Measuring Serum Thyroid–Stimulating Hormone, Thyroid Hormones, Thyroid-Directed Antibodies, and Transport Proteins
Offie P. Soldin
Introduction
Thyroid function tests (TFTs) are among the most commonly requested laboratory tests (1). The aim of this chapter is to present relevant information on analytical and clinical aspects of serum-based laboratory tests most frequently used in clinical practice to assess thyroid function.
Thyroxine (T4) and triiodothyronine (T3) production is stimulated by pituitary thyroid–stimulating hormone (TSH, thyrotropin), the synthesis of which is regulated by hypothalamic thyrotropin–releasing hormone (TRH). In turn, T4 and T3 inhibit TRH and TSH production by negative feedback, thus establishing an equilibrium; this “setpoint” is tightly regulated within an individual but varies among subjects, likely reflecting genetic and other factors (2). Cellular deiodinases mediate thyroid hormone metabolism at a pre-receptor level, and three nuclear receptor subtypes with differing tissue expression, encoded by the THRA and THRB genes, mediate thyroid hormone actions (3).
Since the diagnoses and the clinical strategies for detecting and treating thyroid disorders are based on the measurement of serum TSH, T4, and T3, it is critically important that their assays are reliable. The specificity and sensitivity of TFTs (TSH, T4, and T3 tests) have progressively improved from the competitive immunoassays of the early 1970s and noncompetitive immunometric assays (IMAs) to the more recent liquid chromatography tandem mass spectrometry (LC/MS/MS). Assay methodologies continue to evolve as performance standards are established and new technology and instrumentation develop. These assays include serum-based assays of free thyroid hormones (FT4 and FT3), total thyroid hormones (TT4 and TT3) (4,5), thyroxine-binding globulin (TBG), transthyretin (TTR/prealbumin) and albumin (6), in addition to more sensitive and specific tests for autoantibodies to thyroid peroxidase (TPO), thyroglobulin (Tg), and the TSH receptor (TR) (7,8).
The interpretation of TFTs is often straightforward with the combination of TSH and thyroid hormone measurements confirming euthyroidism, hypothyroidism, or hyperthyroidism consistent with a patient’s clinical status. However, in a small but significant number of cases, the laboratory results either do not fit with the clinical picture or they form an unusual, nonphysiologic pattern. To prevent misdiagnosis or inappropriate management, it is necessary to reassess the clinical context and recognize potential pitfalls such as assay interference. Moreover, physicians need to be aware that thyroid tests in general have a low index of individuality necessitating that the test results be interpreted relative to patient-specific factors.
Thyroid-Stimulating Hormone
TSH is a 28-kDa glycoprotein released from thyrotrophs in the anteromedial region of the pituitary. TSH is a heterodimer consisting of together α and β subunits that are tightly, but noncovalently, bound (9). The α subunit is common to TSH, follicle-stimulating hormone (FSH), luteinizing hormone (LH), and human chorionic gonadotropin (hCG). The β subunit confers specificity to the molecule since it interacts with the thyroid cell TSH receptor and is rate limiting in the formation of the mature heterodimeric protein. However, the free β subunit is inactive and requires noncovalent combination with the α subunit to express hormonal bioactivity.
Functional Versus Analytical sensitivity of TSH Assays
Analytical sensitivity is defined as the lowest serum concentration of TSH that can be distinguished by statistical analysis from the zero standard with a single assay when multiple replicates are analyzed. It is not suitable for estimating sensitivity since clinical laboratories do not routinely analyze samples in replicates. In addition, the sensitivity of a single assay does not represent the day-to-day variability that occurs in routine use. The key to identifying assay sensitivity lies in constructing a precision profile—a plot of the coefficient of variation (CV) against serum TSH concentration, using results obtained over a long period of time from multiple batches of reagents. Functional sensitivity has been defined as the TSH concentration associated with a 20% between-run CV determined by a protocol that allows for changes in operator and reagent batch and is assessed in at least 10 different assay runs and established from assays run over a 6 to 8 week period (typical interval for measuring TSH during clinical management) using at least two reagent lots and instrument calibrations (10) (Fig. 13A.1). Clinical laboratories and manufacturers have adopted the functional sensitivity definition as the lowest reporting limit for TSH assays. The use of functional sensitivity gave rise to
the “generation” nomenclature for TSH assays, with each generation as having a 10-fold difference in functional sensitivity.
the “generation” nomenclature for TSH assays, with each generation as having a 10-fold difference in functional sensitivity.
