Imaging Studies in the Diagnosis and Management of Pediatric Malignancies
Rajesh Krishnamurthy
Heike E. Daldrup-Link
Jeremy Y. Jones
Stephan D. Voss
Geetika Khanna
Farahnaz Golriz
Robert P. Guillerman
INTRODUCTION
Since the publication of the previous version of this chapter, technological advances like new generation computed tomography (CT) scanners that are capable of significantly decreasing the radiation exposure associated with diagnostic CT, positron emission tomography-magnetic resonance imaging (PET-MRI) that fuses the morphologic and tissue characterization capabilities of MRI with the metabolic information from PET, and advanced postprocessing and guidance techniques like elastography and image-guided surgery, have ensured that imaging stays at the forefront of innovations in oncology. As critical assessment of the diagnostic performance of new imaging technologies lags behind their clinical application, clinical decisions often must be made in the absence of evidence-based guidelines. To provide a foundation for such decisions, this chapter discusses the relative merits of currently available imaging modalities and provides general imaging recommendations for tumor diagnosis, staging and risk stratification, therapy response assessment, surveillance for recurrent disease, screening for primary malignancy, and detection of treatment complications. Imaging characteristics and evaluation of particular pediatric malignancies are addressed in detail in the disease-specific chapters.
RELATIVE MERITS OF AVAILABLE IMAGING PROCEDURES
Conventional Radiography and Contrast Fluoroscopy
Conventional radiographs are easy to perform, rapid, inexpensive, require no anesthesia or sedation, and deliver a far smaller radiation dose than CT. Conventional chest radiography can identify primary intrathoracic tumors, mediastinal lymphadenopathy, and pulmonary metastases. Chest radiography remains the initial imaging procedure of choice for delineating central vascular catheter placement, detecting pleural or pericardial effusion or airway compression in association with a mediastinal mass (Fig. 9.1), or identifying pulmonary infections in immunocompromised patients. Coupled with injection of iodinated contrast, chest fluoroscopy is of value in determining whether malfunction of a central venous catheter is attributable to fibrin sheath formation, vascular thrombosis, or catheter breakage.
For evaluation of abdominal masses in pediatric oncology patients, conventional radiographs have been largely superseded by cross-sectional imaging modalities such as ultrasound, CT, and MRI. Nevertheless, conventional radiographs of the abdomen performed for acute indications depict many intra-abdominal masses based on the identification of tumor calcifications or mass effect on the surrounding bowel loops or parenchymal organs, and lead to the performance of cross-sectional imaging. Conventional radiographs of the abdomen also remain an excellent method for evaluating bowel obstruction, pneumatosis (Fig. 9.2), and perforation.
Fluoroscopic studies with barium or water-soluble contrast media are a simple means of evaluating for gastrointestinal (GI) tract lesions. However, with the exception of non-Hodgkin lymphoma (NHL), primary neoplastic involvement of the GI tract is rare in children. Even in the case of lymphoma, CT is more advantageous because it demonstrates not only the bowel wall, but also the mesenteric and retroperitoneal lymph nodes and the solid abdominal viscera (Fig. 9.3). Mucosal-based processes (e.g., esophagitis, gastritis, gastroduodenal ulceration, and colitis) are better seen with barium fluoroscopy studies than advanced cross-sectional imaging. However, contrast enemas are generally contraindicated in neutropenic patients because of the risk of bacteremia. CT and ultrasonography are superior imaging studies for the detection of typhlitis. Oral ingestion or enteric tube infusion of contrast agents is generally safe, even in immunocompromised children, though the presence of oropharyngeal mucositis may hinder the patient’s ability to swallow the contrast agent. Barium is an inexpensive and readily available contrast medium for the evaluation of the GI tract. However, when bowel perforation is suspected, the use of barium is contraindicated due to the proclivity of barium to exacerbate peritonitis; water-soluble contrast media should be used in this case.
Conventional radiography is also a good starting point for screening for systemic involvement, or tumor predisposition syndromes. Conventional radiographs are generally less sensitive than bone scintigraphy or MRI for detecting neoplastic involvement of the skeleton. However, skeletal radiographs should be the first imaging study of a pediatric patient with localized musculoskeletal pain (Fig. 9.4). Bone scintigraphy is generally preferred over conventional radiographic skeletal survey for the screening of bony metastases. An exception is Langerhans cell histiocytosis, in which the two approaches are complementary. Bone scintigraphy is more sensitive in detecting histiocytic lesions in the ribs, spine, and pelvis, and radiographic skeletal survey is more sensitive in identifying lesions in the skull.1 Although MRI and bone scintigraphy are highly sensitive, they are less specific than conventional radiography; thus, abnormalities detected by bone scintigraphy or MRI should be correlated with findings on conventional skeletal radiographs.
Skull radiographs are not useful for evaluating primary intracranial tumors. Skull radiographs may be included as part of a radiographic survey to detect calvarial metastases, but virtually every lesion of the skull is better depicted by CT or MRI. Even as a mechanism for screening for calvarial metastases, skull radiographs are not as sensitive as CT or bone scintigraphy. In the case of a scalp lump, skull radiographs may be obtained to evaluate for underlying cephalohematoma, fracture, or lytic lesion of the calvarium, but CT or MRI is needed if possible intracranial extension is to be defined.2
Spinal radiographs are valuable for identifying vertebral compression fractures secondary to tumor infiltration or steroid-induced osteoporosis. Spinal radiographs are less sensitive than bone scintigraphy or MRI for the early detection of metastatic disease or osteonecrosis, and CT and MRI better depict bony spinal lesions and associated intraspinal abnormalities.
Conventional myelography is occasionally helpful in evaluating patients with metallic orthopedic spinal hardware, because the presence of such hardware degrades the quality of CT and MR images.
Angiography
Diagnostic catheter angiography has been largely superseded by Doppler sonography, CT angiography (CTA), or MR angiography (MRA). Ultrasound, CT, and MRI have replaced inferior vena cavography for the evaluation of intravascular spread of malignancy, as in hepatoblastoma and Wilms tumor. Although conventional invasive catheter angiography is occasionally performed, particularly when partial hepatectomy or limb-salvage procedures are contemplated, the vasculature of most tumors, including those of the liver and extremities, can be adequately studied by the noninvasive cross-sectional imaging techniques of CTA3 or MRA.4 Invasive vascular imaging is typically employed in the context of image-guided procedures such as tumor embolization and intra-arterial chemotherapy delivery.5
Nuclear Medicine
Radiotracer-based images provide valuable information about the metabolic and functional status of a spectrum of pediatric tumors, including musculoskeletal tumors, lymphoma, neuroblastoma, and thyroid cancer. One of the most commonly used radiotracer-based imaging tests in pediatric oncology patients is bone scintigraphy, typically applied for the detection of bone metastases. The test is performed after intravenous injection of a Tc99m-labeled phosphonate radiopharmaceutical, such as methylene diphosphonate (MDP), which accumulates in osteoblasts. 2D images of the skeleton are obtained with standard gamma cameras and tomographic images can be added as needed with single-photon emission CT
(SPECT) technology (see next paragraph). Tc99m phosphonate-based bone scintigraphy is of less value than MRI in defining the local extent of primary bone tumors. Tc99m phosphonate-based bone scintigraphy has long been the examination of choice for the detection of bone metastases in children, with the exception of Langerhans cell histiocytosis.1 False-negative bone scans can occur in neuroblastoma metastasis to the ends of long bones, where uptake related to tumor can be masked by physiologic uptake within the growth plate. False-positive results can occur after amputation or limb-salvage procedures or after administration of colony-stimulating factor.
(SPECT) technology (see next paragraph). Tc99m phosphonate-based bone scintigraphy is of less value than MRI in defining the local extent of primary bone tumors. Tc99m phosphonate-based bone scintigraphy has long been the examination of choice for the detection of bone metastases in children, with the exception of Langerhans cell histiocytosis.1 False-negative bone scans can occur in neuroblastoma metastasis to the ends of long bones, where uptake related to tumor can be masked by physiologic uptake within the growth plate. False-positive results can occur after amputation or limb-salvage procedures or after administration of colony-stimulating factor.
