding capabilities of such proteins was the revelation by proteomics techniques of the association of EWS protein to the IRES of central proteins in some pathogenic processes. This was the case in hepatitis C virus-infected cells [114]. The authors used shotgun peptide sequencing to identify proteins in quadruplicate protein affinity extracts of lysed cells, obtained using a biotinylated IRES. EWS protein contains an RRM RNA recognition motif and a zinc finger RNA-binding region, through which interaction occurs. From a mechanistic point of view, protein binding to the IRES is an indication of the importance of that protein, both for its functional value as well as a potential drug target. In particular, EWS may shuttle from the nucleus to the cell surface [115] and function as a transcriptional cofactor with CBP/p300 [106]. This interpretation is particularly interesting in view of the proposed role of the nucleolus as a gateway for viral infection and site of replication for many viruses. Although to our knowledge this point has not been studied in sarcomas, the evidences gathered to date indicate that it might be interesting to investigate the potential role of EWS as an IRES-binding protein.
Despite the numerous reports aiming at the function of TET proteins as a partner of fusion proteins in different sarcoma translocation types, little evidence has been collected about the cellular function of the normal proteins, let alone from proteomic approaches. Anumanthan et al. reported the interaction between a WD domain-containing protein, serine-threonine kinase receptor-associated protein (STRAP) and EWS, using matrix-assisted laser desorption/ionization, time-of-flight and tandem mass spectrometry (MALDI-ToF MS/MS) [116]. STRAP interacts with EWS in the nucleus and induces inhibition of EWS transactivation function. Their research found that normal EWS protein is upregulated in human cancers, suggesting a cooperative role of these two proteins in tumorigenesis. Results suggested that STRAP inhibits the transactivation function of EWS by displacing p300 from the functional transcriptional complex. In consequence, this study provided a novel TGF-ß-independent function of STRAP and described a mechanism by which STRAP regulates the physiologic function of oncogenic EWS protein in the nucleus.
Gaining insight into the role of TET proteins in RNA metabolism, Guipaud et al. characterized one of the precise cellular functions of these multifunctional proteins, DNA pairing [117]. Conservation across species suggests that TET proteins have important physiological roles. TET proteins are thought to be involved in RNA transcription and/or processing [118, 119]. Besides these functions, several lines of evidence suggested a new role for TLS/FUS in DNA transaction and in DNA double-stranded break rejoining [120, 121]. Particularly, they addressed the question whether EWS displays pairing on membrane (POM) activities. Since EWS share similar features with TLS/FUS (structure, partners, and affinities), they raised the question whether it also exhibits POM activities. Accordingly, they applied the assay to 2-DE coupled to MS analysis for a global screening of catalysis of homologous DNA POM activities. This test allowed them to identify EWS, in addition to hTAF(II)68, TLS/FUS, but no other proteins, indicating a feature specific to a protein family whose members share extensive structural similarities.
This common activity suggests a role for TET proteins in genome plasticity control. The results suggest for TET proteins a role in DNA metabolism in addition to their role in pre-mRNA splicing and transcription regulation. Proteins that function at the interface between transcription and RNA metabolism affect genetic stability by regulating transcription-associated recombination. Significantly, the homologous DNA pairing activity of TLS/FUS is supported by the phenotypes of TLS/FUS-deficient mice [122, 123]. Based on these findings and on their DNA pairing activities, it is thus tempting to speculate on a role for POM proteins in genome plasticity control. This common enzymatic feature could also be of importance in understanding tumor development involving these proteins.
34.5.3 The Role of Chimeric Proteins in Sarcoma Molecular Mechanisms
Much of the understanding about the function of sarcoma genes is derived from studies of oncogenic fusions that arise from gene rearrangement after chromosomal translocation. And, obviously, even more research has been devoted to understand the mechanisms of action of the chimera proteins themselves, as necessary (but not sufficient?) effector of the tumorigenic cascade of events that ultimately cause the onset of tumoral alterations. However, although extensive work has been performed on cellular, molecular, and genomic lines of attack, little knowledge has been reported so far related to proteomics approaches (Fig. 34.2).