Clinical decisions based on the measurement of low TSH may be compromised if an assay performs inconsistently or its functional detection limit is insufficient to discriminate between values in the low range. It is therefore essential that the serum TSH assays be sufficiently sensitive for clinical diagnostic purposes. Laboratories should confirm the functional sensitivity quoted by the manufacturer and have quality assurance procedures in place to ensure that it is regularly monitored. Consultation with the endocrine laboratory or clinical pathologist may be useful in determining the true functional capabilities of the assay in use.
Considerations for Serum TSH Measurements
The diagnostic superiority of TSH measurement arises principally from the inverse log/linear relationship between circulating TSH and FT4 concentrations (8,11,12). As a single hormone determination, serum TSH provides the most sensitive index to reliably detect thyroid function abnormalities (8,13,14). The early radioimmunoassays (RIAs), with functional sensitivity between 1 and 2 mIU/L, were designated as “first generation” and IMAs with functional sensitivity between 0.1 and 0.2 mIU/L were designated as “second generation.” Most commercially available assays have the potential to perform at the second-generation level and should be useful for the initial evaluation of both high- and low-TSH states. However, not all IMAs perform consistently in measuring very low levels of TSH, and the sensitivity of TSH assays used in clinical laboratories (functional sensitivity) may differ significantly from that stated by the manufacturer (analytical sensitivity)(13).
Most current nonisotopic, automated methods have functional sensitivity between 0.01 and 0.02 mIU/L and are designated as “third generation” (8). The current standards of care recommend the use of third-generation TSH assays with functional sensitivity <0.02 mIU/L (1,8,15,16). This level of sensitivity is necessary for detecting the differing degrees of TSH suppression. For example, TSH concentrations ranging between 0.01 and 0.1 mIU/L represent a significant risk for atrial fibrillation in older patients and are often an iatrogenic consequence of levothyroxine (LT4) suppression or an unintended result of replacement therapy (17,18). In addition, targeting the degree of TSH suppression plays a critical role in the management of thyroid cancer (19,20).
It is important to obtain serum TSH measurement when hypothyroidism or thyrotoxicosis is first suspected to establish the baseline thyroid status, and then when equilibrium has been reached or clinical status is changed.
Hyperthyroidism (suppressed serum TSH concentrations typically <0.1 mIU/L) includes cases of Graves’ disease, toxic adenoma and nodular goiter, subacute and lymphocytic (silent, postpartum) thyroiditis, iodine-induced hyperthyroidism, and exogenous thyroid hormone excess. Serum FT4 and FT3 measurements are indicated when TSH level is less than 4 mIU/L. In the case of Graves’ patients, TSH measurement should not be used to monitor therapy. Close monitoring of TSH and FT4 is recommended for pregnant women with thyroid disease or severely ill hypothyroid patients, who sometimes have malabsorption of oral T4. In severely ill patients with nonthyroidal illness (NTI), however, serum T4 assessment may be more informative than TSH (21).
Isolated abnormalities of serum TSH levels do not always connote sustained thyroid dysfunction. The causes of isolated TSH elevation may include: (a) Mild (subclinical) hypothyroidism, (b) recovery from hypothyroxinemia of nonthyroid illnesses, and (c) medications such as amiodarone, which can inhibit thyroid hormone synthesis and metabolism and may cause transient reversible elevation of serum TSH (22). Hyperthyroid patients are expected to have serum TSH concentrations of less than 0.01 mIU/L except in TSH-induced thyrotoxicosis and T4/T3 resistance. Patients with resistance to thyroid hormones may have mixed thyroid status, with hypothyroidism in some tissues and thyrotoxicosis in others, resulting in normal or mildly elevated TSH levels. Isolated TSH suppression is sometimes seen in cases of mild (subclinical) hyperthyroidism, recovery from overt hyperthyroidism, NTIs, pregnancy during the first trimester, and in patients using medications such as dopamine and high-dose glucocorticoids.
