Fig. 12.1
Hallmarks of cancer in the tumor progression continuum. The indicated phenotypes along the tumor progression continuum do (+), do not (−), or sometimes (+/−) involve the indicated properties as described by Hanahan and Weinberg [79]. Note that not all of the hallmarks are present at each stage along the entire progression. The invasion and metastasis hallmarks have been separated according to the discussion in the text. Also, the distinction has been made between the potential to form micrometastasis from development of macroscopic metastases. Examples of mouse femurs with microscopic and macroscopic metastasis are shown. The fluorescent images are from human breast cancer cells labeled with GFP that were injected intra-cardiac into athymic mice.
12.2 Tumor Heterogeneity: Generation of a Metastatic Cell
The definition of metastasis is the dissemination of neoplastic cells to discontiguous nearby or distant secondary (or higher order) sites where they proliferate to form a mass [1, 7] (Fig. 12.2). Fortunately, this process is highly inefficient [8]. Of the millions of cells that enter the vascular compartment per gram of primary tumor per day, only small fractions (much less than 0.1 %) actually form a macroscopic mass [9, 10]. Just as most tumors are clonal in origin [5, 11, 12], each metastasis arises from a single cell [6, 13, 14]. By the time the primary mass is apparent to the individual or the diagnosing physician, it is usually composed of 1010 or 1011 cells based on the fact that a cubic centimeter of tissue contains approximately 109 cells. Although not all cells in a neoplasm are capable of completing the required steps for metastasis, histological examination reveals that these cells are pleiomorphic and that single cell clones isolated from a tumor vary dramatically in terms of biological behavior [5, 6, 11].


Fig. 12.2
Steps of cancer metastasis. The necessary steps for metastasis are listed in order. In the first step, tumor growth is noted with an increase in desmoplasia (1) host response to the presence of the tumor mass. Tumor cells invade away from the primary tumor (2) and eventually intravasate (3) into a circulatory compartment. Emboli involving multiple tumor cells and platelets/thrombin (4a) often occur. However, most tumor cells succumb to sheer or anoikis (4b) following entry into the vasculature. Tumor cells then specifically adhere to endothelial cells lining vessels (5), depending upon reciprocal recognition of surface molecules or arrest due to size limitations (6) at vessel bifurcations. Some cells can proliferate intravascularly (6); whereas, others extravasate (7) prior to proliferating at the secondary site (8).
Heterogeneity describes how cancers are composed of cells which are inherently and/or behaviorally different. The basis of the behavioral differences can be genetic, epigenetic, positional, and temporal. Genetic refers to the inherent properties of tumor cells themselves. Genetic heterogeneity is demonstrated by isolation of single cell clones that stably differ for a given phenotype. Like genetic heterogeneity, epigenetic heterogeneity refers to the chemical modifications of DNA and chromatin that lead to the selective regulation of gene transcription. Epigenetic changes can occur because of position (positional heterogeneity), for example the accessibility of a cell to external stimuli (O2, pH, growth factors, cytokines, chemokines, etc.). An example is noted with radiation sensitivity being proportional to oxygenation. Thus, two identical cells would exhibit differences in radio-response depending upon distance from a capillary since oxygen tension is inversely proportional to distance from blood. Heterogeneity can be observed temporally as well, as cells change due to cyclical signals. One example would be that cells in the G0/G1 phase of the cell cycle would be less sensitive than cells in S phase to drugs targeting DNA replication.
At first glance, isolation of multiple cell subpopulations from virtually every tumor mass would appear to support the hypothesis that cancers are of multicellular origin. However, tumors express either maternal or paternal isoenzymes, but rarely both, strongly supporting unicellular origination. In addition, analysis of karyotypes reveals that virtually all cells within a tumor share common abnormal chromosomal changes upon which additional karyotypic abnormalities may be superimposed. Therefore, the majority of tumors are monoclonal in origin and it is the divergence of single transformed cells into multiple phenotypically distinct progeny that generates genetic heterogeneity [5, 11].