SPECT applies mathematical reconstructions used in CT scanning to produce tomographic multiplanar images. SPECT imaging agents emit noncoincident gamma rays (as opposed to the coincident gamma rays associated with positron emitters for PET imaging). SPECT has the unique advantage that it allows detection of single photon radionuclides (e.g., Tc99m, In111, I123, Tl201, Xe133) that emit gamma rays at different energy levels, thereby potentially allowing simultaneous detection of multiple biochemical targets (multiplexing). Some examples of clinical SPECT imaging agents that target molecular processes in pediatric tumors include I123-metaiodobenzylguanidine (MIBG), a functional
analogue of norepinephrine taken up by amine transporters on the cell surface of neuroblastomas, and In111-DPTA-octreotide (DTPA, diethylenetriamine pentaacetate) for imaging the somatostatin receptor in neuroendocrine tumors.6 Limitations of SPECT include a lower sensitivity when compared to PET and lack of data quantification due to the need to estimate attenuation. However, the ongoing development of multidetector CT coupled with SPECT in newer SPECT/CT devices7,8,9 promises to significantly improve the quantitative precision of SPECT relative to PET in the future.
analogue of norepinephrine taken up by amine transporters on the cell surface of neuroblastomas, and In111-DPTA-octreotide (DTPA, diethylenetriamine pentaacetate) for imaging the somatostatin receptor in neuroendocrine tumors.6 Limitations of SPECT include a lower sensitivity when compared to PET and lack of data quantification due to the need to estimate attenuation. However, the ongoing development of multidetector CT coupled with SPECT in newer SPECT/CT devices7,8,9 promises to significantly improve the quantitative precision of SPECT relative to PET in the future.
MIBG is an analogue of norepinephrine that is concentrated in adrenergic storage vesicles of neural crest-lineage tumors such as neuroblastoma. MIBG labeled with iodine-123, iodine-124, or iodine-131 provides a radiopharmaceutical that is highly sensitive and specific for neuroblastoma.10 For planar imaging and SPECT imaging, labeling with iodine-123 is preferable to labeling with iodine-131 in children, due to more favorable radiation dosimetry, more optimal photon energy for gamma camera detection, and the ability to perform SPECT in a shorter acquisition time with the former. Iodine-131 has been used for radiotherapy of MIBG-avid neuroblastoma metastases. And more recently, iodine-124 labeling has been introduced as an alternate option for MIBG scanning with PET.11
Both MIBG and Tc-99m phosphonate-based bone scintigraphy are useful for the detection of skeletal neuroblastoma, but MIBG scintigraphy has an overall higher sensitivity. MIBG scintigraphy is favored to identify otherwise occult neuroblastomas in opsoclonus-myoclonus syndrome, which in more than half of cases in children is associated with neuroblastoma, usually small and localized.12 The use of MIBG scintigraphy to evaluate patients with neuroblastoma is routine, with the International Neuroblastoma Staging System (INSS), the new International Neuroblastoma Risk Group Staging System (INRGSS), and the International Neuroblastoma Response Criteria (INRC) recommending the performance of MIBG scintigraphy in patients with neuroblastoma at the time of initial staging and during therapy. Tc-99m phosphonate-based bone scintigraphy is suggested if the primary tumor is not MIBG-avid or if an MIBG examination is unavailable.13,14
18F-2-fluoro-2-deoxy glucose (18F-FDG) PET and 18F-FDG PET/CT imaging have been established as a clinical tool for staging and restaging of pediatric tumors for many years.11 Advantages include its high sensitivity (detecting picomolar concentrations) and a high target-to-background contrast.15 Several studies demonstrated improved sensitivities and specificities of 18F-FDG PET/CT compared to all collective standard staging procedures, specifically for patients with lymphomas, malignant sarcomas (bone and soft tissue), and head and neck cancers.16,17,18,19,20,21,22
PET is based on detection of positron-emitting radiopharmaceuticals. Intravenously injected PET radioisotopes emit positrons, which travel a small distance in tissues until they annihilate with an electron. This leads to an emission of two gamma photons of the same energy (511 keV), which leave the annihilation site in opposite directions at an angle of 180 degrees to each other. PET-detector rings use the signal from coincident gamma photons to form an image with superior spatial resolution, higher tumor-to-background uptake ratio, and more readily quantifiable data than conventional gamma camera techniques. The use of image coregistration by combined PET/CT scanners allows precise correlation of functional abnormalities with anatomic sites, permitting better differentiation between physiologic and pathologic uptake and more accurate delineation of disease for biopsy and therapy planning.23 While initial staging tests of pediatric cancers had been performed with stand-alone 18F-FDG PET scanners,24,25,26 these have been largely replaced by integrated18F-FDG PET/CT scanners.27,28
The only clinically approved radiotracer for PET imaging to date is Flourine-18 fluorodeoxyglucose (FDG), a glucose analogue that is preferentially taken up by tumor cells because of increased glucose transporter activity and glycolysis compared to normal organs. Several studies demonstrated improved sensitivities and specificities of 18F-FDG PET/CT compared to all collective standard staging procedures, specifically for pediatric patients with malignant lymphoma,29,30,31 bone sarcomas,16,32 rhabdomyosarcomas,16,32 and neck cancers.19 18F-FDG-PET/CT applications for Wilms tumor33 and neuroblastoma staging remain controversial. However, 18F-DOPA and 124I-MIBG PET/CT have recently shown higher overall accuracy than 123I-MIBG scintigraphy.20,34
The metabolic activity of solid pediatric cancers, quantified by the standardized uptake value (SUV), can provide important
information for treatment monitoring. In adult patients, physiological criteria have been introduced for some tumors, based on a decline in 18F-FDG-tumor metabolism after two cycles of chemotherapy (PERCIST criteria = Positron Emission Tomography Response Criteria in Solid Tumors).35 In PERCIST, response to therapy is expressed as percentage change in SUV peak between the pre- and posttreatment scans. A complete metabolic response is defined as visual disappearance of all metabolically active tumor (Fig. 9.5). A partial response is considered more than a 30% decline in SUV peak, and progressive disease is classified as more
than a 30% increase in SUV peak or new lesions, if confirmed.35 Accordingly, several studies in pediatric patients with malignant lymphomas and bone sarcomas have shown that a decline in tumor glucose consumption, as measured on posttreatment 18F-FDG-PET scans, correlates with chemotherapy response.30,36,37 Changes in tumor 18F-FDG-metabolism occurred before changes in tumor size were apparent. However, this concept does not apply to all pediatric tumors. In pediatric patients with stage III or IV nonlymphoblastic NHL, only response at posttreatment PET/CT, but not interim PET/CT, could predict progression-free or overall survival.36 Thus, the prognostic value of PERCIST criteria awaits systematic, tumor-specific validations in pediatric patients.
information for treatment monitoring. In adult patients, physiological criteria have been introduced for some tumors, based on a decline in 18F-FDG-tumor metabolism after two cycles of chemotherapy (PERCIST criteria = Positron Emission Tomography Response Criteria in Solid Tumors).35 In PERCIST, response to therapy is expressed as percentage change in SUV peak between the pre- and posttreatment scans. A complete metabolic response is defined as visual disappearance of all metabolically active tumor (Fig. 9.5). A partial response is considered more than a 30% decline in SUV peak, and progressive disease is classified as more
than a 30% increase in SUV peak or new lesions, if confirmed.35 Accordingly, several studies in pediatric patients with malignant lymphomas and bone sarcomas have shown that a decline in tumor glucose consumption, as measured on posttreatment 18F-FDG-PET scans, correlates with chemotherapy response.30,36,37 Changes in tumor 18F-FDG-metabolism occurred before changes in tumor size were apparent. However, this concept does not apply to all pediatric tumors. In pediatric patients with stage III or IV nonlymphoblastic NHL, only response at posttreatment PET/CT, but not interim PET/CT, could predict progression-free or overall survival.36 Thus, the prognostic value of PERCIST criteria awaits systematic, tumor-specific validations in pediatric patients.