Methods for Measuring Serum TSH
TSH Immunoassays
The first generation of TSH assays (1965 to 1985), were based on RIA employing polyclonal antibodies that cross-reacted with hCG, had limited functional sensitivity (∼1.0 mIU/L), and were therefore unable to detect the lower limit of the euthyroid reference interval (0.4 to 4.1 mIU/L) (23,24). Their clinical utility was therefore limited to the diagnosis of primary hypothyroidism (25). The more sensitive IMA methodology (“sandwich” or “noncompetitive” methodology) replaced RIAs in the mid-1980s (26,27,28,29). These IMA assays, based on the excess antibody approach, provided a full order of magnitude greater functional sensitivity (0.1 mIU/L) and, therefore, could reliably detect the low-serum TSH values characteristic of hyperthyroidism (30,31). A different poly- or monoclonal
TSH antibody, targeted to a different TSH epitope(s) and labeled with an isotopic signal (I-125), was then added, followed by a further incubation and washing to remove unbound constituents. The radioactivity bound to the solid support was quantified as being directly proportional to TSH levels in the test sample. However, some patients have antibodies to mouse gamma globulins. These heterophile antibodies (HAbs) may affect assay results if mouse antibodies are used in the assay. Therefore, modifications to the basic concept were made by using two highly specific monoclonal antibodies raised to epitopes on the α and β subunits of the TSH molecule thus reducing interference by heterophile and human anti-mouse antibodies (HAMAs) (24,32).
TSH antibody, targeted to a different TSH epitope(s) and labeled with an isotopic signal (I-125), was then added, followed by a further incubation and washing to remove unbound constituents. The radioactivity bound to the solid support was quantified as being directly proportional to TSH levels in the test sample. However, some patients have antibodies to mouse gamma globulins. These heterophile antibodies (HAbs) may affect assay results if mouse antibodies are used in the assay. Therefore, modifications to the basic concept were made by using two highly specific monoclonal antibodies raised to epitopes on the α and β subunits of the TSH molecule thus reducing interference by heterophile and human anti-mouse antibodies (HAMAs) (24,32).
TSH assay sensitivity has been further enhanced by the adoption of nonisotopic (chemiluminescent and fluorescent) signals. IMA nonisotopic methods have inherently greater assay sensitivity and specificity, resulting in narrower TSH reference intervals due to reduced cross-reactivity and improved precision, and offer the additional advantage of being easier to automate (33,34). Automated third-generation immunometric TSH assays have become the current standard of care (8). Fourth-generation assays, which have a sensitivity of 0.001 to 0.002 mIU/L, have now been developed for research purposes (35).
The Clinical Utility of TSH Measurement
Serum TSH testing provides better sensitivity for detecting thyroid dysfunction than does FT4 testing (8,16,36,37). Moreover, TSH ranges vary slightly depending on differences between methods of analysis (38). In ambulatory hypothyroid patients, serum TSH concentrations are used as the target for adjusting LT4 dosing within a very narrow therapeutic index (39,40).
Serum TSH normally exhibits a diurnal variation with a peak between midnight and 04:00 and the lowest values between 10:00 and 16:00 hours (39). This variation should not influence the diagnostic interpretation of test results since most clinical TSH measurements are performed on ambulatory patients between 08:00 and 18:00 hours, and the reference intervals for TSH are typically established using specimens collected at a similar time. Further, because serum T4 with a half-life of approximately 7 days does not change sufficiently in 1 day to raise TSH secretion, there is no need to withhold LT4 therapy on the day of blood testing for TSH (8).
TSH Reference Intervals
Setting a reference interval for TSH is critical for diagnosing mild (subclinical) hypo- or hyperthyroidism. The current clinical guidelines recommend that TSH reference intervals should be established from the 95% confidence limits of the log-transformed TSH concentrations of at least 120 rigorously screened normal euthyroid volunteers who have (a) no detectable thyroid peroxidase (TPO) or Tg autoantibodies measured by sensitive immunoassay, (b) no personal or family history of thyroid dysfunction, (c) no visible or palpable goiter, and (d) who are taking no medications except estrogen (8).
