Fig. 7.1
Early pregnancy events in sheep. This schematic summarizes the relative changes in embryo/blastocyst/conceptus development after fertilization in relation to position in the female reproductive tract and circulating levels of ovarian steroid hormones. Fertilization occurs in the oviduct, and the morula-stage embryo enters the uterus on day 4. The blastocyst is formed by day 7, and it hatches from the zona pellucida by day 9. The blastocyst develops from a spherical to a tubular form by days 12 to 13 and then elongates to a filamentous conceptus between days 13 and 19. Elongation of the conceptus marks the beginning of implantation, which involves apposition and transient attachment (days 12 to 16) and firm adhesion by day 16 and is concomitant with the synthesis and secretions of interferon tau (IFNT) and prostaglandins (PG) by the trophectoderm. E2 estrogen, P4 progesterone
7.2.1 Sheep
The morula (16–32 cells)-stage embryo enters the uterus from the oviduct on day 4 after mating (day 0 = estrus/mating) (Fig. 7.1). The blastocyst is formed on day 6, and the zona pellucida is shed between days 8 and 9. The zona pellucida is thought to prevent the trophoblast from contacting and attaching to the endometrial LE. The blastocyst is spherical on day 8, measures 200 μm in diameter, and contains approximately 300 cells. By day 10, it measures 400–900 μm in diameter and contains approximately 3000 cells. After day 10, the growth of the blastocyst begins, and it is now termed a conceptus that develops first into an ovoid or tubular and then a filamentous conceptus (Wintenberger-Torres and Flechon 1974).
Between days 9 and 14, no definitive cellular contacts are observed between the trophectoderm and endometrial LE, and the blastocyst can be easily recovered from the uterus by lavage without causing structural damage. Starting on day 12, the spherical or slightly tubular conceptus begins to elongate until it reaches a length of 25 cm or more by day 17 and resembles a long filament composed mainly of extraembryonic trophoblast. By day 13, it reaches a length of 10–22 mm (1–2.2 cm), whereas by day 14, it has elongated markedly and is about 10 cm long. The primitive streak appears at this stage and somites develop soon thereafter. The conceptus, first located in the uterine horn ipsilateral to the CL, elongates into the contralateral horn and may fill more than half of its length on day 17 when only one ovulation has occurred (Rowson and Moor 1966).
Apposition of the conceptus involves the trophectoderm becoming closely associated with the endometrial LE followed by unstable adhesion. After day 14, the filamentous conceptus appears to be immobilized in the uterine lumen, and the trophectoderm maintains close contact with the endometrial LE (King et al. 1982; Guillomot et al. 1993). A close association of the apical membranes of both cell types is observed, although the conceptus can still be recovered intact from the uterus by lavage. Apposition of the blastocyst is ensured by interdigitation of cytoplasmic projections of the trophectoderm cells and uterine epithelial microvilli (Guillomot et al. 1981). In ruminants, the openings of uterine glands are also sites of apposition (Guillomot and Guay 1982; Guillomot et al. 1993). Between the caruncles, the trophoblast develops fingerlike villi or papillae, which penetrate into the mouths of the superficial ducts of the uterine glands at days 15–18 (Guillomot et al. 1981; Wooding et al. 1982). During their short life (they disappear by day 20), these trophoblastic differentiations are hypothesized to anchor the periattachment conceptus and absorb histotrophic secretions of the glands (Guillomot et al. 1981). Similar features were described for the cow conceptus from day 15 of pregnancy, but, curiously, the goat conceptus lacks trophoblast papillae.
On day 16, the trophoblast begins to adhere firmly to the endometrial LE. Uterine lavage to recover the conceptus causes superficial structural damage at this time. The interdigitation of the trophectoderm and endometrial LE occurs in both the caruncular and intercaruncular areas of the endometrium. Adhesion of the trophectoderm to the endometrial LE progresses along the uterine horn and appears to be completed around day 22 (Boshier 1969; Guillomot et al. 1981). Coincident with apposition and adhesion of mononuclear cells of the trophectoderm to the LE, trophoblast giant binucleate cells (BNC) begin to differentiate by day 16 within the trophectoderm (Wooding 1984) from mononucleate stem cells (Wooding 1992). Migration of BNC to the microvillar junction and then fusion with individual LE cells produce trinucleate fetomaternal hybrid cells (Wooding 1984). Continued BNC migration and fusion with trinucleate cells, together with displacement and/or death of the remaining uterine LE, apparently produce multinucleated syncytial plaques, linked by tight junctions and limited in size to 20–25 nuclei that cover the caruncles (Wooding 1982, 1984, 1992). The syncytial plaques and BNC form specialized structures on the placenta termed cotyledons that interdigitate with the endometrial caruncles of the maternal uterus to form a structure termed a placentome (Igwebuike 2006). Blood flow to the uterus and from the fetus is predominantly routed to the placentomes during later pregnancy, which provides hemotrophic nutrition from the mother to the fetus.
7.2.2 Cattle
Blastocyst growth and conceptus elongation are very similar in cattle and sheep (King et al. 1982), with the major difference that the elongation of the conceptus is initiated later and takes more time. The morula-stage embryo enters the uterus on days 4–6 post-mating and then forms a blastocyst that contains an inner cell mass and a blastocoele or central cavity surrounded by a monolayer of trophectoderm. After hatching from the zona pellucida (days 9–10), the blastocyst slowly grows into a tubular or ovoid form and is then termed a conceptus (Guillomot 1995; Hue et al. 2012). In cattle, the hatched blastocyst forms an ovoid conceptus between days 12–14 and is only about 2 mm in length on day 13. By day 14, the conceptus is about 6 mm and reaches a length of about 60 mm (6 cm) by day 16. It is 20 cm or more in length by day 19. Thus, the bovine blastocyst/conceptus doubles in length every day between days 9 and 16 with a significant increase (~10-fold) in growth between days 12 and 15 (Betteridge et al. 1980; Berg et al. 2010). After day 19 in cattle, the elongating conceptus is adhered to the LE and starts the process of placentation (Guillomot et al. 1981). Many aspects of placentation are similar in cattle and sheep, although some differences have been noted in placentome morphology and cellular architecture (Wooding and Wathes 1980; King and Atkinson 1987; Wooding 1992).