As with most aspects of tumor biology, development of heterogeneity is not unique to tumor progression. Pluripotent hematopoietic stem cells generate cells along multiple lineages, and a zygote yields a multicellular organism with organs and tissues. Stem cells have the capacity for self-renewal and progenitor production via asymmetric cell division and are regulated by the microenvironmental niche [15, 16]. Although stem cell theory accommodates diversification, the molecular mechanisms underlying differentiation and diversification of normal cells are still being elucidated [17]. Additionally, the relevance of cancer stem cell theory is still being debated by many researchers to account for the diversification of cancer cells [18–23].
One of the first formalized conceptual frameworks of tumor progression was introduced by Peyton Rous, who described the steps involved in the transformation of skin carcinomas from the study of tar tumors in rabbits [24]. His concepts were expanded by Leslie Foulds, who studied the acquisition of hormone independence by mammary tumors. Foulds defined progression as “…the acquisition of permanent, irreversible qualitative changes of one or more characteristics in a neoplasm…” [25]. Both Rous and Foulds provided evidence that tumor progression occurs in a constant, unique and stepwise pattern. The trend is toward increased autonomy; however, individual characteristics within a tumor independently assort [6].
The mutation-selection theory of tumor progression proposes that genetic instability drives the process by random generation of variants (Fig. 12.3). Expanding upon Theodor Boveri’s original observations that alterations of chromosomal material were significant in the generation and progression of tumors [26], Peter Nowell proposed that neoplastic cells are more genetically unstable than normal counterparts [27]. Fluctuation analyses for a variety of genes and phenotypes show that transformed cells are significantly (frequently 10,000- to 100,000-fold, but as high as 107-fold higher) more genetically unstable than normal counterparts [11]. Progression is believed to occur as a result of mutation and coupled selection via Darwinian selection principles [6]. Although not formally proven, subpopulations of cells that have acquired the ability to migrate and establish themselves at other sites are thought to have a selective advantage since these tumor cells are not limited by space or location.


Fig. 12.3
Mutation-selection theory of tumor progression. Although mutation rates can be highly variable according to specific cell types, tumor cells generally have higher mutation rates than normal cells. Tumor cells with higher mutation rates (upper series) have increased chances for survival after lethal selection pressures. Variants are selected continually and are either eliminated by selection or are overwhelmed by other cells with more robust growth characteristics. Populations of cells with low mutation rates are more susceptible to lethal selection pressures (bottom series of cells).
Isaiah “Josh” Fidler and Margaret Kripke tested these hypotheses with regard to the metastatic phenotype using combinations of cloning and Luria–Delbrück fluctuation analysis [28]. Single cell clones isolated from a single tumor varied considerably in their metastatic potentials. George Poste and colleagues later showed that highly metastatic cells, when grown in culture continuously and re-cloned, yielded populations that contained non-metastatic or poorly metastatic cells [29, 30]. Likewise, continuous culture of poorly metastatic cells yielded subpopulations that were highly metastatic. In other words, the clonal populations did not remain homogeneous.
At an organismal (i.e., tumor) level, progression typically follows a sequence (Fig. 12.1). Prior to becoming tumorigenic, cells lose the ability to differentiate fully, are no longer contact inhibited or anchorage dependent and have acquired genetic instability. The ability to form a neoplastic mass typically goes through a phase with expansile growth in the absence of invasion. While cells may be pleiomorphic at this stage, they are often encapsulated by a dense fibrous network (i.e., desmoplasia) [31, 32]. Tumors that have failed to invade through a basement membrane are referred to as benign or as carcinoma in situ. With continued generation of variants and selection, subsets of the cells acquire the ability to escape through a basement membrane, the hallmark of malignancy. Acquisition of the ability to detach from the primary tumor and move elsewhere is required for metastasis. It is important to emphasize that tumor progression is typically measured in terms of the tumor mass, rather than the individual cells within it. The stage of a tumor is defined by the most malignant cells found. Even if 99.9 % of cells are indolent, a tumor is defined as malignant if a single cell has penetrated a basement membrane.
At a molecular level , certain chromosomal and genetic changes are more prevalent in early versus late stages of tumor progression, despite unpredictability in specific genetic changes occurring within a cell. Use of this information has allowed prediction of genetic underpinnings controlling tumorigenesis, invasiveness, and metastasis [6]. The complexity of tumor progression leading to a metastatic cell, as described above, shows that a single genetic change is not enough to accurately predict whether a person will have an increased chance of a lesion becoming metastatic and defined subsets of genes have become more predictive as prognostic tools [33, 34].