It is important to be aware of sites of exaggerated physiologic 18F-FDG uptake in pediatric patients, such as the thymus, hematopoietic marrow, physes, benign fibro-osseous lesions, and brown fat.38,39,40 Uptake of FDG by brown fat is much more prominent in infants and children than in adults, is higher in winter than in warmer seasons, and is higher in those with lower body mass index values. FDG uptake by brown fat is most conspicuous in the lower neck, supraclavicular, and interscapular regions, but it is also seen in the axillary, mediastinal, paravertebral, and perinephric regions. In addition, it can mimic neoplastic uptake and lead to false-positive interpretations.41,42 Potentially confounding FDG uptake in brown fat can be reduced by patient warming and premedication with intravenous fentanyl or oral diazepam.43
Because of the limited availability of PET/CT scanners at most children’s hospitals, a large proportion of pediatric PET/CT studies are performed at adult imaging centers. In this setting, there are special considerations in the performance and interpretation of PET imaging of children that must not be neglected. These include fasting, sedation, or other motion-suppression techniques, radiopharmaceutical dosing, and clearance of excreted radiopharmaceutical from the urinary tract. The radiation exposure of PET/CT exams is of concern for the pediatric imaging community and various “low dose” approaches are currently being pursued,15,44,45,46,47 such as eliminating duplicate diagnostic CT scans, performing low dose diagnostic contrast-enhanced CT scans as part of the PET/CT scan, using the diagnostic CT for attenuation correction of PET data (thereby eliminating a duplicate attenuation correction scan), lowering the dose of the applied radiotracer, and replacing the diagnostic CT by MR. Integrated 18F-FDG PET/MR scans retain information about tumor metabolism, and benefit from lack of ionizing radiation, improved soft tissue contrast, and unique tissue characterization capabilities of MRI.48 These new imaging technologies could solve the conundrum of balancing the need for critical diagnostic testing for tumor assessment with concurrent risk of radiation-induced secondary cancer development later in life.
Ultrasound
Diagnostic ultrasound uses sound waves in the range of 2 to 20 MHz, well above the 20,000 Hz, which is the upper limit of normal human aural perception. Higher frequency sound permits greater imaging resolution but penetrates tissues to a lesser depth than does lower frequency sound. Thus, high frequencies (7 to 17 MHz) are used for high-resolution imaging of superficial structures (e.g., testes, thyroid gland, and breasts), and lower frequencies (2 to 7 MHz) are used for imaging deeper structures like the intra-abdominal and pelvic organs. The fluid in a structure such as the urinary bladder or a simple cyst appears sonolucent or anechoic. Solid structures, such as the abdominal parenchymal organs or soft tissue tumors, produce echoes of variable number and intensity, resulting in an echogenic appearance. Bone, fat, and the interface between air and soft tissue hinder transmission of sound waves and obscure anatomic detail.
Ultrasound is an excellent method for evaluating the abdomen, pelvis, thyroid, breasts, superficial soft tissues (Fig. 9.6), and scrotal contents of children. Its utility in other areas of the body depends on the amount of bone or gas present in or near the structure to be imaged. Diagnostic ultrasound is relatively quick and inexpensive,
uses no ionizing radiation, has no known adverse effects, and usually does not require sedation or anesthesia. High-quality abdominal ultrasound images are easier to obtain in infants and young children than in older children and adults because of the relative paucity of abdominal fat in younger patients.
uses no ionizing radiation, has no known adverse effects, and usually does not require sedation or anesthesia. High-quality abdominal ultrasound images are easier to obtain in infants and young children than in older children and adults because of the relative paucity of abdominal fat in younger patients.
Ultrasound is particularly useful in evaluation of the liver, gallbladder, spleen, kidneys, and pelvic organs. Ultrasound can reliably differentiate solid from fluid-filled masses and can usually distinguish the organ of origin of an abdominal or pelvic mass. Imaging of the pancreas, aorta, inferior vena cava, bowel, and retroperitoneal lymph nodes is less consistently successful with ultrasound than with CT or MRI because of overlying intestinal gas. During surgery, high-resolution sonographic imaging can be performed by placing a sterile-dressed ultrasound probe in direct contact with the surface of an organ of interest. Such intraoperative ultrasound is helpful in localizing tumors within the brain, spinal cord, pancreas, liver, or kidneys that are not apparent by visual inspection or palpation. The major disadvantages of conventional ultrasound are inferior resolution compared with CT or MRI for some applications, high dependence on the skill of the sonographer, the limited field of view, and the interference of bone and gas.
Recent advances in ultrasound technology have improved image resolution and diagnostic capability. Broader bandwidth transducers and coded pulse-excitation technologies provide a more favorable compromise between image resolution and penetration depth. Spatial compounding enhances delineation of tumor margins and detection of microcalcifications and low-contrast lesions. Tissue harmonic imaging improves resolution and penetration, particularly in obese patients. Panoramic extended field-of-view imaging allows large lesions and major anatomical landmarks to be included on the same image. Three-dimensional (3-D) imaging techniques permit true volumetric analysis. Elastography measures the compressibility or elasticity of tissue and is being clinically evaluated as a means of detecting and differentiating lesions in organs such as the breast, thyroid, and liver.49
Blood flow imaging has also progressed with the advent of power Doppler, advanced dynamic flow imaging techniques, and microbubble contrast agents. Although Doppler waveform analysis has limited use in distinguishing malignant from benign disease, the ability of Doppler sonography to detect intravascular thrombus is valuable in assessing thrombotic complications of therapy, guiding placement of central vascular catheters, and therapeutic planning for Wilms tumor. Microbubble contrast agents confer strong echogenicity to the blood pool that increases the conspicuity of blood vessels and many parenchymal lesions.50
Disadvantages of ultrasound include the high degree of technical skill required to obtain optimal studies, resulting in observer dependence, and a certain degree of subjective interpretation of spatial relations, morphologic measurements, and functional information.
Computed Tomography
Since the 1970s, CT has been the preferred imaging modality for the diagnosis and management of many tumors. CT of the head allows rapid identification of tumor masses, intracranial hemorrhage, hydrocephalus, and parenchymal complications of anticancer therapy. Although MRI has supplanted CT as the imaging modality of choice for detailed evaluation of the brain and contents of the spinal canal, CT imaging continues to play a major role in the evaluation of intracranial pathology, because it is quicker, less expensive, and more readily available. Although CT remains the primary advanced imaging modality for studying the contents of the thoracic, abdominal, and pelvic cavities, except for the uterus and ovaries, MRI has surpassed CT in the evaluation of most bone and soft tissue masses.
CT images are produced by a fan- or cone-shaped x-ray beam that is rotated around the body section of interest. After being attenuated by their passage through the body, the x-rays strike an array of detectors that convert the energy into electrical signals, and these signals are analyzed to construct images for display. The images can be manipulated to emphasize specific features; for example, images can be windowed to highlight the details of radiodense bones or radiolucent lungs. The advent of multidetector (multislice) helical CT scanners revolutionized the practice of CT. The x-ray tube and an array of multiple detectors rotate continuously about the patient, collecting data from multiple slices simultaneously as the patient is advanced through the gantry. Because data is acquired in a volumetric fashion, the scan time is reduced, small lesions are more accurately detected, and the quality of multiplanar and 3-D reconstructions is vastly improved. (Fig. 9.7). The new generation of CT scanners features faster gantry rotation, broader detector arrays, and dual x-ray tube sources. Multislice CT has rapidly evolved, and in 2014, 64 slice CT is commonplace, with 256 and 320 detector scanners appearing on the marketplace. A multislice CT with “x” number of detectors can obtain “x” times more
data per revolution than single slice spiral scanners. The individual detectors within the detector arrays have been reduced in size so that slice sections less than 1-mm thickness are readily achievable, enhancing the spatial resolution and the quality of image reconstructions.51 The modern CT scanners also have gantries that spin faster than three revolutions per second, further increasing the speed of data acquisition. The increased speed could be traded, if desired, for improved longitudinal resolution, increased volume of coverage, or improved image quality. This allows for considerable flexibility in CT protocols, especially in small children who cannot lie still, or cannot suspend respiration.52
data per revolution than single slice spiral scanners. The individual detectors within the detector arrays have been reduced in size so that slice sections less than 1-mm thickness are readily achievable, enhancing the spatial resolution and the quality of image reconstructions.51 The modern CT scanners also have gantries that spin faster than three revolutions per second, further increasing the speed of data acquisition. The increased speed could be traded, if desired, for improved longitudinal resolution, increased volume of coverage, or improved image quality. This allows for considerable flexibility in CT protocols, especially in small children who cannot lie still, or cannot suspend respiration.52
High-resolution CT (HRCT) enhances the ability of radiologists to identify subtle interstitial lung disease that is not apparent on chest radiographs or conventional chest CT scans. For HRCT, noncontiguous thin (typically 1.25 mm or less) axial sections are obtained during suspended respiration and images are reconstructed using a high spatial-frequency algorithm that accentuates lung parenchymal detail. In pediatric oncology, HRCT has proven valuable in identifying interstitial lung disease caused by chemotherapeutic agents, thoracic irradiation, and pulmonary Langerhans cell histiocytosis. Because of the noncontiguous nature of HRCT, only samples of the lung are obtained; thus, HRCT is not appropriate for screening the entire lungs for small nodules such as those in metastatic disease or fungal infection.