Fig. 34.2
Flow-chart diagram illustrating the experimental steps taken in a proteomics approach to characterize and identify the protein(s) present in a clinical sample. (1) Samples are collected following the recommendations from the donor tumor bank, (2) protein extracts are separated from the cellular debris, and (3) prepared for the proteomic analysis through two-dimensional electrophoresis (2-D PAGE). After isoelectric focusing in a pH gradient the electrophoretic separation is completed in a second polyacrylamide gel dimension. Proteins can be then visualized after staining (chemically or radioactively). A close-up 2-D PAGE image of an assay showing protein expression differences in an experimental ES cell line TC71 versus a control illustrates the proceeding of the comparison, that eventually renders information that can be linked with the pathogenesis of the studied disease; (4) proteins are digested by proteolytic enzymes to smaller peptides and their precise identity is achieved using mass spectrometry (generally performed in two ways); (5) either, precise molecular weights are measured using MALDI-ToF instruments, and the results compared with protein sequences from available databases. Or, (6) tandem mass spectrometry gives information about protein ) sequence that is subjected to the search in databases; (7) finally, the identification of the protein(s) in the initial sample can be solved with almost full certainty in 90 % of the cases.
An alternative proteomic approach has been recently developed in our laboratory, aiming at identifying differences in protein expression and posttranslational modifications attributable to the loss of EWS-ETS protein activity: an interference shRNAi model for EWS-FLI1 was constructed in the TC71 ES cell line [124]. Protein expression levels were analyzed in cell extracts of the shRNAi clone model to gain insights into the differential changes associated with the interference of the EWS-FLI1 transcript [125]. Two-dimensional PAGE analyses were performed to visualize differences in a plethora of different conditions. Most of the changes associated with the shRNAi clone were presented as an overexpression of their protein levels (Fig. 34.2). In contrast, about one third of the proteins showed a clear reduction of their expression amounts. Further examination of those results by a MALDI-ToF MS analysis enabled the identification of significant protein changes. Interestingly, many of the identified proteins with the highest scores were molecules directly involved in RNA and DNA processing and functioning. Given the fact that the protein chimera EWS-FLI1 acts as an aberrant transcriptional factor, this evidences suggest that the interference model is altering several protein regulations, in pathways directly and indirectly related to EWS-FLI1, which in consequence brings about changes in expression patterns for molecules involved in nucleic acid activities.
Proteomic expression pattern showed differences for nuclear proteins. For instance, a component of the RNA polymerase II holoenzyme complex that is involved in DNA recombination, DNA repair, and protein folding, in agreement with previously commented reports about the involvement of TET-ETS fusion proteins in direct regulation of RNA polymerase II. Similarly, we have observed alterations in a structural constituent of the ribosome, which binds RNA during translation. An aminoacyl-tRNA synthetase was also selected; through the regulation of Akt, and therefore, modulation of the HSP90 stress-responsive activity, the biological function of this tRNA synthetase may play a role in the response of ES cell machinery to stress conditions [126]. Another factor engaged in protein translation is a component of the polyribosome, whose molecular function is the binding of mRNA. This protein has been reported to participate in Wnt signaling, which, in turn, has been proven to play an important role in the development of some types of sarcoma [41, 127]. The list includes more proteins involved in DNA/RNA metabolisms, as well as some others implicated in RNA translation and protein synthesis.
Besides that aspect of chimeric protein regulation, there is another group of proteins altered in sarcoma cells, whose functions are associated to cell cycle and cell division activities. Another protein involved in cell cycle is a serine/threonine phosphatase, essential for cell division. From previous analysis (immunohistochemistry, flow cytometry), it has been suggested that this phosphatase is involved in the accelerated growth of malignant tumor cells in sarcoma.
Finally, we identified in the shRNAi clone a group of targets related to cellular protein metabolic processes. In particular, several of them are molecules engaged in protein binding, folding, or rearranging of polypeptide bonds and structures in specific protein species. It seems that the chimera protein inhibits, in some way, several effectors that have to do with the assembling of tertiary structures of certain proteins. The overexpression of these or similar proteins in the RNA-interfered model may provide some clues about the molecular changes accompanying the redifferentiation program of cells after inhibition of the fusion gene induction. Alternatively, other candidates in this group are connected with proteolytic degradation processes. This is the case of a proteasome subunit. It has been recently pointed that EWS-FLI1 plays an important role in the prevention of senescence, leading to the unlimited growth and oncogenesis of ES cells through a decrease in the stability of p27 protein, due to increased action of Skp2-mediated 26S proteasomal degradation [128].