However, the mutation-selection theory of tumor progression is not without its detractors. Some argue that the acquisition of invasive and metastatic behaviors is more a recapitulation of a process that occurs during embryogenesis—the epithelial–mesenchymal transition (EMT) [35]. Since invasive cells frequently dramatically change their cell shape to a non-polarized, motile, spindle shaped cell resembling a fibroblast, some hypothesize that neoplastic cells dedifferentiate to a more motile mesenchymal cell phenotype. Developmental EMT and cancer EMT are not necessarily equivalent at a molecular level, but share some characteristics. Cancer EMT is characterized molecularly by the loss of epithelial-specific E-cadherin from the adherens junctions, and a switch from the expression of keratins as the major intermediate filament to the mesenchymal intermediate filament vimentin. Ultimately, some believe that more epigenetic mechanisms may be driving tumor progression toward malignancy than mutation and selection [36].
12.3 Invasion
Invasion, the distinguishing feature of malignancy, is the capacity for tumor cells to disrupt the basement membrane and penetrate underlying stroma. Although invasion is required for metastasis, the ability to invade is not sufficient. This point is highlighted by clinical observations. Some tumors are highly aggressive, forming secondary lesions with high frequency (melanoma, pancreatic carcinoma, small cell carcinoma of the lung), whereas others are rarely metastatic despite being locally invasive (basal cell carcinomas of the skin, glioblastoma multiforme). It should be emphasized that if an invasive cell cannot complete any of the subsequent steps in the metastatic cascade, it will not form a metastasis.
The process of invasion requires major changes to the cell (morphology and phenotype) and to the surrounding environment. During invasion, three important processes are dynamically regulated that include adhesion, extracellular matrix (ECM) reorganization, and motility. Normal epithelial cells form polarized sheets that are maintained by tight junctions and desmosomes. They are anchored to the basement membrane by hemidesmosomes and their associated intermediate filaments, and integrin contacts that organize actin. Therefore, to be invasive, cells must alter cell-to-cell and cell-to-matrix adhesion in conjunction with reorganization of the ECM and cellular motility [37, 38]. The structural and functional proteins that regulate cell adhesion and migration are key downstream targets of oncogenes and tumor suppressor-controlled signaling pathways and provide insights into how oncogenic transformation results in progression to an invasive phenotype. Many of the proteins involved in tumor invasion have also been observed to affect other processes that are part of the hallmarks of cancer including cell survival, growth, apoptosis and angiogenesis. This highlights the intricate network of interrelated pathways controlling cell behavior [39].
The dramatic changes to a tumor cell morphologically during invasion have been referred to as EMT, which describes the conversion from an epithelial morphology to a non-polarized, motile, spindle shaped cell resembling a fibroblast [35, 40, 41]. EMT is associated with the loss of epithelial-specific E-cadherin from the adherens junctions, and a switch from the expression of keratins as the major intermediate filament to the mesenchymal intermediate filament, vimentin. EMT is influenced by the tumor microenvironment, and has been observed primarily at the edge of the tumor mass that is in contact with the tumor stroma [42]. A key regulator of EMT is transforming growth factor beta (TGF-β) signaling but other mediators include hepatocyte growth factor/scatter factor (HGF/SF), PI3 kinase signaling pathway, MAP kinases, and the transcriptional regulators Twist and Snail. Other signaling pathways implicated in stem cell maintenance that are linked to EMT are Wnt, Notch and Hedgehog. Tumor cells may also reverse the process and undergo a mesenchymal-to-epithelial transition (MET) in the absence of EMT-inducing signals. This transient nature of EMT helps explain why metastatic cells can morphologically resemble cells in the primary tumor despite the fact that they by necessity accomplished all the steps of the metastatic cascade [40, 43].