7.3 Maternal Recognition of Pregnancy
Maternal recognition of pregnancy in ruminants (sheep, cattle, goats) requires that the conceptus elongate and produce IFNT, which is the pregnancy recognition signal (see Spencer et al. 1996b; Roberts et al. 1999, 2008; Spencer and Bazer 2002, for review). The antiluteolytic effects of IFNT result in the maintenance of the CL and, hence, secretion of progesterone that is essential to maintain a uterine environment that supports events critical to the successful development of the conceptus to term.
7.3.1 Luteolytic Mechanism
Domestic ruminants are spontaneous ovulators that undergo uterine-dependent estrous cycles until the establishment of pregnancy (Wathes and Lamming 1995; McCracken et al. 1999; Spencer and Bazer 2002). The estrous cycle is dependent on the uterus, because it is the source of the luteolysin, prostaglandin F2 alpha (PGF2α). During the estrous cycle, the endometrium releases oxytocin-induced luteolytic pulses of PGF2α that result in functional and structural regression of the ovarian CL, termed luteolysis. In sheep, the source of luteolytic PGF2α pulses is the endometrial LE and superficial ductal glandular epithelium (sGE) (Gray et al. 2000a), because they express the oxytocin receptors (OXTR) (Wathes and Lamming 1995) and prostaglandin-endoperoxide synthase 2 (PTGS2), a rate-limiting enzyme in the synthesis of prostaglandins (Charpigny et al. 1997b; Simmons et al. 2010).
As illustrated in Fig. 7.2, the luteolytic mechanism that develops in the endometrial LE and superficial GE (sGE) involves sequential effects of progesterone, estrogen, and oxytocin, acting through their respective receptors (McCracken et al. 1984; Spencer et al. 1996b; Spencer and Bazer 2002). At estrus (day 0), estrogens from the antral follicle(s) increase uterine estrogen receptor alpha (ESR1), progesterone receptor (PGR), and OXTR expression (Wathes and Hamon 1993; Spencer and Bazer 1995); however, PGF2α is not secreted, because OXT is not present due to the absence of a CL. During early diestrus, progesterone from the newly formed CL stimulates the accumulation of phospholipids in LE/sGE that can liberate arachidonic acid for the synthesis and secretion of PGF2α. Progesterone levels increase and act via PGR to “block” expression of ESR1 and OXTR in the endometrial LE and sGE (McCracken et al. 1984). During most of diestrus, the expression of ESR1 and OXTR is not detected between days 5 and 11 of the cycle. The promoter of the ovine OXTR gene contains several SP1 elements that appear to mediate responsiveness to ligand-activated ESR1 (Fleming et al. 2006). Continuous exposure of the uterus to progesterone for 8–10 days downregulates the expression of PGR in endometrial LE/sGE after days 11–12 (Spencer et al. 1995b), allowing for rapid increases in the expression of ESR1 on days 12 and 13 followed by OXTR on day 14 (Hixon and Flint 1987; Spencer et al. 1995a). PTGS2 is also upregulated between days 10 and 12 post-estrus/mating (Charpigny et al. 1997b; Simmons et al. 2010). Oxytocin, secreted from day 9 of the estrous cycle and pregnancy from the posterior pituitary and/or CL, then induces the release of luteolytic PGF2α pulses between days 14 and 16 (Wathes and Lamming 1995). The CL undergoes regression, allowing the ewe to return to estrus and complete the 17-day estrous cycle. Thus, progesterone is paradoxically involved first in suppressing and then inducing the development of the endometrial luteolytic mechanism in cyclic ewes. The timing of the PGR downregulation by progesterone appears to determine when the luteolytic mechanism develops in the endometrium. This hypothesis is supported by the finding that exogenous progesterone administration during metestrus decreased the interestrus interval in sheep and cattle (Woody et al. 1967; Garrett et al. 1988a) and that treatment of cyclic sheep with RU486, a PGR antagonist, during the early luteal phase extended the interestrus interval (Morgan et al. 1993).
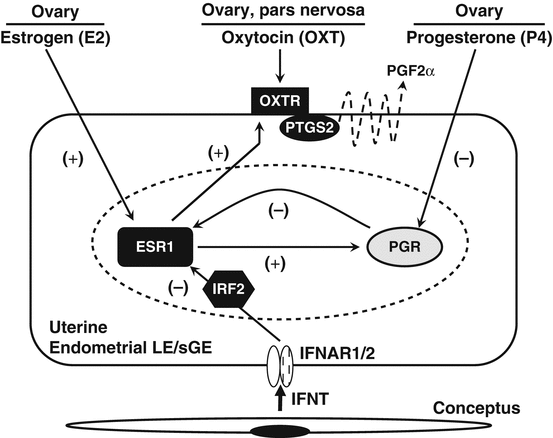
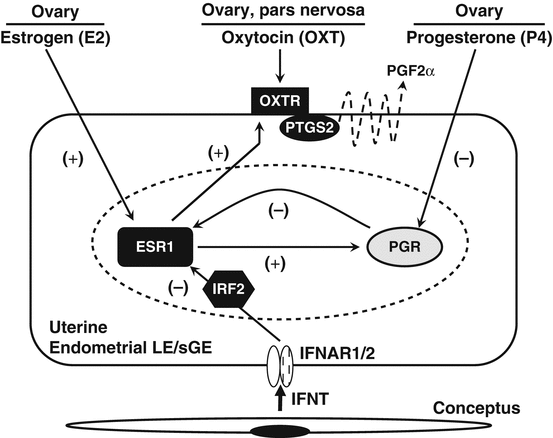
Fig. 7.2
Schematic illustrating hormonal regulation of the endometrial luteolytic mechanism and antiluteolytic effects of the conceptus on the ovine uterine endometrium. During estrus and metestrus, the expression of oxytocin receptors (OXTR) by uterine luminal and superficial ductal glandular epithelia (LE/sGE) increases in response to estrogens from the ovarian follicles that first stimulate the expression of estrogen receptor alpha (ESR1) and estrogens act via ESR1 to increase OXTR. Progesterone receptors (PGR) are expressed by LE/sGE during metestrus and diestrus, but low systemic levels of progesterone are insufficient to act via PGR to suppress ESR1 and OXTR gene expression. During early diestrus, endometrial ESR1 and estrogen are low, but progesterone levels begin to increase with the formation of the corpus luteum (CL). Progesterone acts through the PGR to suppress ESR1 and OXTR synthesis for 8 to 10 days. Continuous exposure of the endometrium to progesterone eventually downregulates PGR gene expression in the endometrial LE/sGE by days 11 to 12 of the estrous cycle. The loss of PGR terminates the progesterone block to ESR1 and OXTR formation. Thus, ESR1 appears between days 11 and 12 post-estrus, which is closely followed by increases in OXTR on days 13 and 14. The increase in OXTR expression is facilitated by increasing secretion of estrogens by ovarian follicles. In both cyclic and pregnant