TSH Upper Reference Limits (97.5th Percentile)
Multiple factors influence the determination of the TSH upper reference limit for a population. These include demographics such as sex, age, ethnicity, iodine intake, body mass index (BMI), smoking status and the exclusion of subclinical autoimmune thyroid disease (AITD). Over the last two decades, the upper reference limit for TSH has steadily declined from ∼10 mIU/L to ∼2.5 to 4.0 mIU/L. This decrease reflects a number of factors including the improved sensitivity and specificity of current monoclonal antibody-based immunometric TSH assays, the elimination of high values resulting from gonadotropin cross-reactivity, and the exclusion of individuals with subclinical AITD who have higher TSH values (8,41). Some guidelines propose the adoption of an empiric upper limit of 2.5 to 3.0 mIU/L, which is in accordance with the TSH interval associated with the lowest prevalence of anti-thyroid antibodies (8,42). However, this remains controversial, since the majority of individuals with serum TSH levels between 2.5 and 4.0 mIU/L have no evidence of thyroid disease serologically or on ultrasonography (43,44,45). The 97.5th percentile for serum TSH has been shown to be higher in groups of elderly or white individuals, women, and those with higher iodine intake than in other population subgroups, suggesting specific TSH reference limits, which are not currently available, should be considered for specific populations (43,46,47,48).
The importance of using age-specific TSH reference intervals for diagnosing thyroid dysfunction is illustrated in the young and old. In newborn, serum TSH concentrations increase from 6 mIU/L at birth to about 60 to 80 mIU/L in the first 30 minutes post-delivery (49). This “TSH surge” results in a serum T4 increase 24 hours following delivery, decreasing within 48 hours. TSH concentrations will decrease to approximately 20 mIU/L by the second day and continue to decrease and reach 6 to 10 mIU/L by the seventh day (50). TSH concentrations will gradually decline until the adult range is reached after puberty (51,52).
Epidemiological studies such as the US-based National Health and Nutrition Examination Survey (NHANES) illustrate that TSH distribution and reference limits shift to higher concentrations with age, even up to centenarians, and are unique for different racial/ethnic groups, being at higher concentrations in whites than either Blacks or Hispanics. The distribution curve derived by the traditional approach represents a composite of curves from specific subpopulations that do not provide appropriate reference limits for those unique groups (43). However, other more controlled studies claim that serum TSH concentrations decrease in healthy elderly subjects due to an age-related decrease in TSH secretion by the pituitary (53). Furthermore, the nocturnal TSH peak is blunted and there is a 1- to 1.5-hour shift in the circadian rhythm of TSH secretion, resulting in an earlier peak (54,55). The mechanism of this reduction in TSH secretion is currently unknown. It may very well be an increased sensitivity of the thyrotrophs to the negative feedback by T4, but other mechanisms such as a reduced hypothalamic TRH secretion cannot be excluded (8). Although reduced TSH levels result in a reduced thyroidal T4 secretion in the elderly (56,57), serum TT4 and FT4 concentrations remain unchanged (8,58). This is because T4 degradation by outer ring deiodination decreases with age resulting in age-dependent decline in TT3 and FT3 levels and an increase in the biologically inactive serum reverse T3 (rT3) levels. It is currently unknown whether an increased inner ring deiodination by deiodinase 3, which would result in an increased clearance of T4 and T3 and an increased production of rT3, also contributes to these changes. This has recently been shown to be a major factor in nonthyroidal illness (59,60).
TSH Lower Reference Limits
Using current third-generation IMA methodology, the lower TSH reference limit (2.5th percentile) is approximately 0.3 to 0.4 mIU/L, irrespective of the population studied or the method used (61). TSH in the 0.1 to 0.4 mIU/L range may represent mild thyroid hormone excess, and in elderly patients might be associated with an increased risk of atrial fibrillation and cardiovascular mortality (62). It is also important to note a shift in TSH distribution in Blacks (43), leading to possible over-diagnosis of subclinical hyperthyroidism. It is therefore important to carefully evaluate patients with TSH levels in this low range for underlying thyroid disease.
TSH and Thyroid Function Assessment in Pregnancy
Normal thyroid function is important for optimal maternal health and fetal neurodevelopment. Moreover, disorders of the thyroid gland are relatively frequent in women of childbearing age (63,64); therefore, TSH measurements are useful in detecting subtle thyroid dysfunction associated with poor pregnancy outcome. During pregnancy, the placenta secretes high levels of hCG, a glycoprotein hormone sharing a common alpha subunit with TSH (65). Immunometric TSH assays have overcome the problems posed by hCG cross-reactivity (64). Moreover, enhanced sensitivity of third-generation assays has established the lower TSH limit for nonpregnant women as ∼0.3 mIU/L and allows for the accurate detection of the low-serum TSH values often seen during the first trimester (66). However, in the case of maternal hypothyroxinemia (low FT4 with normal TSH), which is increasingly recognized for its association with neurodevelopmental deficits, TSH is within the normal range and is therefore not useful in detecting this problem (67).