Epithelial cell–cell interactions are mediated primarily by cadherins, transmembrane glycoproteins that form calcium-dependent homotypic complexes. The loss of E-cadherin that occurs during EMT correlates with increased invasion and metastatic potential in most tumor types and reexpression in experimental models can block invasion. This observation suggests that loss of E-cadherin is causative for invasion. E-cadherin loss occurs at multiple levels including transcriptional repression and proteolytic degradation. The zinc finger transcriptional repressors Snail and Slug in particular have been implicated in regulating EMT by virtue of their ability to repress E-cadherin transcription. Cadherins are regulated by catenins (α, β, γ and p120 catenins), cytoplasmic proteins that functionally link the cadherin complex to the actin cytoskeleton. β-Catenin is both a cell adhesion protein and a transcription factor. In addition to its role in adherens junctions, it participates in canonical Wnt signaling, a signaling pathway important in development and cancer. E-cadherin levels and function are also disrupted by loss of p120 catenin, which occurs in many tumor types and may also contribute to tumor metastasis.
E-cadherin is not the only cell–cell adhesion molecule associated with invasion and metastasis. NCAM, a member of the immunoglobulin cell adhesion molecule Ig-CAM family, is downregulated in several tumor types, and NCAM loss results in an increased ability of tumor cells to disseminate [44, 45]. Other Ig-CAMs, such as DCC, CEACAM1, and Mel-CAM, also demonstrate reduced expression in specific cancer types. It should be noted, however, that not all cell–cell adhesion molecules can be viewed as potential invasion suppressors. Several are overexpressed in advanced cancers and have functions associated with cancer progression including Ig-CAMs such as L1, CEA, and ALCAM. Additionally, N-cadherin promotes cell motility. This complexity may be explained by direct or indirect signaling functions for these molecules that are distinct from their role in cell–cell adhesion. The interrelatedness of tumor growth and tumor invasion, and limitations of experimental model systems, does not always allow a distinction between growth effects that influence the appearance of an invasive phenotype and an effect on actual cellular invasion.
Cells induced to undergo EMT not only exhibit enhanced motility but are resistant to apoptosis, another key requirement for successful metastasis. However, some cancer cells use EMT independent modes of migration including collective and amoeboid [38]. Although EMT and MET are well accepted processes necessary for normal development and can be demonstrated and manipulated in many experimental tumor models, it has been questioned whether they are actual requirements for human cancer progression.
The ECM provides a scaffold for the organization of cells and spatial cues that dictate cell behavior [46]. This matrix is composed of proteins, primarily triple-helical collagens, glycoproteins such as laminins and fibronectin, and proteolgycans. The basement membrane is an organized ECM that forms a barrier separating polarized epithelial, endothelial, and muscle cells from the underlying tissue. Interstitial matrix provides the structural characteristics of connective tissues. The molecular composition of the ECM varies between tissues and organs, and provides important contextual information to cellular constituents. In addition, the ECM interacts with many secreted molecules to serve as a repository for regulatory proteins and growth factors. Thus, the interaction of cells with ECM molecules dictates their ability for survival, growth, differentiation, and migration. Moreover, selective proteolysis of ECM components leads to release of fragments that further regulate protein function and may be involved in cell signaling. These factors are collectively known as matrikines .
Adhesion of cells to their surrounding matrix occurs primarily through a family of transmembrane glycoproteins known as integrins that are assembled as specific combinations of 18 alpha and 8 beta subunits [47, 48]. Each combination of integrin subunits binds to distinct but overlapping subsets of ECM components and may be either tumor promoting or inhibitory. During tumor progression, cancer cells tend to downregulate the integrins that mediate adhesion and maintain a quiescent, differentiated state, and upregulate integrins that promote survival, migration, and proliferation. Although there is a cell-type dependency on integrin function, generally integrins α2β1 and α3β1 are viewed as suppressors of tumor progression, while αvβ3, αvβ6, and α6β4 promote cellular proliferation and migration. Integrins mediate signals in both directions, so that changes in intracellular signaling pathways can modulate cellular adhesion, and changes in cellular adhesion can alter cellular phenotype. A well-described and important mechanism whereby integrin-ECM interactions modulate cell function is by cooperative signaling with different growth factor receptors. Many of the cellular responses induced by activation of tyrosine kinase growth factor receptors are dependent on the cells being able to adhere to an ECM substrate in an integrin-dependent fashion. Signaling in response to ECM interaction usually activates focal adhesion kinase (FAK) and non-receptor tyrosine kinases of the src-family.