ewes, oxytocin is released from the posterior pituitary and ovarian corpus luteum beginning on day 9. In cyclic ewes, OXT binds to OXTR on LE/sGE and increases the release of luteolytic pulses of prostaglandin F2α (PGF2α) to regress the CL through a PTGS2-dependent pathway. In pregnant ewes, interferon tau (IFNT) is synthesized and secreted by the elongating conceptus beginning on day 10 of pregnancy. IFNT binds to type I IFN receptors (IFNAR) on the endometrial LE/sGE and inhibits transcription of the ESR1 gene through a signaling pathway involving interferon regulatory factor 2 (IRF2). These antiluteolytic actions of IFNT on the ESR1 gene prevent OXTR formation, thereby maintaining the CL and progesterone production required for the establishment and maintenance of pregnancy. E2 estradiol, ESR1 estrogen receptor alpha, IFNAR type I IFN receptor, IFNT interferon tau, IRF2 interferon regulatory factor 2, OXT oxytocin, OXTR oxytocin receptor, P4 progesterone, PGF prostaglandin F2α, PGR progesterone receptor, PTGS2 prostaglandin-endoperoxide synthase 2 (prostaglandin G/H synthase and cyclooxygenase)
7.3.2 Pregnancy Recognition
Embryo transfer experiments in sheep initially defined the period of maternal recognition of pregnancy by finding that the conceptus must be present in the uterus prior to the onset of luteolysis to extend CL lifespan (Moor et al. 1969). Moor and Rowson (Moor and Rowson 1966a; Moor and Rowson 1966b) found that a conceptus must be present in the uterus by days 12 or 13 of the cycle in order for a successful pregnancy to be obtained following embryo transfer. Removal of conceptuses from the uteri of ewes before day 13 of pregnancy had no effect on estrous cycle length, whereas removal after that time resulted in extension of CL lifespan past day 17 (Moor and Rowson 1964, 1966a; Moor et al. 1969). Thus, maternal recognition of pregnancy in the ewe occurs around days 12 and 13.
7.3.3 Discovery of Interferon Tau (IFNT)
Homogenates of day 14–15, but not day 21–25, conceptuses extended CL lifespan and the interestrous interval when infused into the uterus of cyclic ewes (Rowson and Moor 1967; Ellinwood et al. 1979; Martal et al. 1979), suggesting that the conceptus secreted an antiluteolytic protein that was produced for a limited amount of time before day 20. The antiluteolytic substance was heat and protease labile (Rowson and Moor 1967; Martal et al. 1979). Godkin et al. (1984b) subsequently demonstrated that intrauterine injections of conceptus secretory proteins from day 15–16 conceptuses would extend the interestrous interval when administered to cyclic ewes between days 12 and 14.
In order to identify the antiluteolytic protein(s), ovine conceptuses at different stages of development where cultured in the presence of radioactive amino acids and de novo synthesized proteins identified by two-dimensional polyacrylamide gel electrophoresis and fluorography (Godkin et al. 1982). In sheep, the major product synthesized and released was a protein of low molecular weight (17–20 kDa). Because it was the first major protein secreted by the trophoblast of the developing ovine conceptus, the protein was later designated as “ovine trophoblast protein one or oTP-1” (Godkin et al. 1984a). Synthesis of oTP-1 was not detectable by day 23 conceptuses, which correlated with the inability of conceptus homogenates from this day to extend the interestrous interval of cyclic ewes. Intrauterine injections of purified oTP-1 into the uterus of cyclic ewes, between days 12 and 14, extended the interestrous interval and maintained progesterone production by the CL (Godkin et al. 1984b). These studies suggested that oTP-1 was the sole antiluteolytic factor present in the total array of conceptus secretory proteins. In an elegant experiment, Vallet et al. (1988) demonstrated that oTP-1 was the sole antiluteolytic protein of those secreted by the trophoblast of the ovine conceptus.
Imakawa et al. (1987) and Stewart et al. (1987) identified oTP-1 as a member of the type I interferon alpha (IFNA) family of proteins based on protein and DNA sequencing technologies. Homology between the 172-amino-acid ovine oTP-1 and the 165-amino-acid bovine IFNA1 mRNA and protein is 63 % and 50 %, respectively (Roberts 1991; Roberts et al. 1991). However, homology with the 172-amino-acid bovine IFN omega 1 (IFNW1) mRNA and protein was 85 % and 72 %, respectively, suggesting that oTP-1 was a distinct subgroup of the type I IFN family (Imakawa et al. 1987). Because of the unique developmental expression of oTP-1 by the trophoblast and its relatedness to other type I IFNs (alpha, beta, omega), oTP-1 was classified as IFNT by the International Cytokine and Interferon Society (Roberts 1991). Cattle and sheep possess three copies of IFNT in their genomes (Hansen et al. 1991). Recent RNA-sequencing data found that two conceptus IFNT genes are expressed in the trophoblast of cattle (Sakurai et al. 2013b). It is now clear that the IFNT are unique to the ruminant ungulates, having diverged from ones encoding its closest relative, IFNW, about 36 million years ago at a time when the ruminant species themselves began to emerge as a separate lineage within the artiodactyl order (Roberts et al. 1997). It is tempting to assume that IFN production and its ability to trigger particular downstream signaling pathways in the endometrium enabled the superficial implantation and placentation of the pecoran ruminants to evolve successfully (Roberts et al. 2008).