These results partly correlate with data from a gene expression microarray experiment, where transcript levels from the shRNAi clone and controls were registered by qRT-PCR. Further and deeper studies are currently being accomplished in our group to characterize the effect of another variables, such as the stability of the RNA inhibition with time progression, posttranslational modifications (e.g., phosphorylation, acetylation, etc.), and to quantitate the magnitude of these changes, both at the protein level, and at the translational level.
34.5.4 Functional Studies of Proteins Determining the Tumorigenicity of Sarcomas : An Overview of Protein Targets Identified
The depiction of cellular functionality that explains and corroborates the mechanisms by which cancer originates and eventually propagates has traditionally been entrusted to genomic and post-genomic strategies. For example, microarray analyses have identified differentially expressed genes from many classes that might contribute to the different steps of cancer generation and progression [129, 130], but these expression changes only suggest functional importance. RNA-mediated interference (RNAi) screens in mammalian cells can functionally assess the genome in high throughput, and these screens for cancer phenotypes have identified new gene targets [131]. However, such screens are limited because RNAi knockdown occurs over many hours, and because proteins that turn over slowly cannot be efficiently depleted. The alternative would be to develop a high-throughput approach that directly addresses protein function and is temporally restricted. Such an approach could identify new and different targets for tumor invasion or other disease-relevant processes.
Jay and colleagues exploited a previously developed technology for functional proteomics to identify and validate proteins required for cancer invasion in fibrosarcoma cells [132]. This technique, known as fluorophore-assisted light inactivation (FALI) , affords the possibility of targeting a particular surface protein for destruction using the specificity of an antibody [133]. The authors revealed that the molecular chaperone heat shock protein 90 (HSP90) plays a crucial role in the invasion of fibrosarcoma cells. HSP90 was shown to promote maturation of the extracellular metalloprotease MMP2, and therefore, was identified as an important extracellular mediator of invasion.
Making also use of the FALI technology and libraries of monoclonal antibodies and phage-expressed single-chain antibodies, the same group identified CD155/PVR as a key protein in cell motility during cell invasion and migration in fibrosarcoma [134]. CD155 was found to be highly expressed in multiple cancer cell lines and primary tumors including glioblastoma. They showed that CD155 is recruited to the leading edge of migrating cells where it co-localizes with actin and alpha v-integrin, known mediators of motility and adhesion.
34.6 Cellular Signaling Pathway Categories
The cell signaling pathways (Fig. 34.3), cell surface adhesion molecules, receptor tyrosine kinases, growth factors, transcription factors, and early developmental genes expressed in these tumors identify potential candidates for therapeutic intervention and diagnostic development.


Fig. 34.3
Main cell signaling pathways described in sarcomas . This figure shows the importance of certain specific pathways in sarcomas. The Wnt-frizzled signaling pathway has been found in both, ES and synovial sarcoma. The MEK/MAPK and PI3-K signaling pathways contribute to the malignant behaviour of ES cells and gastrointestinal stromal tumor. The AKT-mTOR pathway plays a critical role in the development of leiomyosarcomas. In dashed lines are shown possible pathways interactions.
A tight clustering has been observed analyzing the gene expression data from 181 tumor types, representing 16 classes of human bone and soft tissue sarcomas on a 12,601-feature cDNA microarray [59]. On one hand, the dendrogram is populated primarily by the more poorly differentiated or dedifferentiated tumors and the typically adult sarcomas with the major branches being formed by the malignant fibrous histiocytoma tumors, leiomyosarcoma, dermatofibrosarcoma, hemangiopericytoma, and liposarcoma. On the other hand, the tightly clustered groups are assigned to the pediatric sarcomas, Ewing sarcoma, synovial sarcoma, osteosarcoma, and RMS. This could denote the existence of common signaling pathways for each branch.