The ECM is remodeled by degradative enzymes that are produced by the tumor cells themselves and additionally by the resident and infiltrating cells as a response to the tumor. These enzymes contribute to matrix degradation and facilitate tumor cell invasion. Proteolytic enzymes of many classes have been implicated in tumor cell invasion, including but not limited to the serine proteinases plasmin, plasminogen activator, seprase, hepsin, and several kallikreins, the cysteine proteinase cathepsin B, the aspartyl proteinase cathepsin D, and metal-dependent proteinases of the matrix metalloproteinase (MMP) and a disintegrin and metalloproteinase (ADAM) families. Other matrix degrading enzymes such as heparanase, an endoglycosidase which cleaves heparin sulfate proteoglycans, and hylauronidase cleavage of its substrate hylauronic acid, have also been causally associated with tumor progression and invasion.
Liotta and colleagues observed that metastatic potential correlates with the degradation of type IV collagen found predominantly in the basement membrane and focused attention on the metal-dependent type IV collagenases or gelatinases that are now recognized as MMP-2 and MMP-9. Subsequently, many of the 23 members of the MMP family of matrix-degrading metalloproteinases have been associated with tumor progression. Elevated MMP levels correlate with invasion and metastasis and poor prognosis in many cancer types, and animal models provide evidence for a causal role for MMP activity in cancer progression [49–51]. Additionally, the plasminogen activator/plasmin system has been causally implicated in cancer invasion, and urokinase plasminogen activator (uPA) and plasminogen activator inhibitor-1 (PAI-1) are validated prognostic and predictive markers for breast cancer.
Regulation of matrix proteolysis occurs at multiple levels and in addition to the expression of proteases by both the tumor cells and adjacent resident and infiltrating cells, they produce specific endogenous inhibitors including the tissue inhibitors of metalloproteinases (TIMPs), serine proteinase inhibitors (SERPINs), and cysteine protease inhibitors (CYSTATINs). Some of these inhibitors are stored in high concentrations within the ECM and paradoxically may be required for activation of the protease . The conversion of pro-MMP-2 to active MMP-2 requires the activity of MT1-MMP (MMP-14), a transmembrane MMP that is activated intracellularly by the propeptidase family member furin, and TIMP-2 (Fig. 12.4). The concentration of each of these molecules is critical for proper function and regulation. Another proteolytic cascade important for regulating protease activity during the degradation of ECM is cathepsin(s) → uPA → plasmin → MMP. Each of the proteases in this cascade is capable of cleaving components of the ECM. Therefore, activity cascades are important regulators of proteolytic function in addition to endogenous inhibitors, protease degradation, and activation.


Fig. 12.4
Example of a proteolytic activation cascade . The proteolytic activities are intricately regulated at several levels during the MMP-2 activation cascade. The activation of proMMP-2 occurs by the balance of TIMP-2, MT1-MMP, and itself. MT1-MMP is activated intracellularly by furin and localized to the plasma membrane. The TIMP-2N-terminal (N) domain binds to and inhibits MT1-MMP activity and recruits proMMP-2 by interaction of TIMP-2 C-terminal (C) domain to proMMP-2 hemopexin domain (Pex). An adjacent MT1-MMP activates proMMP-2 to MMP-2 through cleavage of the pro-domain (P).
The original view that proteolytic enzymes function predominantly to remove physical ECM barriers has been expanded with the realization that proteolysis regulates multiple steps of tumor progression. For example, MMP substrates in the matrix or on the cell surface that modulate cellular growth, differentiation, apoptosis, angiogenesis, chemotaxis, and migration have been identified. The abundant evidence for a role for MMPs in tumor progression led to the design and testing of synthetic MMP inhibitors for cancer therapy. These inhibitors proved to be ineffective in clinical trials, results that have been explained by problems with inhibitor or clinical trial design, as well as a lack of understanding of the broad range of MMP activities resulting in both cancer-promoting and cancer inhibitory effects.
In addition to ECM remodeling, locomotion of a cell occurs through the coordination of polymerization and depolymerization of the actin cytoskeleton to extend a pseudopod at the leading edge of the cell, followed by contraction associated with disassembly of cell–matrix adhesive contacts at the trailing edge [52]. Lamellipodial protrusions at the leading edge are nucleated by a branched actin network involving the Arp2/3 complex and its regulators, the WASP family, cortactin, and the GTPase Rac. Actin contractility is regulated by myosin light chain kinase and upstream small GTPases, in particular Rho and its effector ROCK. As alluded to above in the discussion of EMT, single cells migrate either with a spindle-shaped morphology, referred to as mesenchymal migration, or with the less adhesive ellipsoid shape used by leukocytes and Dictyostelium termed amoeboid migration. Collective migration can occur when the cells retain cell–cell junctions and clusters of cells move in single file through a tissue.