7.3.4 Expression of IFNT
Immunocytochemical studies found that IFNT is confined to mononuclear cells of the trophectoderm (Godkin et al. 1984a; Guillomot et al. 1990). In situ hybridization analysis of conceptuses also localized IFNT mRNA exclusively to trophectoderm cells, and expression was not detected in the extraembryonic endoderm, yolk sac, allantois, or embryo proper (Farin et al. 1989; Guillomot et al. 1990). During maternal recognition of pregnancy, the mononuclear cells of the conceptus trophectoderm synthesize and secrete IFNT between days 10 and 21–25 with maximal production on days 14–16 (Bazer et al. 1992; Roberts et al. 1999). On day 15, ovine conceptuses release greater than 100 μg of the protein in culture in a 24-h period (Ashworth and Bazer 1989b). Concentrations of IFNT mRNA in the conceptus appear to peak around day 14 in sheep and day 20 in cattle (Hansen et al. 1988; Stewart et al. 1989). Ashworth and Bazer (Ashworth and Bazer 1989a) detected low amounts of IFNT as early as days 8 and 10 of pregnancy. In situ hybridization analyses of IFNT mRNA in ovine conceptuses confirmed the protein production results with mRNA detected as early as days 10 and 11 with maximum expression after day 13 and a decline after day 17 (Farin et al. 1989, 1990, 1991; Guillomot et al. 1990). The reduction in IFNT gene expression occurs after the conceptus has adhered to the epithelium during definitive placentation. Thus, IFNT is transiently produced by the conceptus, and the expression is highest prior to the formation of OXTR in the endometrial epithelium on days 13 to 14 in cyclic or nonpregnant ewes.
IFNT expression is unique in at least four respects when compared to other type I IFNs: It is confined to the ruminant ungulates, there is lack of viral inducibility, expression is restricted to the embryonic trophectoderm, and high-level synthesis is sustained over several days and then terminates (Roberts et al. 2008). The cellular and molecular mechanisms that regulate IFNT gene expression in the mononuclear trophectoderm are only partially understood (see Roberts et al. 2008). Elements that control tissue and temporal expression are in the 5’-flanking region of the intronless IFNT genes and are highly conserved across the ruminant species (Leaman et al. 1994). Interestingly, the arrest of IFNT gene expression occurs in regions of the mononuclear trophectoderm that have established cellular contacts with the LE during the implantation process (Guillomot et al. 1990). The molecular mechanism of IFNT gene silencing may involve transcriptional repressors, such as eomesodermin, that are upregulated in trophectoderm cells adhered to the LE that culminates in implantation (Sakurai et al. 2013a).
7.3.5 Antiluteolytic Effects of IFNT
The most unique biological effect of IFNT is its antiluteolytic activity in ruminants. Intrauterine injections of ovine IFNT into sheep (Godkin et al. 1984b; Vallet et al. 1988; Ott et al. 1993), as well as cattle (Knickerbocker et al. 1986a; Knickerbocker et al. 1986b; Thatcher et al. 1986; Meyer et al. 1995; Thatcher et al. 2001) and goats (Newton et al. 1996), abrogate the development of the endometrial luteolytic mechanism and extend CL lifespan and the interestrous interval. It must be noted that the same mechanism(s) involved in IFNT action in sheep may be slightly different from those in the cow (Thatcher et al. 1992; Hansen et al. 1999). Therefore, the discussion of detailed mechanisms of the antiluteolytic actions of IFNT on the endometrium is limited to sheep given that less is known about those aspects of IFNT action in cattle.
IFNT appears to be the sole factor produced by the conceptus that prevents the development of the endometrial luteolytic mechanism (Vallet et al. 1988). In sheep, IFNT does not act to stabilize PGR expression in the endometrial epithelium during pregnancy (Spencer and Bazer 1995, 1996; Spencer et al. 1995b). As illustrated in Fig. 7.2, IFNT acts in a paracrine fashion on endometrial LE/sGE to suppress the transcription of ESR1 and OXTR genes (Spencer and Bazer 1996; Spencer et al. 1996a; Fleming et al. 2001), thereby abrogating the development of the endometrial luteolytic mechanism. The increases in ESR1 and OXTR gene expression detected in the LE/sGE on days 11–17 post-estrus in cyclic sheep do not occur in pregnant sheep (Spencer and Bazer 1995) or in cyclic sheep infused with recombinant ovine IFNT (Spencer et al. 1995c). By inhibiting increases in OXTR expression, IFNT prevents endometrial production of luteolytic pulses of PGF2α. However, IFNT does not inhibit basal production of PGF2α, which is higher in pregnant than cyclic ewes, and the conceptus and IFNT do not affect PTGS2 expression in the endometrial LE/sGE (Charpigny et al. 1997b; Kim et al. 2003b; Simmons et al. 2010). Thus, the antiluteolytic actions of IFNT are to prevent increases in epithelial ESR1 and OXTR gene expression, which are estrogen responsive, by directly inhibiting transcription of the ESR1 gene. The precise cellular and molecular mechanisms involved in IFNT inhibitory actions on the ovine ESR1 gene are not fully known but involve IFN regulatory factor 2 (IRF2) (Fleming et al. 2001) (Fig. 7.3).
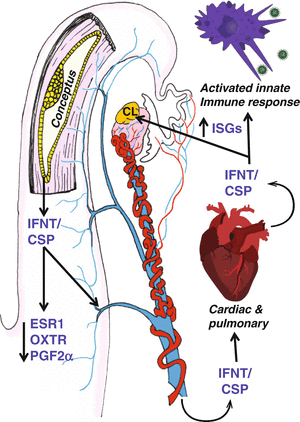
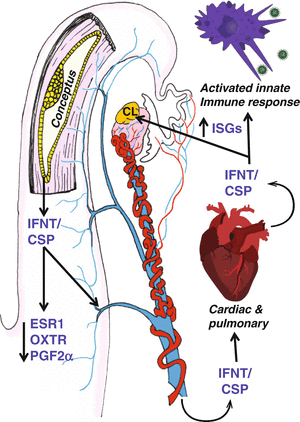
Fig. 7.3
Endocrine action of pregnancy in ruminants. IFNT is a major conceptus secretory protein (CSP) that is released by the expanding and elongating blastocyst. IFNT has been show to suppress the upregulation of ESR1, which leads to the suppression of OXTR, disruption of pulsatile PGF2α release, and antiluteolytic action on the corpus luteum. Because the bovine OXTR gene does not contain estrogen response elements, the mechanism disrupting PGF may be slightly different. Regardless, paracrine action of IFNT alters PGF2α pulses in both sheep and cattle and, thereby, protects the CL so that it can continue to produce progesterone, which supports the production of histotroph and further development and attachment of the conceptus. In addition to activating ISGs in the endometrium, IFNT, in addition to other CSP, may be released into the uterine vein to act in peripheral/endocrine action on immune cells and the corpus luteum. The consequences of activated innate immune responses during the establishment of pregnancy in ruminants are unknown and need to be clarified as functionally important or simply consequential to massive release of IFNT by the developing conceptus. Concerns with the utility of detection of ISGs in blood cells as indicators of pregnancy center on massive induction of these same ISGs in response to viral infections and other inflammatory responses (i.e., bacterial infections such as mastitis in dairy cows). However, the endocrine action of pregnancy and IFNT when inducing ISGs in the CL may be relevant to the establishment of luteal resistance to PGF2α. This is certainly implicated through studies demonstrating resistance of the CL induced by endocrine delivery of IFNT in response to both endogenous PGF2α and exogenous PGF2α
Unlike the promoter for the ovine OXTR gene, the bovine OXTR gene lacks a classical palindromic estrogen response element (Bathgate et al. 1998), and no change in ESR1 expression was observed in the uterine epithelia of pregnant as compared with nonpregnant cattle (Robinson et al. 1999). Thus, pregnancy and IFNT can apparently alter OXTR mRNA expression independent of ESR1 in the endometrium of cattle. Of note, IRF2 can regulate the expression of the bovine OXTR gene (Telgmann et al. 2003), suggesting a common role as an effector of IFNT antiluteolytic actions manifest on the endometrium to establish pregnancy.