34.6.1 Tyrosine Kinases
ES proliferation and survival is also determined by autocrine and paracrine activation of growth factor receptors and their ligands as insulin-like growth factor 1 [135]. EWS-FLI1 shRNA interference affects IGF-1/IGF-1R survival pathway and its downstream targets (Fig. 34.4) [136]. shRNAi clone showed a marked decrease in IGF-1 transcript and protein levels, both early and late stages (Fig. 34.4a, b), but there were no significant changes in IGF-1R protein level (Fig. 34.4b). shRNAi clone was more sensitive to the action of IGF-1R signaling pathway inhibitors (Fig. 34.4c). A higher apoptotic index was detected, as analyzed by FACS for apoptosis using annexin V (Fig. 34.4d) as well as the reduction of proteins involved in the activation of IGF-1R signaling pathway after treatment with AEW571 combined with inhibitors LY294002 and PD98059 (Fig. 34.4e).


Fig. 34.4
EWS-FLI1 shRNA interference affects IGF1/IGF1R survival pathway and its downstream targets. (a) IGF1 mRNA expression level in TC71wt, shRNAi and mock (early and late stages) determined by qRT-PCR. (b) IGF1 and IGF1R protein levels as assessed by Western Blot. (c) IC50 of proliferation after AEW571, LY294002 or PD98059 treatment at 72 h of incubation measured by MTT assay. shRNAi clone is more sensible to the action of drug and inhibitors. (d) Apoptotic index after AEW571, LY294002 or PD98059 treatment at 72 h of incubation cells were seeded on 24 well plates, 72 h after cells were analyzed by FACS for apoptosis using an annexin V detection kit assay. The means ± standard deviations (error bars) of 4 independent experiments are shown. (e) Effects of AEW571 combined with inhibitors LY294002 and PD98059 on the activation of IGF1R signaling pathway. All conditions were treated with AEW571 during 15 min and with the inhibitors for 2 h, before IGF1 stimulation (50 ng/ml) during 15 min (serum-free conditions). Data obtained from Herrero et al. 2010. Permission applied [136].
Benini et al. analyzed the contribution of the 2 major pathways of the intracellular IGF-1R signaling cascade to the overall effects elicited by IGF-1 in Ewing sarcoma [137] Both the mitogen-activated protein kinase (MAPK) and phosphatidylinositol-3-kinase (PI3-K) signaling pathways appeared to be constitutively activated in Ewing sarcoma, likely due to the presence of the IGF-1R-mediated autocrine loop. Exogenous IGF-1 completely reverted LY294002-induced growth inhibition by abrogating anti-proliferative and pro-apoptotic effects of the PI3-K inhibitor. By contrast, IGF-1 could not rescue cells from growth inhibition induced by PD98059. MEK/MAPK blockade also significantly reduced the migratory ability of ES cells, both in basal and IGF-1-induced conditions, and increased chemosensitivity to doxorubicin, a leader drug in the treatment of ES patients.
Hernando et al. analyzed the PI3K-AKT signaling cascade in a cohort of sarcomas and found a new and critical role for the AKT-mTOR pathway in smooth muscle transformation and leiomyosarcoma genesis [35]. PTEN is a dual–function lipid and protein phosphatase originally identified as a protein encoded by a tumor suppressor gene, found to be frequently mutated in sporadic cancers and hereditary disorders. The main substrate of PTEN is phosphatidylinositol-3,4,5-triphosphate (PIP3), a second-messenger molecule generated by the action of PI3Ks. PIP3 activates the serine-threonine kinase AKT, involved in cell survival, proliferation, and growth. Other alterations, such as IGF-1 or IGF-1R overexpression, also frequently observed in leiomyosarcoma [138], could ultimately account for the observed upregulation of this pathway.
The putative aberrant signaling provided by c-kit overexpression may be dispensable for Ewing sarcoma development and is unlikely to constitute a critical therapeutic target [139, 140] Baird et al. found highly expressed tyrosine kinases or receptor tyrosine kinases associating with half of the tumor groups [59] Along with known highly expressed kinases, such as KIT in gastrointestinal stromal tumor and PDGFRB in dermatofibrosarcoma protuberans, they additionally found JAK1 in Ewing sarcoma, FLT1 in hemangiopericytoma, EGFR and PDGFRA in synovial sarcoma, and several FGFR in osteosarcoma.