Tumor cells can secrete factors that stimulate motility in an autocrine fashion. Tumor cell-produced lysophospholipase D (autotaxin) stimulates motility, as does lysophosphatidic acid (LPA), which can be produced by lysophospholipase D activity on lysophosphatidylcholine. Hepatocyte growth factor/scatter factor (HGF/SF) interacts with its receptor, c-met, to induce chemokinetic activity of epithelial cells, resulting in an invasive phenotype. Directional motility is a chemotactic (following a soluble concentration gradient) or haptotactic (following an insoluble concentration gradient) effect in response to a gradient of soluble or localized factors, respectively. Chemotaxis is often the result of growth factors such as insulin-like growth factor (IGF), and chemokines of the CCR and CXC family. Haptotaxis is characterized as a response to gradients of ECM components such as laminin-5 and fibronectin, and can be modulated positively or negatively by proteolysis [53].
The coordination of cell–cell and cell–matrix adhesion, matrix degradation, and cytoskeletal activity is required for cellular invasion. The type of cell migration (collective, mesenchymal, or amoeboid) is influenced by the relative levels of adhesion mediated by cadherins and integrins, proteolytic activity, and actin contractility. Modulation of any of these factors is thought to convert one type of motility into another. Structures identified in invading cells in three dimensions that represent the physical convergence of the adhesive, proteolytic, and motility component of invasion are known as invadopodia [54]. Invadopodia are actin-rich organelles that protrude from the plasma membrane and contact and locally degrade the ECM [55–58]. Invadopodia contain adhesion molecules, including several β1 integrins and CD44, and the proteinases seprase, dipeptidyl dipeptidase IV, and several MMP and ADAM metalloproteinases. Inside the plasma membrane, invadopodia contain actin and actin assembly molecules, as well as multiple signaling molecules including FAK, src associated proteins such as p130Cas and Tks5/FISH, and the small GTPases cdc42, Arf1, and Arf6. Thus, invadopodia are implicated as key cellular structures that are used to coordinate and regulate the various components of the process of cancer invasion.
12.4 Further Steps Required for Metastasis
Invasion and metastasis are not equivalent phenotypes. Although invasion is prerequisite for metastasis, the ability to invade is insufficient to develop a metastasis. Once cells have invaded away from the primary tumor, they disseminate. Most commonly, metastasis is described in terms of hematogenous (blood-borne) dissemination. However, secondary tumors can arise because tumor cells have migrated via lymphatics (i.e., lymph nodes are common sites of metastasis for most carcinomas) or across body cavities (e.g., ovarian carcinoma cells most frequently establish secondary tumors by dissemination in the peritoneum while rarely forming metastases via hematogenous spread) [59]. Additional examples are dissemination of melanoma cells along the space between endothelium and basement membrane or perineural spread in pancreatic and prostatic carcinomas. Some question whether non-hematogenous spread is actually metastasis. We submit that the route of dissemination is irrelevant to the definition of metastasis, as long as the cells are discontinuous from the primary tumor mass. Nonetheless, for the remainder of this chapter, we will focus on blood-borne metastasis since this route is responsible for the majority of visceral metastases.