7.3.6 Endocrine Actions of IFNT
For many years, IFNT was not thought to be released from the uterus and was believed to have only paracrine effects on the endometrium, because it was not detected in peripheral blood. However, ISG mRNAs were found to be upregulated in peripheral blood monuclear cells (PBMC) in response to pregnancy in both sheep (Yankey et al. 2001) and cattle (Han et al. 2006; Gifford et al. 2007). The impact of pregnancy on induction of ISGs in blood cells was intriguing, especially in light of opinion that IFNT was not released from the uterus into peripheral circulation. Exactly how PBMC became activated to express ISGs was unknown; however, 674 genes were upregulated and 721 genes were downregulated in PBMC from pregnant compared with nonpregnant cattle on day 18 (Hansen et al. 2010a). Importantly, many of the upregulated genes were ISGs, suggesting that IFNT exited from the uterus and had an endocrine effect on maternal tissues. Schalue-Francis et al. (Schalue-Francis et al. 1991) reported very low levels of antiviral activity in uterine vein blood of pregnant sheep. Next, significant antiviral activity was found in uterine vein blood from day 15 pregnant sheep (Oliveira et al. 2008). This antiviral activity was shown to be specifically induced by IFNT because preadsorption of IFNT using anti-IFNT antibody eliminated antiviral activity in uterine vein blood from day 15 pregnant sheep (Bott et al. 2010). Based on antiviral activity, the amount of IFN released from the uterus was estimated to be approximately 200 μg per 24 h. Further, uterine venous blood had 500- to 1000-fold higher concentrations of bioactive IFN than uterine arterial blood on day 15 of pregnancy. Thus, Bott and coworkers (Bott et al. 2010) concluded that IFNT exited the uterus in sheep and could be detected in uterine vein blood on day 15 of pregnancy. Indeed, IFNT has been identified in the uterine vein blood of early pregnant sheep by mass spectrometry as well as radioimmunoassay (T. R. Hansen, unpublished results).
7.3.6.1 Regulation of CL Function by IFNT
Moor and Rowson (Moor and Rowson 1966a) and Mapletoft and coworkers (Mapletoft et al. 1976b) described a local effect of the conceptus in maintaining the ipsilateral but not contralateral CL in ruminants. Those studies were interpreted to indicate that the conceptus does not have a systemic effect on the CL. However, the CL of pregnancy is much more resistant to lytic effects of PGF2α (Inskeep et al. 1975; Mapletoft et al. 1976a; Pratt et al. 1977; Silvia and Niswender 1984). Thus, the elongating conceptus could have endocrine effects on the CL during early pregnancy in sheep.
Pregnant and cyclic ewes have very different patterns of PGF2α release in the blood between days 12–16 post-ovulation (Thorburn et al. 1972; Zarco et al. 1988a). Cycling ewes released PGF2α in a pulsatile manner, while pregnant ewes lack the pulsatile pattern but have higher basal circulating concentrations (Peterson et al. 1976; Zarco et al. 1988b). Higher levels of PGF2α are found in the uterine vein of day 13 pregnant as compared to cyclic ewes (Wilson et al. 1972). Although IFNT clearly inhibits the uterine production and release of luteolytic pulses of PGF2α, PGF2α synthesis by the endometrium is not inhibited, and there is a possibility that the CL produces PGF2α (Silva et al. 2000). Thus, mechanisms inducing resistance of the CL to PGF2α may need to be activated during early pregnancy to prevent luteolysis.
As found in the PBMC, ISGs are upregulated in the CL in pregnant sheep and cattle (Oliveira et al. 2008; Bott et al. 2010; Yang et al. 2010). For example, ISG15 mRNA levels were much higher in CL from day 15 pregnant compared with nonpregnant ewes. Likewise, ISG15 protein and its ISGylated protein targets also were upregulated in CL in response to pregnancy, predominantly in large luteal cells on day 15 of pregnancy, with diminished but significant localization to small luteal cells. IFNT, but not PGE2, treatment of small, large, and mixed luteal cells from day 10 cyclic ewes induced ISG15 expression (Antoniazzi et al. 2013; Romero et al. 2013). Further, intrauterine injections of recombinant ovine IFNT (roIFNT) induced ISG expression in the CL of cyclic ewes (Spencer et al. 1999b). These studies strongly supported the idea that IFNT exited the uterus of early pregnant sheep and had an endocrine effect on the CL and many other maternal tissues.
In order to examine the potential endocrine actions of IFNT, osmotic pumps were implanted into day 10 cyclic ewes and 200 μg of roIFNT was infused into the uterine vein each day. ISG15 mRNA was upregulated in the ipsilateral and contralateral CL as well as in the endometrium and liver (Oliveira et al. 2008; Bott et al. 2010). When the uterine vein of cyclic ewes was infused with roIFNT from day 10 to day 17 post-estrus, the interestrous interval was extended to greater than 32 days, whereas cyclic ewes infused with bovine serum albumin returned to estrus by day 19. Thus, endocrine delivery of IFNT into the uterine vein for 7 days was able to block luteolysis from endogenously produced PGF2α. Further, Bott and coworkers (Bott et al. 2010) demonstrated that delivery of 200 μg of roIFNT into the uterine vein would protect the CL from the luteolytic actions of PGF2α. More recently, 24 h infusion of only 20 μg of roIFNT per day into the uterine vein or subcutaneously into the neck on days 10–11 of the estrous cycle was able to significantly protect the CL from the lytic action of PGF2α exogenously administered on day 11 (Antoniazzi et al. 2013). Collectively, these results strongly support the idea that resistance of the CL in pregnant sheep to luteolytic PGF2α is due to the endocrine actions of IFNT, perhaps by protecting the integrity and steroidogenic machinery and/or attenuating apoptosis in the CL of pregnancy (Hansen et al. 2010a; Antoniazzi et al. 2013; Romero et al. 2013).