Pediatric patients tolerate ultrafast CT scanning without the sedation that is normally required for more lengthy MRI examinations. However, the use of ionizing radiation and intravenous contrast remain required in some cases. CT studies performed with iodinated contrast agents are typically more informative than those performed without contrast. The temporal pattern and intensity of enhancement across tissues vary on the basis of the relative blood volume and flow, and the extraction of contrast media by the tissues. Intravenous contrast agents do not accumulate in normal brain tissue with an intact blood-brain barrier. Thus, the administration of contrast medium increases the conspicuity of brain pathology associated with disruption of the blood-brain barrier. Contrast enhancement is particularly helpful in differentiating neoplastic tissue from surrounding edema. In the body, intravenous contrast is useful for optimal assessment of the parenchyma of the liver, spleen, and kidneys (Fig. 9.8); evaluation of intratumoral necrosis; and differentiation of blood vessels from other structures such as lymph nodes, particularly in the mediastinum and retroperitoneum.
Radiologists and oncologists should be aware of the guidelines for safe use of iodinated contrast that are periodically updated by various organizations.53 Nonionic, low-osmolar iodinated contrast agents are most commonly used in children and have a favorable safety profile; acute allergic-like reactions occur with a frequency of 0.18% per administration and are usually mild.54 Previous reaction to contrast is the most important risk factor in prediction of an adverse event, and the likelihood of a recurrent reaction is 8% to 25%. A history of other allergies or asthma is associated with a small elevation in the risk of reaction. The overall risk of allergic-like reactions can be reduced by premedication with steroids with or without antihistamines. Premedication should be considered in those with previous allergic-like reaction to iodinated contrast material, multiple (four or more) allergies or a severe allergy to another substance, or asthma with frequent, recent, or severe attacks.55
Contrast-induced nephropathy (CIN) typically manifests as an increasing serum creatinine level within 24 to 48 hours after contrast administration, peaking at 3 to 5 days, and returning to baseline by 10 days. Most cases are nonoliguric; thus, renal insufficiency may be clinically occult. In more severe cases, serum creatinine levels may continue to rise for 5 to 10 days and, in rare cases, dialysis is needed. Risk factors for CIN include preexisting renal insufficiency, diabetes mellitus, dehydration, large volume of injected contrast medium, severe congestive heart failure, concomitant nephrotoxic medications, and a history of contrast-associated acute renal failure.56
Intravascular iodinated contrast agents are relatively contraindicated or warrant particular caution in patients with renal insufficiency, a history of severe adverse reactions to contrast, severe thyrotoxicosis, suspected or known pheochromocytoma, and those receiving metformin or high-dose methotrexate. In the case of suspected or known pheochromocytoma, the risk of adrenergic hypertensive crisis provoked by intravascular iodinated contrast may be mitigated by pharmacologic adrenergic blockade prior to administration of contrast, although the intravenous administration of nonionic contrast media is most likely safe, even without such blockage.57 Children and adolescents may be on metformin (dimethylbiguanide) for treatment of non-insulin-dependent
(type 2) diabetes mellitus or insulin-resistance syndromes. Renal insufficiency leads to retention of metformin and the development of potentially fatal lactic acidosis. Renal function should be measured in patients on metformin before considering the administration of intravascular contrast, and published guidelines for the safe administration of intravascular contrast agents for elective and emergent radiologic procedures in patients on metformin with normal or impaired renal function should be followed.53 High-dose methotrexate is used in the treatment of various pediatric malignancies and is associated with mucosal, bone marrow, liver, central nervous system (CNS), and renal toxicity. Both methotrexate and intravenous iodinated contrast are excreted by glomerular filtration, and their combined exposure incurs a higher risk for nephropathy and methotrexate toxicity. To prevent methotrexate toxicity, iodinated contrast should not be administered until the patient’s serum methotrexate level is less than 0.1 mmol/L.58
(type 2) diabetes mellitus or insulin-resistance syndromes. Renal insufficiency leads to retention of metformin and the development of potentially fatal lactic acidosis. Renal function should be measured in patients on metformin before considering the administration of intravascular contrast, and published guidelines for the safe administration of intravascular contrast agents for elective and emergent radiologic procedures in patients on metformin with normal or impaired renal function should be followed.53 High-dose methotrexate is used in the treatment of various pediatric malignancies and is associated with mucosal, bone marrow, liver, central nervous system (CNS), and renal toxicity. Both methotrexate and intravenous iodinated contrast are excreted by glomerular filtration, and their combined exposure incurs a higher risk for nephropathy and methotrexate toxicity. To prevent methotrexate toxicity, iodinated contrast should not be administered until the patient’s serum methotrexate level is less than 0.1 mmol/L.58
Enteric contrast is routinely used for abdominal and pelvic CT scans to help differentiate bowel loops from lymph nodes and soft tissue masses. The use of enteric contrast is especially important in children to increase lesion conspicuity because of the relative lack of retroperitoneal and mesenteric fat surrounding and separating anatomic structures. Orally administered barium suspensions or diluted iodinated agents are typically used for enteric contrast. Significant adverse reactions to enteric contrast are very rare.
The disadvantages of CT include its cost, risk of adverse reaction to contrast media, the need for conscious sedation or general anesthesia in some patients, and the relatively high dose of ionizing radiation. The dose of ionizing radiation can range from less than 1 mSv for a sinus CT to approximately 5 to 7 mSv for a body CT, although this may go up to 30 mSv if multiple phases are obtained, and adult rather than pediatric technique parameters are followed.59 By comparison, the effective dose for a pediatric chest radiograph is 0.02 to 0.12 mSv.60 Thus, the effective dose for a pediatric CT is the equivalent of tens to hundreds of chest radiographs. The number of CT examinations performed, including those performed on children, is rapidly increasing.61 Not only is CT the primary method for diagnosis, staging, response evaluation, and surveillance of many pediatric malignancies, it is also a routine method for evaluating complications. This escalating use of CT has prompted public health concerns, because children are at higher risk of radiation-induced malignancy.
Estimates of the risk of radiation-induced malignancy from pediatric CT examinations have received much attention. The lifetime attributable risk of fatal cancer from a pediatric abdominal CT scan is estimated at approximately 0.07% to 0.14%, assuming a linear, no-threshold dose response and applying the cancer mortality data observed in the A-bomb survivor cohort.61 However, such mathematical modeling has methodological limitations; for example, the radiation doses may differ substantially from those imparted by contemporary low-dose CT examinations, and the risk of cancer from CT examinations is calculated rather than observed. Demonstrating an added cancer mortality risk of 0.1% is difficult by epidemiologic methods, because cancer is the cause of death in more than 20% of the overall population, and a very large number of subjects would be required for an adequately powered study.62 Several recent population based studies have reported higher risks for cancer in children exposed to CT scan compared to adults.63,64,65,66,67 A retrospective cohort study of 180,000 young patients who underwent CT scan before 22 years of age in the United Kingdom demonstrated excess risk of leukemia and brain tumors in the first decade after the CT scan. According to their study, cumulative radiation doses of 50 mGy can triple the risk of leukemia, and radiation doses of 60 mGy can almost triple the risk of brain cancer.65 Mathews et al.66 evaluated a large cohort of patients in the Australian National Medicare system that was exposed to CT scan during childhood and adolescence and found an increase in the incidence of many types of cancer.