TSH Trimester-Specific Reference Intervals
During pregnancy the lower and upper reference limits for serum TSH are decreased by about 0.1 to 0.2 mIU/L and 1.0 mIU/L respectively, compared to the TSH reference interval of 0.4 to 4.0 mIU/L of nonpregnant women (65,68). The Endocrine Society and the recent American Thyroid Association (ATA) guidelines recommend using a TSH upper limit of 2.5 mIU/L for preconception and first trimester, and 3.0 mIU/L for the second and third trimesters (37,69). Since hCG concentrations are higher in multiple pregnancies than in singleton pregnancies, a greater downward shift is observed in twin pregnancies (70). Of note, in 1.7% of pregnancies, TSH can be greatly suppressed (<0.01 mIU/L) and yet still represent a euthyroid pregnant patient (71). The differences in normal TSH intervals in the various ethnic groups persist during pregnancy (72); therefore, providing specific normal ranges for the local population is of particular advantage. Taken together, and in accordance with the new ATA guidelines for women in pregnancy, trimester-specific reference intervals for TSH, as defined in populations with optimal iodine intake, should be applied, although they are not generally provided (37). If trimester-specific reference ranges for TSH are not available in the laboratory, the following reference intervals are recommended by the ATA: First trimester 0.1 to 2.5 mIU/L; second trimester 0.2 to 3.0 mIU/L; third trimester 0.3 to 3.0 mIU/L (37,73) (see Chapter 56).
Hospitalized Patients
Nonthyroidal illnesses can frequently alter thyroid hormone peripheral metabolism and hypothalamic–pituitary–thyroidal (HPT) function resulting in thyroid test abnormalities, including both decreased and increased serum TSH levels (74). It is important to distinguish the generally mild, transient TSH alterations typical of NTI from the more profound and persistent TSH changes associated with hyper- or hypothyroidism (75) (see Chapter 11C).
Research studies report that within-person (intra-individual) TSH variability is relatively narrow, and varies by only 0.5 mIU/L when tested every month over a span of 1 year. In comparison, between-person variability is more variable (2,76,77). This somewhat limits the usefulness of population-based reference intervals to detect thyroid dysfunction in individuals (11,78). Theoretically, it may be important to evaluate individuals with marginally (yet confirmed) low (e.g., 0.3 to 0.4 mIU/L) or high (3.0 to 4.5 mIU/L) TSH levels relative to patient-specific risk factors for cardiovascular disease, rather than relative to the normal TSH reference interval (79). However, there are no data to show increased morbidity and mortality in individuals with serum TSH levels that are not within a person’s individual range but still fall within the population reference interval.
TSH Isoforms
Serum TSH analysis alone may fail to detect the presence of pituitary and/or hypothalamic disease such as central hypothyroidism or TSH-secreting pituitary tumors (TSHomas), since TSH levels are often normal in these circumstances (80,81). Furthermore, current TSH assays cannot distinguish between normal and biologically altered TSH isoforms. TSH isoforms with impaired biologic activity are typically secreted in central hypothyroidism, whereas TSH isoforms with enhanced biologic activity are often secreted by TSHomas (80,81). These abnormal TSH isoforms can result in paradoxically low, normal, or high TSH levels being reported in the face of clinical and biochemical hypo- or hyperthyroidism (81). Therefore, when suspecting pituitary or hypothalamic disease, it is important to measure serum FT4 and TSH concentrations concomitantly.
Table 13A.1 Causes of Low-Serum Tsh Concentrations | ||||||||||||||||||||||||||||||||||
---|---|---|---|---|---|---|---|---|---|---|---|---|---|---|---|---|---|---|---|---|---|---|---|---|---|---|---|---|---|---|---|---|---|---|
|
Total Thyroid Hormone Measurements (TT4 and TT3)
T4 is the principal hormone secreted by the thyroid gland. Circulating T4 is ∼99.98% bound to binding proteins, mainly TBG (60% to 75%), transthyretin (TTR, prealbumin) (15% to 30%), or albumin (∼10%) (82). In contrast, only about 20% of circulating T3 is synthesized by the thyroid while most of T3 is formed enzymatically in nonthyroidal tissues by 5′-monodeiodination of T4 (83). Approximately 99.7% of circulating T3 is bound to plasma proteins, primarily TBG (84) (see Chapter 6).