The endocrine effects of IFNT on CL function are less established in cattle, but ISG mRNAs are upregulated in PBMC in response to pregnancy in cattle (Han et al. 2006; Gifford et al. 2007; Hansen et al. 2010a). Indeed, ISGs may be useful as an early pregnancy test in cattle (Han et al. 2006; Gifford et al. 2007; Green et al. 2010; Pugliesi et al. 2014). However, concerns with utility of detection of ISGs in blood cells as indicators of pregnancy center on massive induction of the same ISGs in response to viral infections and other inflammatory responses, i.e., bacterial infections such as mastitis in dairy cows (Hansen et al. 2010b; Smirnova et al. 2012).
7.3.7 IFNT Regulation of Endometrial Function and Conceptus Elongation
In addition to antiluteolytic effects on the endometrium, IFNT induces or enhances the expression of ISGs in the endometrium of both early pregnant sheep and cattle that are hypothesized to regulate uterine receptivity for conceptus elongation and implantation (Hansen et al. 1999, 2010a; Spencer et al. 2008; Bazer et al. 2009a). The actions of IFNT are mediated by the interferon (alpha and beta) receptor (IFNAR), which is composed of two subunits, IFNAR1 and IFNAR2 (Hansen et al. 1989). To test the hypothesis that IFNT and its receptor have biological roles in conceptus elongation, an in vivo loss of function study was recently conducted by inhibiting IFNT or IFNAR1/2 mRNA translation in the trophectoderm of the ovine conceptus using morpholino antisense oligonucleotides (MAO) delivered via osmotic pumps from days 8–14 post-mating (Brooks and Spencer 2014). Elongating, filamentous-type conceptuses were recovered from day 14 ewes receiving a control morpholino or IFNAR MAOs. In contrast, severely growth-retarded and malformed conceptuses were recovered from IFNT MAO-infused ewes. Those conceptuses contained abnormal trophectoderm cells that were apoptotic. Available studies support the idea that IFNT is a critical regulator of conceptus elongation and its effects are most likely indirectly mediated by IFNT-stimulated embryotrophic factors from the endometrium.
7.3.7.1 Classical Type I IFN-Stimulated Genes in the Endometrium
A number of transcriptional profiling and proteomic experiments conducted with human cells, ovine endometrium, bovine endometrium, and bovine peripheral blood lymphocytes have elucidated classical ISGs induced by IFNT during pregnancy (Hansen et al. 1999; Spencer et al. 2007a, 2008; Ott and Gifford 2010; Forde et al. 2011; Bauersachs et al. 2012). The development of a bovine endometrial cell line called BEND cells allowed study of signal transduction following treatment with IFNT in vitro. Using these cells, it was demonstrated that IRF1 and STAT1, STAT2, and STAT3 proteins were phosphorylated in response to IFNT (Perry et al. 1999; Thatcher et al. 2001). Also, specific binding of IRF1 to the bovine ISG15 gene promoter (ISRE) was described using shift and supershift transcription factor/promoter assays (Perry et al. 1999). The effects of IFNT in the bovine endometrium are not as well understood compared to ovine endometrium in terms of nonclassical ISGs and associated signal transduction, but recent studies have started to unravel those effects in cattle (Forde et al. 2011; 2012; Bauersachs et al. 2012).
In vivo studies revealed that the majority of classical ISGs are induced in the endometrial stroma and glands as well as the myometrium of the ovine uterus during early pregnancy (Johnson et al. 1999b, 2001; Choi et al. 2001, 2003; Song et al. 2007). The lack of classical ISG expression in the endometrial LE or sGE during pregnancy may be a critical mechanism preventing immune rejection of the semi-allogeneic conceptus (Choi et al. 2003). One challenge has been to determine which of the large number of classical ISGs induced in the endometrium by IFNT has a biological role in conceptus elongation and implantation, as traditionally the main function of type I IFN is to inhibit viral infection and has primarily been associated with cellular antiviral responses (Pestka 2007). It is likely that the classical ISGs induced by IFNT in the endometrium have biological roles in conceptus implantation and establishment of pregnancy by actions on the trophectoderm (Imakawa et al. 2006) or modulation of immune cells at the conceptus–maternal interface (Hansen 1995, 2007, 2013; Hansen et al. 1999).
7.3.7.2 Nonclassical IFNT-Stimulated Genes in the Endometrium
Transcriptional profiling of human U3A (STAT1-null) cells and ovine endometrium and candidate gene analyses were used to discover novel “nonclassical” ISG in the endometrial LE during pregnancy (Kim et al. 2003a; Song et al. 2005; Gray et al. 2006; Satterfield et al. 2006; Song et al. 2006). Subsequently, a series of transcriptomic and candidate gene studies found that IFNT stimulates the expression of a number of elongation- and implantation-related genes that are initially induced by progesterone specifically in the endometrial LE, sGE, and(or) GE (Spencer et al. 2007a, 2008; Bazer et al. 2009a, b). None of these genes are classical type I ISGs and thus can be referred to as “nonclassical or novel” ISG. Indeed, IFNT stimulation of these nonclassical ISG requires initial induction in the endometrial epithelia by progesterone. Importantly, all of the nonclassical ISGs encode factors whose actions on the trophectoderm (proliferation, migration, attachment and (or) adhesion, nutrient transport) would be or are important for conceptus elongation (see Spencer et al. 2004a, 2008; Bazer et al. 2011, 2012a; Dorniak et al. 2013, for review).