The current evidence suggests that the risk posed by radiation from CT is minimal, but real, and must be balanced against the benefits of the examination. When performed for appropriate indications, especially in newer generation scanners, with proper technical parameters that follow the ALARA (as low as reasonably achievable) principle for patient exposure, the benefits of CT far exceed the very small individual risk. Besides strictly limiting the indications for pediatric CT to those in which diagnostic information cannot be obtained from an alternative nonradiating imaging modality such as ultrasound or MRI, there are several means of decreasing the radiation dose, including confining the study to the anatomical region of interest, using a volumetric technique rather than a helical technique, using a larger pitch, adjusting settings based on the child’s size, body region scanned, and clinical indication, minimizing the use of multiphasic examinations, using low tube voltage in contrast-enhanced studies such as CT angiography, adopting new dose-reducing technology such as automatic tube current modulation, iterative image reconstruction algorithms, and protective bismuth shields for radiosensitive tissues,68,69,70,71 shielding of sensitive areas like breast, thyroid, gonads, bone marrow, and the eye, and avoiding the need for repeat examinations by attention to meticulous technique, including use of proper immobilization, and sedation.
Clinicians are responsible for judiciously ordering CT and nuclear medicine examinations and considering the use of alternative modalities such as ultrasound and MRI that do not involve ionizing radiation. Approximately 30% of CT examinations performed in young patients are judged to be noncontributory or unjustified.72 In the context of pediatric oncology, CT examinations are frequently obtained for staging, evaluation of therapy response during treatment, and surveillance after treatment, as well as for evaluation of unexplained fever and complications of therapy. Clinical trial protocols for pediatric cancers often require that multiple radiographs, nuclear medicine examinations, and CT examinations be obtained, resulting in cumulative radiation doses of at least 100 mSv.73 Further research is needed to determine whether outcomes would be adversely affected by using fewer of these examinations, particularly repetitive low-yield examinations with relatively high-dose radiation. Given the obvious benefits of diagnostic imaging, the risks of radiation from CT or other radiologic examinations should be kept in proper perspective, and balanced against the higher risks of complications from the disease and its therapy, particularly in patients with shortened life expectancy from aggressive cancers. Inaccurate or nondiagnostic results due to a poor-quality CT examination or failure to perform a CT examination when indicated carries a high risk of harm to the patient, and overconcern about the long-term effects of radiation could be more harmful than the risk from excess radiation.74
Magnetic Resonance Imaging
MRI involves interactions between a strong external magnet field, radiofrequency (RF) waves, and the hydrogen nuclei in the body. Once aligned in the strong magnetic field, the body’s hydrogen nuclei precess or “wobble” at a frequency proportional to the magnetic field’s strength. When the hydrogen nuclei are bombarded with RF waves at the precessional frequency, energy is absorbed by the nuclei. The RF waves are then turned off, and the hydrogen nuclei return to their initial alignment. This results in a release of energy that induces voltage in a wire-receiving coil, producing the MR signal. Spatial encoding, the process of localizing the MR signal to generate an image, is accomplished by the use of magnetic field gradients. Unlike CT, in which the slices are almost always obtained in the transaxial plane, MRI slices can be obtained in any desired plane.
The most common strength of external magnetic fields found on current MRI scanners is 1.5 T, about 30,000 times the strength of the earth’s magnetic field. To achieve high-field (1.0 to 3.0 T) or ultrahigh-field (>3.0 T) strengths, superconducting magnets are used. High-field systems have higher signal-to-noise ratio and thus better image quality, improved MR spectroscopy, and functional
MRI capability. Disadvantages of higher field systems include the higher magnet cost, the need for greater shielding, which restricts selection of suitable sites for scanner installation, and an increase in some artifacts.
MRI capability. Disadvantages of higher field systems include the higher magnet cost, the need for greater shielding, which restricts selection of suitable sites for scanner installation, and an increase in some artifacts.
To produce a useful diagnostic image, contrast in appearance between normal tissues is needed for anatomic detail, and contrast in appearance between normal and diseased tissue is needed for sensitivity to pathology. The primary sources of tissue contrast in MRI are differences in hydrogen spin densities, T1 relaxation times, and T2 relaxation times. The particular weighting of these sources of tissue contrast is determined by the MRI pulse sequence design. Pulse sequences are continuously being developed and refined for various applications, and a detailed description of the multiple MR sequences available is beyond the scope of this chapter. On T1-weighted images, substances with short T1 relaxation times (e.g., fat, methemoglobin, melanin, proteinaceous fluid, and gadolinium contrast) are depicted with bright signal intensity, and those with longer T1 relaxation times (e.g., edema, tumor, hemosiderin) are depicted with intermediate-to-low signal intensity. On T2-weighted images, substances with short T2 relaxation times (e.g., white matter, tendon, hemosiderin, and fibrosis) are depicted with low-to-intermediate signal intensity, and substances with longer T2 relaxation times (e.g., edema, tumor, cerebrospinal fluid [CSF]) are depicted with bright signal intensity (Fig. 9.9). Images acquired with TR and TE intermediate to those used for T1- and T2-weighted images are often referred to as proton-density images. The signal characteristics on these images reflect a mixture of T1 and T2 relaxation times and the proton density of the imaged tissue.
Pulse sequence development beyond conventional spin echo sequences has been a fertile area for clinical investigation. These efforts have focused primarily on suppressing signal from certain tissues, reducing scanning time, and increasing conspicuity of blood flow. Inversion recovery (IR) sequences permit selective nulling of signal from a specific tissue based on its T1 relaxation time and the chosen inversion time (TI). A variant of the IR sequence, short tau inversion recovery (STIR), selectively suppresses the signal from fat and has proven very valuable in body and musculoskeletal applications, particularly when fat suppression by chemical shift methods is unsuccessful. The fluid-attenuated inversion recovery sequence suppresses signal from the CSF and is highly sensitive for lesions in the brain and spinal cord.