Since most thyroid diseases require prolonged periods of treatment, it is crucial to obtain an accurate diagnosis prior to treatment. Recent advancements in laboratory TFTs provide greater choice, sensitivity, and specificity, thus improving the likelihood of an early detection of occult thyroid diseases and
nonthyroidal diseases. Better assessment tools are also helpful in excluding a diagnosis of thyroid dysfunction when symptoms and signs closely mimic a thyroid ailment. Moreover, the availability of a wide choice of complementary and overlapping laboratory tests suggests that the procedures have their limitations and no single test has proven to be consistently reliable (85,86).
nonthyroidal diseases. Better assessment tools are also helpful in excluding a diagnosis of thyroid dysfunction when symptoms and signs closely mimic a thyroid ailment. Moreover, the availability of a wide choice of complementary and overlapping laboratory tests suggests that the procedures have their limitations and no single test has proven to be consistently reliable (85,86).
Serum TT4 and TT3 Assay Methodology
Total thyroid hormones, TT4 and TT3, are the free hormones bound to their carrier proteins; they are therefore found in nanomolar concentrations while FT4 and FT3 are present in the picomolar range. It is easier to measure total hormones relative to the free hormones, since FT4 and FT3 need to be displaced from their binding proteins prior to measurement (using various chemical agents or other separation techniques). The technical problems associated with serum TT4 and TT3 measurements are limited to assay interference and calibration issues. Other issues of concern are the purity of the standards, type of matrix (serum) used to prepare the calibrators, and the lack of an international reference method (8,86). To prevent matrix discrepancies between manufacturer reagents and patient specimens laboratories should routinely include serum-based QC materials in every run.
Most clinical laboratories routinely measure TT4 and TT3 by automated RIA kits or chemiluminescent enzyme immunoassay. RIA or IA approaches have high sensitivity but can sometimes lack specificity for thyroid hormones due to either endogenous factors such as abnormal binding proteins, dialyzable protein-binding competitors, HAbs, autoantibodies, or in vitro factors (free fatty acids, assay antibodies, analogs, intrinsic dilution) (86).
Clinical Utility of TT4 and TT3 Measurements
In clinical practice, problems associated with TT4 and TT3 concentrations occur mostly in patients with abnormalities in hormone-binding proteins that may, in turn, increase or decrease total hormone concentrations (84). The diagnostic accuracy of total hormone measurements would be proportional to that of free hormone tests if all patients had similar binding protein concentrations. Unfortunately, many conditions commonly encountered in clinical practice are associated with serum TBG abnormalities that distort the proportionality of the total to free thyroid hormone ratio. Furthermore, some patients have abnormal thyroid hormone–binding proteins, thyroid hormone autoantibodies, or are taking drugs that render total hormone measurements diagnostically unreliable. In patients with NTI, TBG may have lower affinity for T4 and T3 than normal TBG. In addition, many drugs may lower serum T4–binding capacity and some patients produce autoantibodies to T4 or T3, which increase hormone-binding capacity and interfere with total thyroid hormone assays (85).
The use of serum TT4 and TT3 assays is becoming less popular with the advent of more reliable methods for the measurements of serum FT4 and FT3. However, if TT4 and TT3 assays are employed, they are rarely used as stand-alone tests but are typically used in conjunction with an estimate of binding proteins [i.e., a thyroid hormone–binding ratio (THBR) test] and reported as a calculated free hormone index. TT4 measurements are considered to have a role in assessing thyroid status in some patients with NTI in whom serum FT4 and TSH values do not concur (Table 13A.1) (8) and in pregnancy, where FT4 values may not be representative of the patient’s true hormonal status (87).
TT4 and TT3 Reference Intervals
TT4 reference intervals range approximately from 4.5 to 12.5 μg/dL (58 to 160 nmol/L) quite consistently, although they may vary to some extent depending on the methods employed for their analysis. During pregnancy, TT4 concentrations are approximately the nonpregnant reference range adjusted by a factor of 1.5 to compensate for the predictable TBG elevation of about 50%. For a more detailed discussion of thyroid hormone measurements during pregnancy, see above. As with TT4, serum TT3 values are method-dependent to some extent with reference intervals approximating 80 to 180 ng/dL (1.2 to 2.7 nmol/L).