7.4 Functional Role of Endometrial Secretions in Implantation and Establishment of Pregnancy in Ruminants
All mammalian uteri contain endometrial epithelia that synthesize and secrete or transport a complex array of proteins and related substances termed “histotroph” (Wimsatt 1950; Amoroso 1952; Bazer 1975), that is, a complex mixture of enzymes, growth factors, cytokines, lymphokines, hormones, transport proteins, and other substances. Evidence from human, primate, and subprimate species during the last century supports an unequivocal role for secretions of endometrium as primary regulators of conceptus survival, development, production of pregnancy recognition signals, implantation, and placentation (reviewed in Bazer et al. 1979; Roberts and Bazer 1988; Gray et al. 2001a; Burton et al. 2002; Filant and Spencer 2014). The microvillous epithelial cells of the uterine lumen present a high secretory activity during the luteal phase of the cycle and at the beginning of implantation (Guillomot et al. 1981). The sheep trophoblast appears to be the site of intense pinocytotic activity that increases as the blastocyst develops and elongates (Wintenberger-Torres and Flechon 1974). Indeed, blastocyst growth into an elongated conceptus does not occur in vitro, as it requires secretions supplied by the endometrium of the uterus (Betteridge and Flechon 1988; Gray et al. 2001c; Lonergan 2011).
7.4.1 Uterine Gland Knockout (UGKO) Ewe Model
The UGKO ewe model is produced by continuous administration of a synthetic, nonmetabolizable progestin to neonatal ewes from birth to 8 weeks of age (Bartol et al. 1999; Gray et al. 2000b). This inappropriate exposure to a progestin permanently ablates the differentiation and development of the glandular epithelia (GE) from LE in the endometrium and produces an UGKO phenotype without altering the development of myometrium or other Müllerian duct-derived female reproductive tract structures or the hypothalamic–pituitary–ovarian axis (Gray et al. 2000b, 2001b). The endometrium is devoid of middle to deep endometrial glands, and the LE surface area is markedly reduced. UGKO ewes exhibit recurrent early pregnancy loss in which the blastocyst fails to elongate. Transfer of blastocysts from normal fertile ewes into the uteri of timed recipient UGKO ewes does not ameliorate this defect (Gray et al. 2001c). Morphologically normal blastocysts are present in uterine flushes of bred UGKO ewes on days 6 and 9 after mating, but not on day 14 (Gray et al. 2001c, 2002). On day 14, uterine flushes of mated UGKO ewes contain either no conceptus or a severely growth-retarded tubular conceptus. Therefore, histotrophic secretions from the endometrial epithelia, particularly the GE, are required for peri-implantation blastocyst survival and conceptus elongation in sheep.
Available results indicate that the defects in blastocyst survival and elongation in UGKO ewes are not due to alterations in the expression of steroid receptors, mucin glycoprotein 1 (MUC1), or adhesive integrins on the endometrial LE or to the responsiveness of the endometrium to the conceptus pregnancy recognition signal IFNT (Gray et al. 2001b, 2002). However, when uterine flushes of day 14 bred UGKO ewes were analyzed for the presence of osteopontin (OPN or secreted phosphoprotein 1) and glycosylated cell adhesion molecule 1 (GLYCAM1) proteins, which are adhesion proteins secreted primarily by GE (Johnson et al. 1999a; Spencer et al. 1999a), very low levels of OPN and GLYCAM1 were found in UGKO as compared to normal day 14 pregnant ewes (Gray et al. 2002). Therefore, the reduction or absence in adhesion proteins of endometrial epithelial origin was proposed to be a cause of recurrent pregnancy loss in the UGKO ewe. Given the complexity of uterine luminal fluid, undoubtedly a number of other factors are deficient in the UGKO uteri that act on the conceptus to stimulate trophoblast survival and proliferation.
7.4.2 Embryotrophic Factors in the Uterine Lumen Regulating Conceptus Elongation
The uterine luminal fluid contains histotroph that governs elongation of the conceptus via effects on trophectoderm proliferation and migration as well as attachment and adhesion to the endometrial LE (Spencer et al. 2007b, 2008; Bazer et al. 2010). Histotroph is derived primarily from transport and (or) synthesis and secretion of substances by the endometrial LE and GE, and it is a complex and rather undefined mixture of proteins, lipids, amino acids, sugars (glucose, fructose), ions, and exosomes/microvesicles (Bazer 1975; Gray et al. 2001a; Koch et al. 2010; Bazer et al. 2012b; Burns et al. 2014). The recurrent early pregnancy loss observed in uterine gland knockout (UGKO) ewes established the importance of uterine epithelial-derived histotroph for support of conceptus elongation and implantation (Gray et al. 2001c). Available evidence supports the idea that ovarian P4 induces the expression of a number of genes, specifically in the endometrial epithelia, that are then further stimulated by factors from the conceptus (e.g., IFNT, PGs, cortisol) as well as the endometrium (e.g., PGs and cortisol) (Dorniak et al. 2013; Brooks et al. 2014). The genes and encoded hormones, cytokines, and other functional mediators in the endometrial epithelia elicit specific changes in the intrauterine histotrophic milieu necessary for conceptus elongation (Spencer et al. 2007b, 2008; Bazer et al. 2010; Forde and Lonergan 2012; Dorniak et al. 2013). The outcome of the progesterone-induced changes in the uterus during the estrous cycle or pregnancy is to modify the intrauterine milieu, such as an increase in select amino acids, glucose, cytokines and growth factors, and adhesion proteins in histotroph, for support of blastocyst growth into an ovoid conceptus and its elongation to form a filamentous conceptus (see Spencer et al. 2008; Bazer et al. 2010; Forde and Lonergan 2012; Dorniak et al. 2013; Brooks et al. 2014). Factors from the endometrium may also stimulate the expression of IFNT in the conceptus trophectoderm (Roberts et al. 2003; Michael et al. 2006; Ealy and Yang 2009; Kim et al. 2011). Several recent reviews catalogue the endometrial contributions to uterine luminal fluid that functions in conceptus elongation in ruminants (Roberts et al. 2008; Spencer et al. 2008; Bazer et al. 2010; Forde and Lonergan 2012; Bauersachs and Wolf 2013; Dorniak et al. 2013; Ulbrich et al. 2013; Brooks et al. 2014; Lonergan and Forde 2014).