Rapid imaging techniques include low flip angle gradient echo sequences, RF-refocused fast (turbo) spin echo sequences, single shot sequences, echo planar imaging, and parallel imaging simultaneously using multiple receive coils. Rapid imaging techniques allow more body coverage, more sequences performed in less time (improved temporal resolution), or more detailed images obtained in the same time (improved spatial resolution).75 These are particularly valuable attributes for performing MRI in children, because small structures are commonly imaged, and minimizing patient motion is imperative. Pediatric whole-body MRI scanning with rapid sequences is now feasible in 25 minutes or less of acquisition time and is particularly suited for surveying the bone marrow.76
Considerable attention has been directed to diffusion-weighted MRI (DWI), a technique that characterizes tissues on the basis of the Brownian molecular motion of constituent water. There is an inverse relationship between the apparent diffusion coefficient (ADC) and tumor cellularity due to the restricted diffusion of water molecules among densely packed cells (Fig. 9.10). DWI is highly sensitive for the detection of acute cerebral ischemia. The ADC is predictive of pediatric brain tumor classification77 and assessment of ADC ratios of enhancing regions in the follow-up of treated high-grade gliomas is useful in differentiating radiation effects from tumor recurrence or progression.78 By exploiting the anisotropic diffusion of water in relation to ordered structures, special applications of DWI termed diffusion tensor tractography and diffusion spectrum imaging can be used to delineate the course and integrity of important white matter tracts in the brain.79
In the body, DWI can detect and delineate a wide range of pediatric tumors from adjacent tissues. Solid tumor components tend to show restricted diffusion and correlate with sites of gadolinium contrast enhancement; liquefactive necrotic or cystic components tend to show increased diffusion.80 The technique of diffusion-weighted whole-body imaging with background body signal suppression (DWIBS) allows volumetric diffusion-weighted images of the entire body to be acquired during free breathing, highlights lesions with restricted diffusion, and provides excellent visualization of lymph nodes.81 However, the use of ADC measurements has not enabled differentiation between malignant and benign extracranial tumors in children.82
MRA has become a widely accepted tool for noninvasively depicting blood vessels in children. The most popular MRA technique currently used in pediatric body imaging is time-resolved gadolinium-enhanced 3-D MRA, a method that relies on the shortening of T1 relaxation time by gadolinium-chelate contrast media within the lumen of the vessel of interest. Timing of injection of contrast and image acquisition allows arteries or veins to be selectively imaged. Time-of-flight, phase contrast (Fig. 9.9), arterial spin labeling, and 3-D steady-state free-precession MRA techniques do not require administration of intravenous contrast and are particularly useful in the setting of renal insufficiency or lack of vascular access, when gadolinium-enhanced MRA may not be feasible. In pediatric oncology, MRA is valuable for depicting vascular anatomy in planning resection or transplantation for liver tumors,4 detecting intravascular invasion by abdominal tumors, and planning limb salvage procedures in cases of extremity sarcomas. MRA has also proven useful in assessing the venous system for patency before central vascular catheter placement, particularly in children with suspected thrombotic veno-occlusive disease related to prior catheters.83
Although the superior soft tissue contrast differentiation of MRI permits identification of most tumors without the administration of contrast agent, intravenously administered gadolinium-chelate contrast agents are used in MRI much like intravenously administered iodinated contrast agents in CT to make tumors more conspicuous, assess tumor vascularity, and evaluate intratumoral necrosis. Gadolinium is a paramagnetic metal that provides enhancement by shortening the T1 and T2 relaxation times. Gadolinium is toxic as a free ion and is usually administered as a chelate to reduce the toxicity and increase elimination, mainly by the kidneys. Acute allergic-like reactions to gadolinium-chelates are rare in children, occurring with a frequency of 0.04% per administration, and are usually mild. Recently, the administration of gadolinium-chelate contrast agents has been associated with the subsequent development of nephrogenic systemic fibrosis (NSF) in patients with acute or chronic renal insufficiency. NSF is characterized by progressive tissue fibrosis, usually starting in the skin of the extremities and advancing over the course of days or weeks to the trunk and extracutaneous structures such as the skeletal muscles, heart, lungs, and esophagus, leading to restricted range of motion, disability, and even death. The risk of NSF is increased by higher gadolinium-chelate doses and greater degrees of renal insufficiency, implying a role of prolonged tissue retention of gadolinium in its pathogenesis, although NSF does not develop in most patients with renal insufficiency, even if they are exposed to high doses of gadolinium-chelates. Circulating fibrocytes and cytokines are thought to mediate the fibrosing process and other possible associated risk factors include high-dose erythropoietin, systemic inflammation, trauma, metabolic acidosis, lanthanum phosphate binders, hepatorenal syndrome, and the perioperative liver transplantation period. Pediatric cases are rare, but have been reported, the youngest being 6 years of age. There have been no reports to suggest that the physiologic renal immaturity of neonates and infants poses an additional risk factor. Like iodinated contrast agents, gadolinium-chelate MRI contrast agents are removed by dialysis, but dialysis is of unproven efficacy for preventing NSF. Rather than relying on serum creatinine, one should calculate the glomerular
filtration rate or estimate it by using the Schwartz formula. The risk-benefit should be assessed, and alternative imaging techniques that do not entail the use of gadolinium chelates should be considered for pediatric patients with renal insufficiency.84
filtration rate or estimate it by using the Schwartz formula. The risk-benefit should be assessed, and alternative imaging techniques that do not entail the use of gadolinium chelates should be considered for pediatric patients with renal insufficiency.84
The dynamics of contrast uptake by tumors is based on the premise that the rate and intensity of contrast uptake correlates with the density and permeability of the microvasculature of viable tumor. Dynamic contrast-enhanced MRI (DEMRI) consists of rapid sequential MR imaging of a tumor following the bolus administration of intravenous gadolinium chelate. Theoretically, DEMRI can predict accessibility of the tumor to drugs before treatment and viability of residual tumor during or after treatment. Viable tumor tends to exhibit early and intense enhancement, while necrotic and fibrotic areas show slower, less intense, or absent enhancement. In DEMRI of bone sarcoma, rapid and pronounced contrast uptake into the tumor prior to preoperative chemotherapy, greater decrease in uptake during therapy, and low uptake at the completion of preoperative chemotherapy correspond to a better response and longer disease-free survival. Analysis of contrast uptake by DEMRI can predict clinically important degrees of necrosis in bone sarcomas in response to induction or neoadjuvant preoperative chemotherapy.85 Response prediction by MRI could facilitate the development of risk-adapted treatment approaches.86 The routine application of DEMRI has been hindered by the need for mathematical postprocessing of the imaging data and the lack of a simple standardized approach for use across institutions with varying MRI equipment capabilities.
Magnetic resonance spectroscopy (MRS) allows noninvasive determination of the relative concentrations of various metabolites in vivo. MRS is based on the principle that small differences in the resonance frequencies of nuclei are determined by the chemical environment. Each metabolite is identified by the position of its peak (or chemical shift) on a frequency scale, with the area under the peak proportional to the concentration of the metabolite within the voxel. In single-voxel spectroscopy, the spectra are obtained during a short period of time, but the volume of interest is usually large and contains heterogeneous tissue. In multivoxel methods, more time is required to acquire the spectra, but the voxel size is smaller and representative of more homogeneous tissue.87 In the brain, the principal metabolites measured by 1H-MRS are choline (Cho), N-acetyl aspartate (NAA), lactate (Lac), and creatine (Cr). Cho is a marker for cell membrane turnover; NAA is a neuronal marker; Lac is a marker of anaerobic metabolism; and Cr is involved in energy metabolism and serves as an internal standard because it varies little in normal brain tissue. Pediatric brain tumors generally exhibit elevated Cho levels, decreased or absent NAA levels, and Lac levels that vary with the degree of necrosis. The 1H-MRS method can be used to identify areas in tumors with the highest Cho levels to improve the diagnostic yield of brain biopsies. The maximum tumor Cho:NAA ratio is predictive of outcome in children with recurrent primary brain tumors,88 and the percentage change in Cho:NAA ratio by 1H-MRS is an important prognostic indicator of tumor progression in children with brain tumors. Metabolite profiles vary with age in developing tissues, and the metabolic profiles of differing pathologies often overlap. MRS quality is very susceptible to degradation by patient motion and magnetic field inhomogeneity, such as in the posterior cranial fossa where pediatric brain tumors are common. The size of the selected volume has to be relatively large (around 1 cm3 for 1H-MRS and 27 cm3 for 31P-MRS) and care must be taken to minimize partial volume effect and signal contamination from adjacent tissue. Some of these technical limitations of MRS are lessened by the higher signal-to-noise ratio and improved spectral resolution of higher field MRI systems that are increasingly available.89
MRI offers several advantages over CT. MRI delineates normal soft tissues and discriminates abnormal from normal soft tissues better than CT and does so without the use of ionizing radiation. Iodinated contrast agents are not required, beam-hardening artifacts from bone are eliminated, and images can be acquired in any desired plane. The disadvantages of MRI include its relatively high cost, limited availability, insensitivity to the presence of calcifications, and limited ability to assess lung parenchyma and bony cortex. MRI image quality is highly susceptible to degradation by normal cardiac and respiratory motion, bowel peristalsis, and vascular pulsations, a disadvantage that is further compounded by the slower image acquisition of MRI compared with ultrasound, CT, or conventional radiography. In addition, gross patient motion substantially compromises the quality of MR images. As a consequence, children who would not require sedation for other imaging modalities often require sedation for MRI, though fast MRI techniques are ameliorating this disadvantage. Safely sedating pediatric patients during an MRI examination requires monitoring of vital signs with MR-safe and MR-compatible monitoring equipment. Facilities that sedate children for MRI examinations or other diagnostic tests should institute standardized protocols based on guidelines for pediatric sedation that have been developed and recommended by professional organizations.90
Since its introduction in the 1980s, the role of MRI in the evaluation of pediatric cancers has expanded at a rapid pace. MRI is now the imaging study of choice for brain and spinal tumors, musculoskeletal tumors, and tumors of the body wall. MRI is complementary to ultrasound in the assessment of the female genital tract and is valuable in the evaluation of certain thoracic, abdominal, and pelvic malignancies.