Free Thyroid Hormone Tests (FT4 and FT3)
Free Thyroxine (FT4) and Free Triiodothyronine (FT3)
In accordance with the free hormone hypothesis, it is believed that the free hormone fraction in the circulation (0.03% for FT4
and 0.3% for FT3) is responsible for biologic activity at the cellular level. FT4 and FT3 measurement is particularly challenging as the assay must detect very low levels of “free” hormone relative to a vast excess of protein-bound analyte (>99.5%). The relatively small size of T4 and of T3 precludes the use of a “sandwich” assay format, thus “competition assays” are commonly used, where labeled T4 (the tracer) competes with serum T4 for a fixed number of anti-T4 antibody-binding sites.
and 0.3% for FT3) is responsible for biologic activity at the cellular level. FT4 and FT3 measurement is particularly challenging as the assay must detect very low levels of “free” hormone relative to a vast excess of protein-bound analyte (>99.5%). The relatively small size of T4 and of T3 precludes the use of a “sandwich” assay format, thus “competition assays” are commonly used, where labeled T4 (the tracer) competes with serum T4 for a fixed number of anti-T4 antibody-binding sites.
FT4 and FT3 Assay Methodology
Free thyroid hormone assays are designed such that the equilibrium between T4 and its binding proteins is conserved during measurement, and, consequently, the amount of tracer displaced reflects the “free” rather than “total” hormone concentration. Clearly, the presence of serum factors affecting this equilibrium will confound hormone assays. For example, both fractionated and unfractionated heparin can cause an artefactual elevation in the measurements of FT4/FT3 by displacement of T4 and T3 from their carrier proteins (88). The mechanism is poorly understood, but likely involves the generation of nonesterified free fatty acids (NEFAs) via heparin-mediated activation of endothelial lipoprotein lipase (LPL), with NEFAs displacing FT4 from TT4 (89). The extent to which NEFAs rise is variable, and as displacement continues in vitro, pre-analytical delay can compound free hormone elevation (Fig. 13A.2). Thyroid function testing prior to administration of heparin, or the measurement of TT4 rather than FT4 (as the artifact is secondary to hormone displacement), can circumvent the problem. Furthermore, other factors, such as circulating thyroid hormone autoantibodies (that can bind to hormone tracers) and HAbs (especially HAMAs and rheumatoid factors), can cause interferences by immunoglobulin aggregation and (or) cross-linking of both capture and signal antibodies (85). Variant thyroid hormone–binding proteins [e.g., albumin in familial dysalbuminemic hyperthyroxinemia (FDH)] with altered affinity for T4 can also interfere with the assay (90).
The use of a “two-step” (back titration) assay method, with a wash step prior to tracer addition, may reduce but not completely eliminate such interferences. If erroneous results are suspected with a “one-step” assay, then re-measurement using a “two-step” assay would be a logical next step (Fig. 13A.3). If the problem persists, hormone measurements following equilibrium dialysis or ultrafiltration remain the recommended procedure for eliminating FT4 assay interference (91). When these are not available, the measurement of total hormone levels with estimation of T4/T3 binding capacity is advocated by some as an alternative (85).
Free hormone methods fall into two categories: Direct methods that employ a physical separation of the free from protein-bound hormone and estimate tests that either (a) calculate a free hormone index from a TBG estimate and measurement of total hormone or (b) use an immunoassay that employs an antibody to sequester a small amount of the total hormone that is proportional to the free hormone concentration. It is important to recognize that no free hormone test is universally valid in all clinical situations. Index tests (FT4I and FT3I) and FT4 and FT3 immunoassays are protein dependent to some extent and most are prone to under- or overestimate FT4 in patients with significant abnormalities in thyroid hormone–binding proteins (90,92,93) (Fig. 13A.4).