7.5 Prostaglandins and Conceptus Elongation
The conceptus and endometrium synthesize a variety of PGs during early pregnancy in both sheep and cattle (Lewis et al. 1982; Lewis and Waterman 1983, 1985; Lewis 1989; Charpigny et al. 1997a, b). The endometrium and uterine lumen contain substantially more PGs during early pregnancy than the estrous cycle (Ellinwood et al. 1979; Marcus 1981; Ulbrich et al. 2009). The dominant cyclooxygenase expressed in both the endometrium and trophectoderm of the elongating conceptus is PTGS2 (Charpigny et al. 1997a, b). Although the antiluteolytic effects of IFNT are to inhibit the expression of the OXTR in the endometrial LE/sGE of early pregnant ewes, it does not impede the upregulation of PTGS2, a rate-limiting enzyme in PG synthesis, in the endometrium (Charpigny et al. 1997b; Kim et al. 2003c; Simmons et al. 2010). In the bovine uterus, PTGS2 is also not downregulated in the endometria of early pregnant cattle, but rather is upregulated by IFNT (Arosh et al. 2004; Emond et al. 2004). Further, IFNT acts as a molecular switch that stimulates PGE2 production in the bovine endometrium (Krishnaswamy et al. 2009). In sheep, PTGS2 activity in the endometrium is stimulated by IFNT, and PTGS2-derived PGs were found to mediate, in part, the effects of progesterone and IFNT on the endometrium of the ovine uterus (Dorniak et al. 2011b, 2012). Indeed, type I IFNs were found to stimulate phospholipase A2 activity and synthesis of PGE2 and PGF2α in several different cell types over 25 years ago (Fitzpatrick and Stringfellow 1980; Fuse et al. 1982).
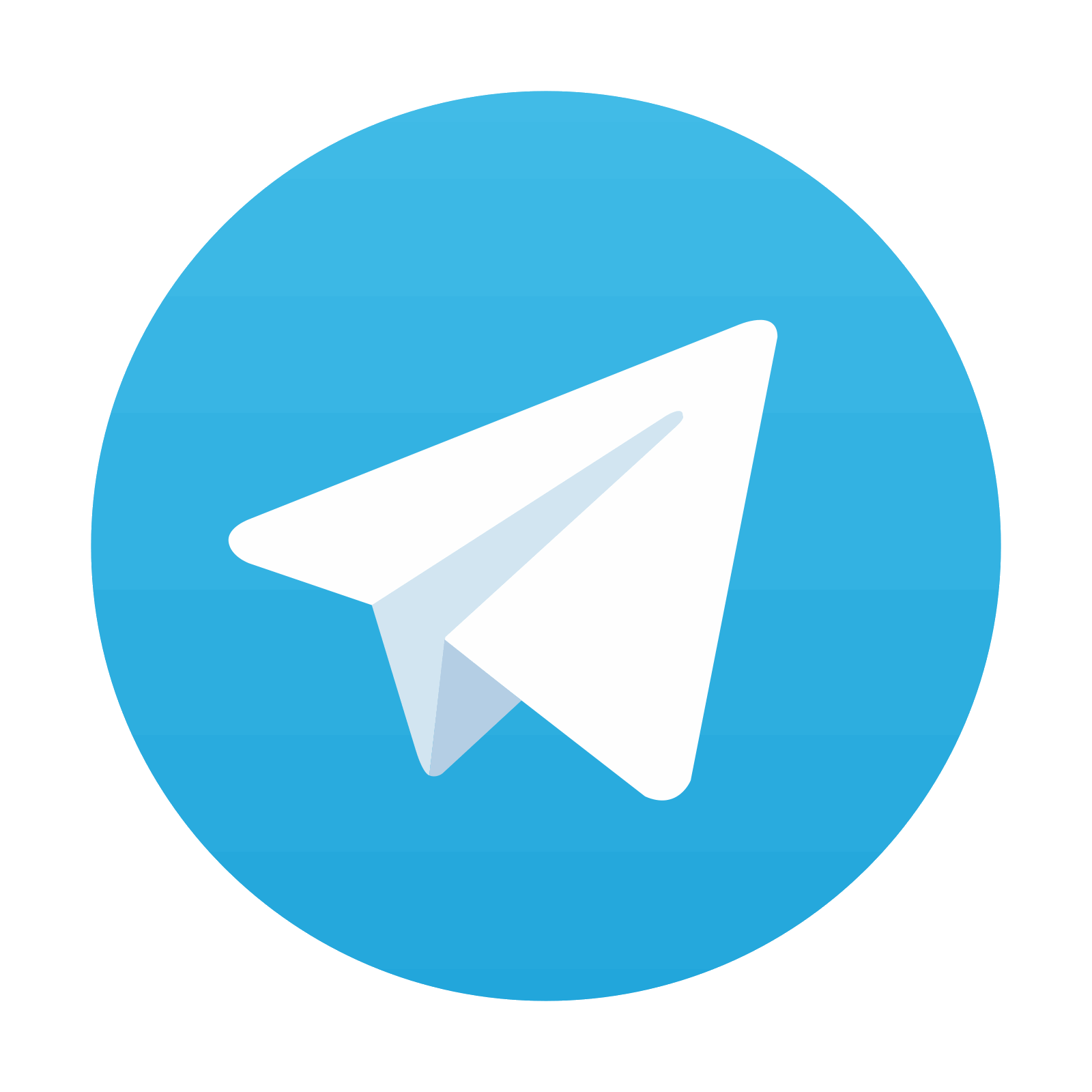
Stay updated, free articles. Join our Telegram channel
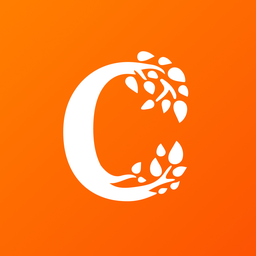
Full access? Get Clinical Tree
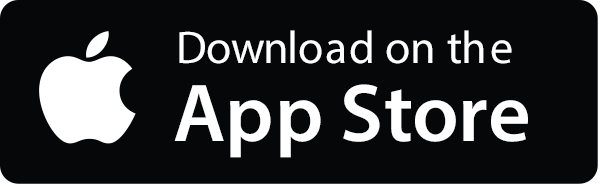
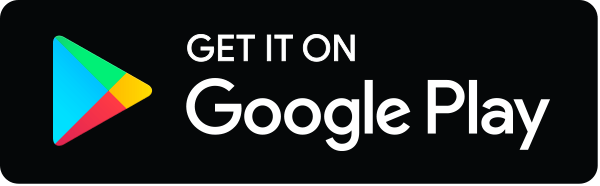