34.6.2 WNT Signaling Pathway
The WNT signaling pathway (Fig. 34.3), involved in the development of brain and the peripheral nervous system, has been found to play a critical role in the formation of several cancers [141], including synovial sarcoma [142–144], Ewing sarcoma [145], and malignant fibrous histiocytoma [41]. Wnts activate a canonical pathway that is characterized by accumulation of β-catenin in the cytoplasm. In the absence of Wnt signaling, cytoplasmic b-catenin forms a complex with Axin, adenomatous polyposis coli (APC) gene product, glycogen synthase kinase-3b (GSK-3b), and casein kinase I-a (CKIa). Phosphorylation of β-catenin by CKIa and GSK-3b results in its ubiquitination and rapid degradation [146–148]. Wnt binding to Fz and LRP5 or LRP6 disrupts this degradation machinery by a process that involves Disheveled (Dvl)-dependent recruitment of Axin to the plasma membrane [147]. As a result, unphosphorylated β-catenin accumulates in the cytoplasm and nucleus, forming a complex with members of the TCF/LEF-1 family of transcription factors that activates transcription of target genes such as c-myc and cyclin-D1 [149–151]. Mutations of Axin, APC, and β-catenin have been characterized in numerous human malignancies including colon cancer, malignant melanoma, hepatocellular carcinoma, endometrial carcinoma, ovarian carcinoma, and prostate cancer [152, 153]. A common outcome of these mutations is the accumulation of free β-catenin, mimicking constitutive Wnt activation. Saito et al. showed that APC mutations also occur in sarcomas, especially in synovial sarcoma, and the possible inactivation of the APC gene by missense mutations was thought to contribute to the accumulation of b-catenin in synovial sarcoma [154]. Baird et al. also found e vidence implicating this pathway in synovial sarcoma [59]. WNT5A expression was pronounced, as was the expression of the WNT signaling targets FZD1, CDH4, EN2, TLE4, and TLE1. TLE1, WNT5A, FZD1, and TLE4 were ranked among the top 50 discriminating genes for the synovial sarcoma, and both WNT5A and FZD1 showed heavy staining on the tissue microarray as well. Uren et al. showed evidence that Wnt/Frizzled signaling is functional in Ewing sarcoma cell lines [145]. They observed a marked stimulation of the β-catenin/canonical Wnt pathway in ES cells treated with Wnt-3a. Wnt-3a induced morphologic changes characterized by the formation of long cytoplasmic extensions in ES cells. β-catenin/canonical Wnt signaling enhanced ES motility, contributing to metastasis, and it could occur through either autocrine or paracrine modes of Wnts since they are expressed in bone, muscle, and soft tissues. They also observed chemotaxis of ES cells in response to Wnt-3a. Both canonical and non-canonical Wnt pathways have been shown to modulate cell motility and tumor metastasis, but non-canonical Wnt pathway is pending of demonstration in ES.
34.6.3 Homeobox and Early Developmental Genes
The homeobox genes are involved in early embryonic development and in the determination of cell fate. There is interest in defining the relationship between deviant expression of these early developmental genes and their role in cancer. Specifically, aberrant homeobox gene expression has been linked to the development of leukemia, testicular cancer, breast carcinoma, as well as several other tumors [155]. Several homeobox genes have been identified in the gene expression profiles of various sarcomas: MEOX2 in synovial sarcoma [144], HOXA5 in liposarcoma [60], and MEOX1 in dermatofibrosarcoma protuberans [156].
34.6.4 Study of Cell Regulation by Proteomics: Proteomic Analysis of Responses to Gene Regulation, Chemicals, Viral Infections, or Therapeutic Treatment in Sarcoma
Alternative proteomics approaches can also combine the disciplines of protein chemistry, molecular biology, and histopathology to paint a portrait of the protein circuitry in diseased cells. It allows the identification of the molecular circuitry of various proteins in a tumor by noting their state of activation (translocation and phosphorylation) and correlative expressions. So far, this approach has been only utilized to depict the mTOR pathway in mesenchymal chondrosarcoma [157].
The combination of 2-DE, MALDI-ToF MS, and bioinformatics offers to the biomedical researcher an opportunity to study the profile of changes in protein levels. Taking advantage of such combination of proteomic tools, and to better understand the mechanisms by which ascochlorin (ASC) regulates physiological or pathological events and induces responses in the pharmacological treatment of cancer, Chang and collaborators performed differential analysis of the proteome of the human osteosarcoma cells U2-OS in response to ASC [158]. In addition, the authors established the first two-dimensional map of the U2-OS proteome. They identified 117 proteins whose expression showed consistent differences in their expression patterns with ASC treatment. Most of the proteins downregulated in U2-OS cells treated with ASC are associated with tumor growth, suggesting that ASC may be useful as a potent clinical suppressor of tumor invasion, a topic of considerable interest in the biological chemistry of chemotherapeutic agents.