MRI is superior to CT in detecting CNS tumors, especially in the posterior fossa. Evaluation of multiplanar images allows more precise localization of neoplastic disease as intra-axial or extra-axial. The vertebrae and the contents of the thecal sac can be studied without the need for myelography. MRI is the modality of choice for evaluation of spinal cord compression or intraspinal invasion by neoplastic masses (Fig. 9.11). However, the small bones of the skull and face are often not adequately evaluated by MRI. Therefore, if
precise evaluation of the small bones of the face and skull is required, CT is recommended, in addition to or in place of MRI.
precise evaluation of the small bones of the face and skull is required, CT is recommended, in addition to or in place of MRI.
Application of MRI to extracranial and extraspinal structures has advanced rapidly. MRI allows direct acquisition of images in the coronal and sagittal planes and has the ability to visualize flowing blood in vessels without the need for intravenous contrast. MRI is equal to or better than CT in the anatomic definition of many abdominal, pelvic, and mediastinal tumors. MRI is generally superior to CT in the evaluation of musculoskeletal tumors (Fig. 9.6), except in the detection of calcification or early cortical bone erosion. MRI is superior to CT, conventional radiographs, or Tc-99m phosphonate-based bone scintigraphy in defining the precise extent of neoplastic infiltration of the bone marrow and extraosseous soft tissues, making MRI the imaging modality of choice for local-regional staging of primary bone tumors. The increased accuracy of MRI in defining the local-regional extent of musculoskeletal tumors is particularly important when primary radiation therapy or limb-salvage surgery is being considered.
MRI is valuable for the assessment of bone marrow disease in pediatric patients.91 The T1 relaxation time in marrow involved with leukemia is longer than that of normal marrow (Fig. 9.11), and marrow from patients with relapsed leukemia shows a similar prolongation of this measure compared with that of marrow from those in remission. The diagnosis of relapsed leukemia can be made by MRI several weeks before it is appreciable by iliac bone marrow aspirate or biopsy, because vertebral marrow, as the primary site of hematopoiesis in children, may reflect the changes of leukemia earlier than iliac crest marrow. MRI of the marrow is also useful in the evaluation and detection of focal areas of macroscopic lymphoma in patients who are symptomatic but have negative bone scans.
The widespread practice of prenatal sonography has led to the recognition of fetal tumors and subsequent pediatric oncology referral. Promoted by the development of fast sequences averting the need for maternal sedation or fetal paralysis and by advances in fetal medicine and surgery, fetal MRI has emerged over the past decade as a useful adjunct to prenatal sonography to better define the extent, composition, and potential complications of fetal tumors. The most common fetal tumors amenable to differentiation by fetal MRI are teratomas, lymphatic malformations, cardiac rhabdomyomas, neuroblastomas, hemangiomas, and mesoblastic nephromas.92 Fetal MRI is superior to ultrasound in delineating tumor mass effect on the fetal airway and neck vessels for planning of airway management at birth.
Interventional Radiology
Like diagnostic radiology, interventional radiology has been the beneficiary of technologic advances that have broadened the contribution of radiologists to the diagnosis and management of pediatric cancers. Refinements in microcatheters, digital fluoroscopy, ultrasound, CT, and MRI have greatly increased the scope of what is feasible for image-guided intervention. Evolution in flat-panel detectors, 3D rotational angiography, and fast CT and MR fluoroscopy techniques has further facilitated image-guided intervention.
Image-guided percutaneous biopsy or aspiration provides a method of tissue sampling for tumor diagnosis or microorganism culture that is less invasive than surgical methods. Almost any lesion of the neck, trunk, or extremities that is visible by imaging is amenable to sampling by interventional radiology procedures. The choice of imaging modality for guidance and method of tissue sampling depends on the interventional radiologist performing the procedure, the site of the lesion, the condition of the patient, and the type of specimen needed to make the diagnosis. In experienced hands, the procedures are generally safe, although they may require anesthesia in young children. In the case of thin-needle biopsies, cytological examination by an experienced pathologist is necessary to determine whether a lesion is benign or malignant. Often, the precise nature of a malignancy cannot be diagnosed, and for this reason, thin-needle biopsies have been more useful for the determination of recurrent disease than primary diagnosis. If a specific histologic diagnosis is needed from a percutaneous biopsy, larger-bore aspiration needles or cutting needles are used to obtain core biopsy specimens. A systematic review of image-guided percutaneous core-needle biopsies of pediatric tumors cited a pooled diagnostic accuracy rate of 94% in cases with adequate material.93 For these procedures, the rate of complications, such as bleeding requiring blood transfusion or pneumothorax requiring chest tube placement, is approximately 1%. These procedures, like all other image-guided interventions, should be performed by an experienced radiologist who is capable of treating potential complications. The major limitations of percutaneous biopsy are related to sampling problems and specimen inadequacy. Although image-guided percutaneous core-needle biopsies of childhood tumors provide an adequate sample for specific diagnosis in 95% of cases, performance of biological studies mandated by some cancer cooperative group clinical trial protocols may require relatively large volumes of tissue. Coordination between the interventional radiologist and pathologist is required for appropriate tissue sampling, handling, and processing to produce adequate specimens for diagnostic pathologic evaluation. Close communication with the oncologist and surgeon is also desirable to plan the path of the biopsy needle through tissue that would be surgically removed if the lesion was malignant, to avoid seeding of the biopsy tract with tumor cells.
Interventional radiology techniques can also be used to localize small lesions for subsequent surgical biopsy or resection. CT or ultrasound can be used to guide needle localization of small soft tissue masses. Preoperative CT localization of pulmonary nodules with methylene blue injection allows small pulmonary nodules or those not located near the pleural surface to be identified and resected thoracoscopically rather than via a more morbid thoracotomy.94
There are indications other than tumor diagnosis for image-guided biopsy in pediatric oncology patients. Percutaneous image-guided lung biopsy can help determine the etiology of pulmonary infiltrates or nodules in immunocompromised patients less invasively than open, thoracoscopic, or transbronchial lung biopsy. In pediatric patients who have undergone bone marrow transplantation and in whom graft-versus-host disease (GVHD) or hepatic veno-occlusive disease (HVOD) is a clinical concern, a liver biopsy may be safely performed via a transjugular approach, when percutaneous liver biopsy is contraindicated because of thrombocytopenia, coagulopathy, or ascites.95
Interventional radiology plays a role in not only the diagnosis but also the therapy of pediatric oncology patients. Preoperative transcatheter selective arterial chemoembolization can be used to induce surgical resectability of previously unresectable hepatoblastoma, especially in patients without distant metastases.96 Transcatheter arterial chemoembolization has also been used to successfully treat liver disease in progressive stage 4s neuroblastoma.97 Image-guided tumor ablation with chemical agents (e.g., ethanol, acetic acid), thermal energy (e.g., RF, microwave, laser, cryogen), or radiation (e.g., yttrium-90 microsphere radioembolization) are emerging methods for symptom palliation and local tumor control. Ongoing experience suggests that this technique is a viable therapeutic option in children with primary and metastatic tumors of the liver, lung, kidney, and skeleton that are not amenable to surgery, chemotherapy, or radiotherapy.95 Multiple lesions may be treated at one session, with the number of lesions treated limited by the patient’s overall condition and the potential risk of renal insufficiency induced by tumor lysis, hemoglobinuria, and myoglobinuria. Potential complications and side effects include injury to adjacent structures, such as bile ducts, bowel, and renal collecting systems, and pain or postablation syndrome, which can include low-grade fever and malaise lasting for several days.