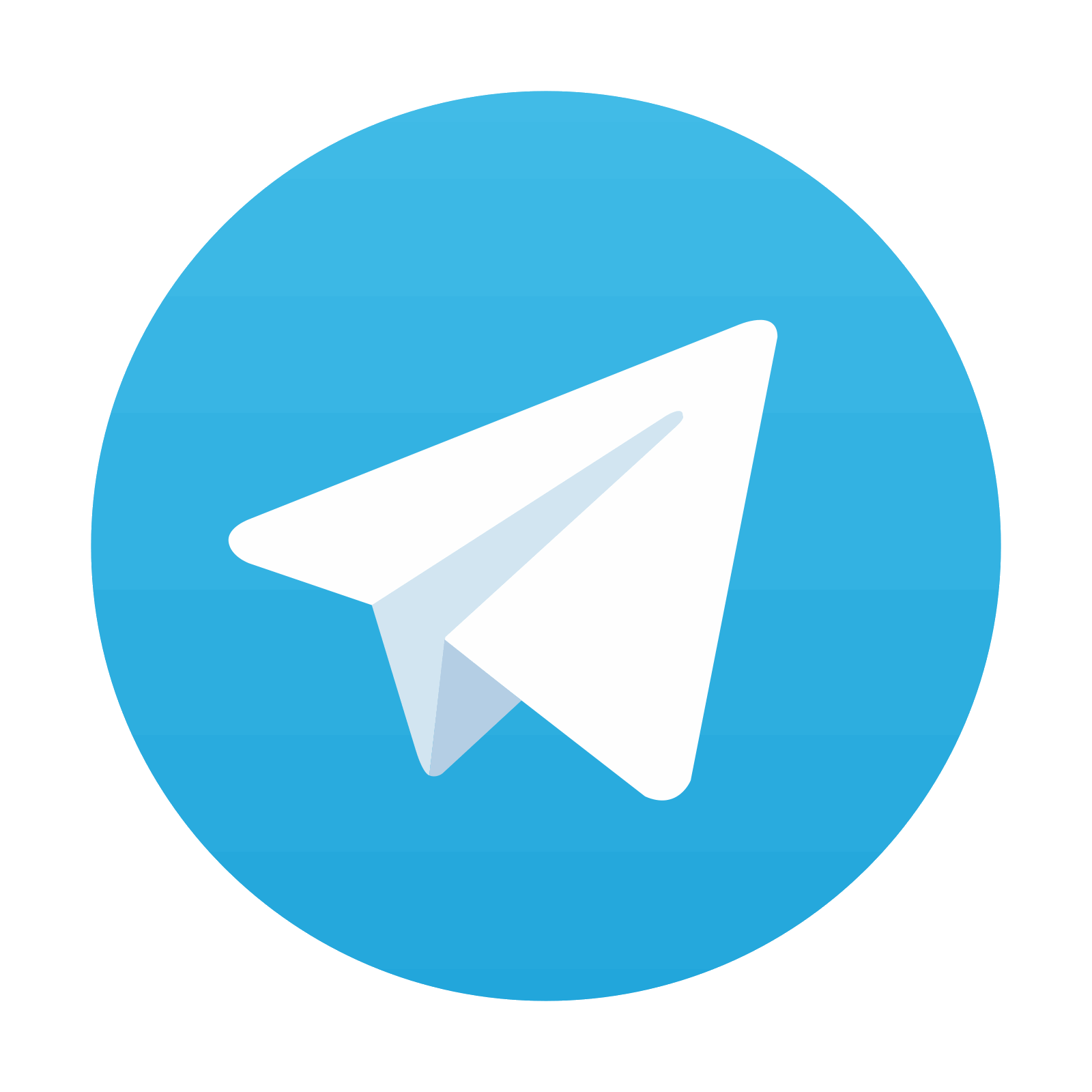
Stay updated, free articles. Join our Telegram channel
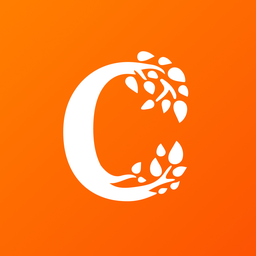
Full access? Get Clinical Tree
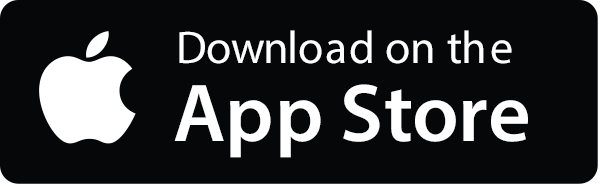
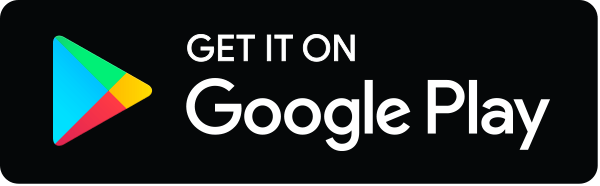