In many cases, as seen in the previous examples, sarcoma cell lines are employed as model systems wherein mechanistic molecular schemes are studied. Control of cell-cycle progression, mediation of invasion and migration, evasion of apoptosis, etc. constitute checkpoints that are usually investigated in cell line models of different types of tumors in response to different agents. E2F1, for example, is an essential transcription factor that regulates cell-cycle progression and apoptosis. Overexpression of E2F1 sensitizes neoplastic cells to apoptosis and leads to tumor growth suppression, making it an interesting target for anticancer therapy. Li et al. performed a differential proteome analysis to identify proteins associated with E2F1 activity in inducible p53-deficient Saos-2ERE2F1 osteosarcoma cells [159]. Thirty-three proteins were reproducibly identified, and most of them were the products of genes known to be cancer related. Proteome analysis provided, in this case, new information that may be considered when using E2F1 as a drug.
Using different models of sarcoma xenografts (SK-N-MC and IMR32 (neuroblastoma), RH1 and RH30 (RMS), and KHOS/NP (osteosarcoma), Izbicka et al. evaluated and compared the effects of docetaxel and paclitaxel [160]. The approach used immunoblotting and surface-enhanced laser desorption/ionization (SELDI) MS to assess the drug effects on the expression of the beta-tubulin isotypes and apoptotic markers (Bcl-2, Bax, Bcl-XL). However, the results of this anticancer activity showed no apparent correlation with drug effects on those proteins. The drugs had significantly different, yet highly heterogeneous effects on the tumor levels of the proteins. In contrast, six protein species identified by proteomic profiling were consistently and differentially regulated by docetaxel and paclitaxel in all xenografts.
The mechanisms underlying the clinical outcome of interferon alpha (IFNalpha) treatment of pleomorphic sarcoma (PS) were also investigated using proteomic approaches. A proteomic analysis of 120 signaling components in growth arrested, apoptotic PS cells showed that the relative endogenous expression levels of the IFNAR2 (the IFNalpha/beta receptor) isoforms influence the cytostatic and pro-apoptotic effect of IFNalpha on PS cells [161]. The levels of the receptors may dictate the signaling pathways triggered by the ligand, such as to cause exclusively cell cycle arrest or induce programmed cell death.
The study of ES has provided, as well, a few examples of how proteomics may deepen our understanding about the mechanisms regulating the response to different treatments. The disease expresses several deregulated autocrine loops mediating cell survival and proliferation that contribute to its pathogenesis. Insulin-like growth factor I receptor (IGF1R) and KIT are transmembrane receptors that mediate two of these loops [139, 162], and are therefore directly involved in the growth and survival properties of ES [163–165]. Their blockade is a promising therapeutic approach for this neoplasm. Martins et al. reported the in vitro impact of IGF1R/KIT pathway blockade on ES cell lines [166] and afterwards they extended their observations to the level of proteomic changes induced by this treatment, to find and validate new possible therapeutic targets. Two-dimensional PAGE analysis and MALDI-ToF studies of ES cell lines treated with ADW742 and/or Imatinib (specific IGF1R/KIT inhibitors) revealed a large panel of differentially expressed proteins, some of them related to stress-induced response. Among them, the changes in protein expression between cell lines sensitive and resistant to IGF1R/KIT inhibitors were particularly significant in the case of HSP90 [167].