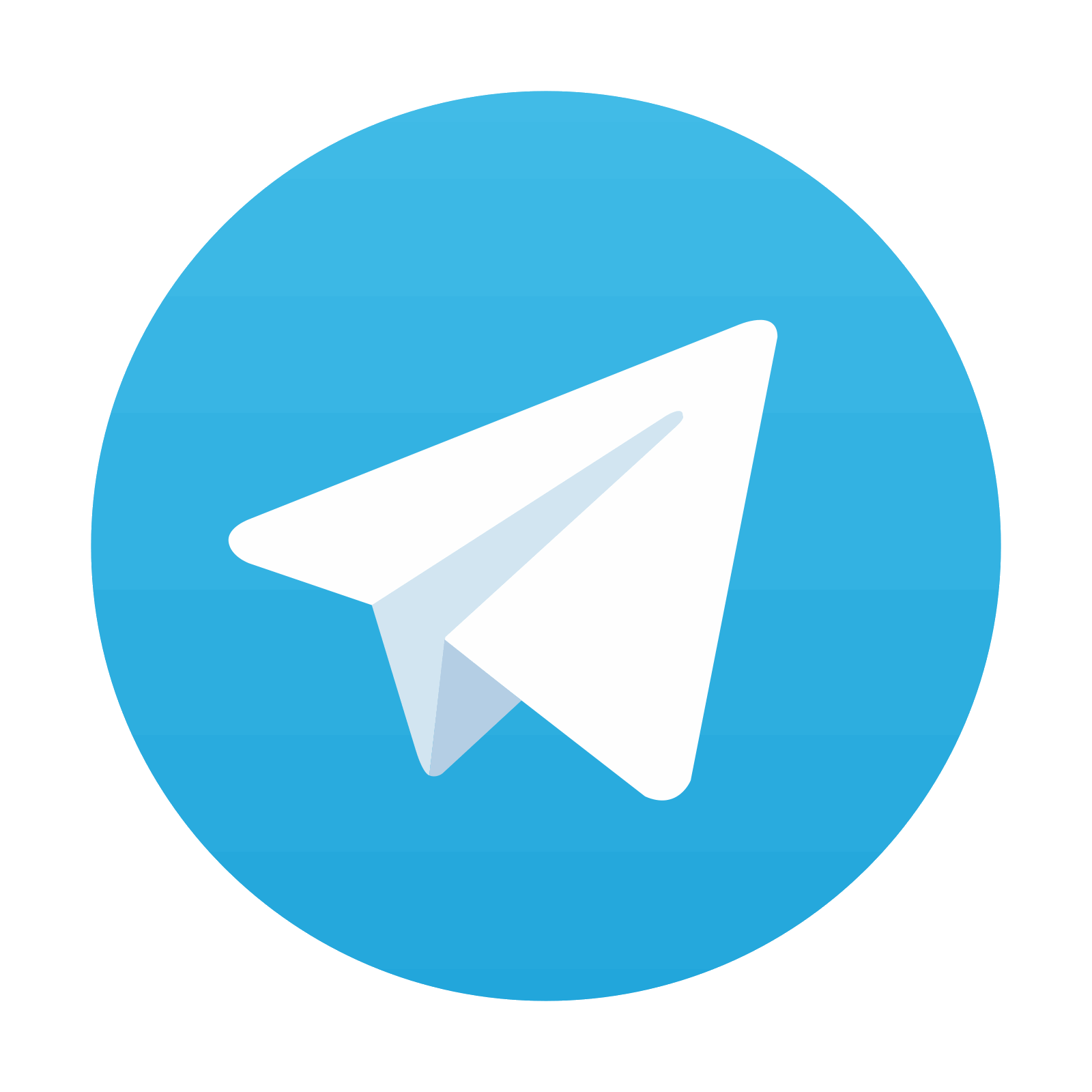
Stay updated, free articles. Join our Telegram channel
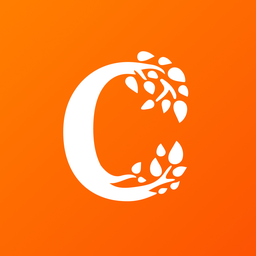
Full access? Get Clinical Tree
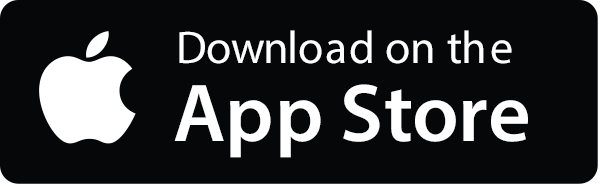
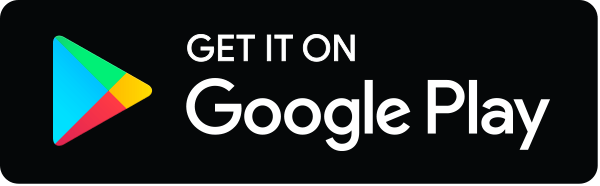