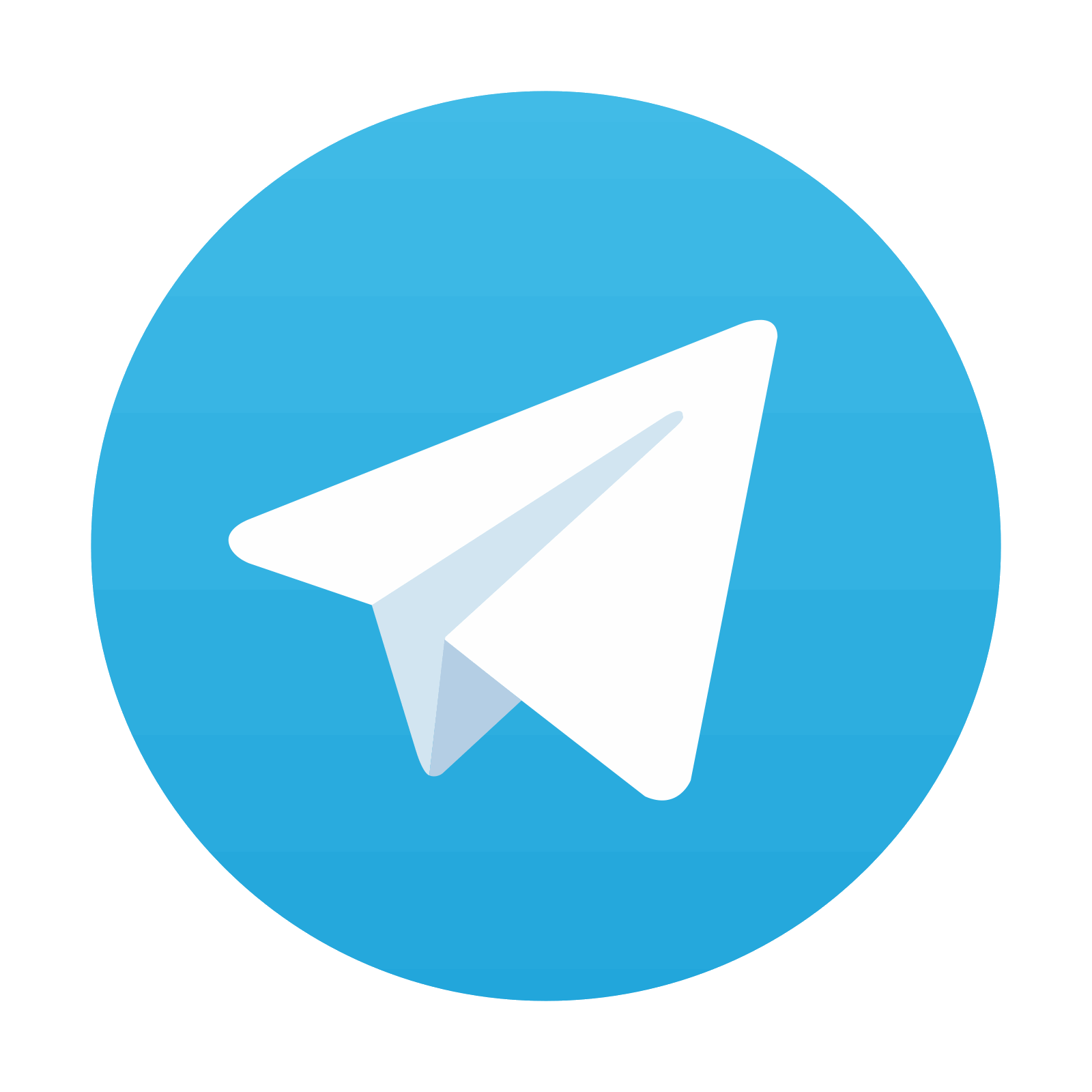
Stay updated, free articles. Join our Telegram channel
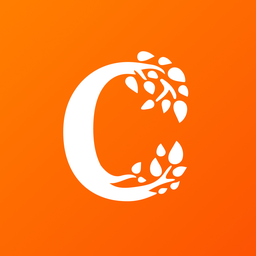
Full access? Get Clinical Tree
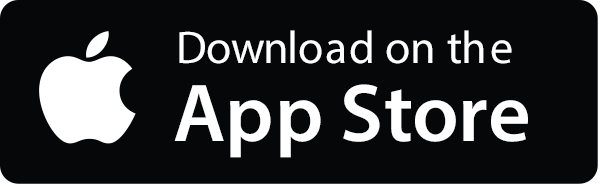
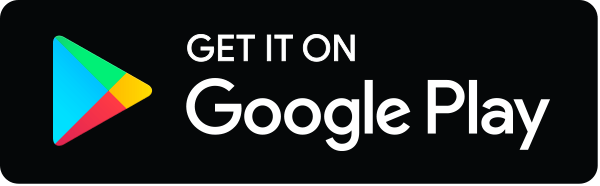