Interventional radiologists are active in the treatment of complications of tumors and tumor therapy. Such treatments include, but
are not limited to, relief or diversion of biliary and urinary tract obstruction (Fig. 9.8), percutaneous abscess drainage, and angioplasty, stenting, or endovascular fibrinolysis for vascular occlusive disease. In addition, radiologists place chest tubes, enteric feeding tubes, and central vascular catheters. Tunneled central vascular catheter placement in pediatric oncology patients has been associated with a lower rate of infection and mechanical complications when performed by image-guided radiology techniques than by surgery.98
are not limited to, relief or diversion of biliary and urinary tract obstruction (Fig. 9.8), percutaneous abscess drainage, and angioplasty, stenting, or endovascular fibrinolysis for vascular occlusive disease. In addition, radiologists place chest tubes, enteric feeding tubes, and central vascular catheters. Tunneled central vascular catheter placement in pediatric oncology patients has been associated with a lower rate of infection and mechanical complications when performed by image-guided radiology techniques than by surgery.98
Interventional radiology procedures involving fluoroscopy or CT for guidance can be associated with relatively high doses of ionizing radiation. In some instances, high doses can induce skin erythema, desquamation, and epilation. These deterministic effects may not present for up to several weeks after the irradiation. Patients receiving more than 3 Gy skin entrance dose should be identified and asked to return for a follow-up examination in 30 days to monitor for these effects. Pediatric oncology patients undergo repeated diagnostic radiology studies during the course of their disease, and interventional radiology procedures are a potential contributor to large cumulative radiation exposures in these patients. It is important that such procedures be performed by interventional radiologists cognizant of the ALARA principle and methods of radiation dose optimization in these patients.99
GENERAL CONCEPTS OF PEDIATRIC TUMOR IMAGING
Imaging plays an important role in guiding clinical management of childhood cancer. This includes detection of conditions requiring emergent or urgent treatment, diagnosis, staging of disease burden and extent for risk stratification, determination of optimal therapy, and assessment of response to antitumor therapy. Imaging also serves as a method of surveillance for tumor relapse and complications of therapy. Details of the diagnostic imaging of specific tumors are discussed in other chapters of this book. Some basic guidelines for the diagnostic imaging of children with malignancies are provided in this section.
Detection of Conditions Requiring Emergent or Urgent Treatment
True life-threatening oncologic emergencies requiring immediate intervention are fortunately uncommon. However, a number of conditions exist for which the radiologist plays a central role in rendering a diagnosis and alerting the clinician to the urgent nature of the process at hand. It is useful to categorize oncologic emergencies as resulting from metabolic, hematologic, or structural abnormalities.100 Metabolic disorders are typically identified on the basis of laboratory and clinical findings, and unless they have a structural basis or imaging correlate (e.g., diabetes insipidus and hypothalamic/pituitary lesions in Langerhans cell histiocytosis) are not primarily imaging diagnoses. An organ system approach to defining structural abnormalities provides a basis for both categorizing lesions and determining the most appropriate imaging and clinical management and includes lesions with primary cardiorespiratory, abdominopelvic, genitourinary and central nervous system involvement, accepting that there is often overlap between these systems, depending on the tumor type.
Cardiorespiratory emergencies in a child, such as pneumothorax or pneumomediastinum, airway compression secondary to a large mediastinal mass, and malignant pericardial or pleural effusions, demand particular attention from the radiologist. If radiographs indicate the presence of significant airway compromise in the setting of a large mediastinal mass (Fig. 9.1), supine positioning, with or without sedation, may lead to further airway compression, impaired venous return and acute cardiorespiratory collapse,101 and CT or MR imaging may be contraindicated without anesthesia consultation. Such mediastinal masses are most commonly encountered in the setting of lymphoma, but may also be seen with mediastinal germ cell tumors and sarcomas and in patients with neuroblastoma. Pleural and pericardial effusions occur in patients with lymphoma, but often become quite large with associated pleural and chest wall involvement in sarcoma patients.
Pneumothoraces occur in oncologic patients in various settings, both at the time of presentation and following tumor response to therapy. Not all patient with pneumothorax present with acute symptoms; indeed some are identified incidentally during routine treatment monitoring (Fig. 9.12). Nonetheless, all should be treated as relative emergencies and require prompt intervention. Similarly, acute pulmonary emboli occur with increased frequency in oncology patients, both as a result of treatment and occasionally at the time of presentation, and while they may be discovered incidentally,102 they should always be regarded as requiring urgent attention.
Abdominopelvic emergencies encountered in pediatric cancer patients include pneumoperitoneum, pneumatosis, bowel ischemia, mesenteric vascular thrombosis, and intussusception. Intussusception is a well-appreciated presentation for patients with Burkitt lymphoma (Fig. 9.3) and may require prompt surgical intervention both for diagnosis and management of the intussuscepted bowel. Even in the absence of intussusception, very large abdominal masses may produce intestinal obstruction (Fig. 9.7), in addition to compressing vital structures such as vessels, nerves, and ureters. Depending on the degree of obstruction, urgent
intervention may be indicated to decompress the affected structure. Pneumatosis can be seen incidentally, particularly in patients receiving high doses of corticosteroids as part of their therapy, as well as in the early post-stem cell transplant setting (Fig. 9.2). However, pneumatosis must be investigated further, since it can also be a sign of bowel ischemia and may be associated with pneumoperitoneum, both of which require urgent intervention.
intervention may be indicated to decompress the affected structure. Pneumatosis can be seen incidentally, particularly in patients receiving high doses of corticosteroids as part of their therapy, as well as in the early post-stem cell transplant setting (Fig. 9.2). However, pneumatosis must be investigated further, since it can also be a sign of bowel ischemia and may be associated with pneumoperitoneum, both of which require urgent intervention.
Obstruction of the genitourinary system can result from masses arising primarily from the kidneys, ureters, or bladder or from extrinsic compression by large intra-abdominal, pelvic, or retroperitoneal masses. Depending on the nature and acuity of the obstruction, an urgent drainage procedure may be required, such as nephrostomy, ureteral stenting (Fig. 9.8), ureterostomy, or suprapubic cystostomy.
CNS emergencies usually result either from mass-occupying lesions causing compression of vital structures, acute cerebral edema (either cytotoxic edema related to cellular necrosis or vasogenic edema), and intracranial hemorrhage (Fig. 9.9). Patients presenting with acute CNS symptoms such as altered consciousness, seizures, motor disturbance or peripheral sensorimotor loss require urgent attention. For evaluation of intracranial processes, CT is the initial modality of choice and can effectively assess for hemorrhage, significant cerebral edema resulting in mass effect, hydrocephalus, and impending herniation. In many cases, CT will provide an initial characterization of the offending lesion, but is almost always followed by MRI once the patient has been stabilized. For symptoms related to spinal cord compression, MRI is the imaging modality of choice and provides accurate assessment of lesion location, extent of spinal cord compression, and associated edema (Fig. 9.11). While CNS emergences are often encountered at the time of presentation, some brain tumor therapies—particularly radiation and antiangiogenic agents—can result in significant hemorrhage and edema as the tumor undergoes necrosis. Asparaginase, which is an effective component of many leukemia treatment regimens, has a well-appreciated association with cerebral sinus thrombosis,103 and patients being treated with this agent who experience new CNS symptoms should undergo urgent head CT to assess for hemorrhage, followed by MRI/MRA to directly evaluate the cerebral sinuses and to assess for presence/extent of cerebral edema (Fig. 9.9).
Diagnosis
Central Nervous System Tumors
CNS neoplasms are the most common solid tumors of childhood and a leading cause of mortality. As they often present with nonspecific signs and symptoms, imaging plays a pivotal role in their diagnosis and management. CT, despite the drawback of ionizing radiation, remains a primary screening tool in the emergency setting due to its widespread availability and speed. CT is sensitive for intracranial neoplasm, with the caveat that infiltrating tumors such as gliomatosis cerebri, small posterior fossa and sellar/suprasellar tumors, and secondary leptomeningeal tumor dissemination may go undetected.104 CT also has utility in assessing tumoral calcification and cortical bone involvement. However, once an intracranial mass is detected or in the nonurgent setting, MRI is the standard of care in diagnosis and assessment. Its ability to acquire a variety of soft tissue contrasts allows superior localization and characterization of intracranial tumor. With MRI features in hand, a limited location-related and age-specific differential diagnosis can be generated, assisting in management of the patient (Fig. 9.10). For posterior fossa tumors, MRI has a significant advantage in depicting the origin of tumor, its relationship to the fourth ventricle and cisterns, and its contiguity with cranial nerves or local arteries. For supratentorial tumors, the location of the tumor with respect to eloquent gyri can be precisely delineated. The relationship of the tumor with respect to deep white matter tracts can also be depicted with diffusion tensor imaging and tractography, as warranted for surgical planning.105,106
It must be remembered that not all intracranial masses that present in the pediatric age group are neoplasms, and these mimics can often be diagnosed or suggested on the basis of MR features. Intracranial pyogenic abscesses, although not common in the antibiotic era, do occur and presents as ring-enhancing masses. They can usually be differentiated on the basis of their thin and hypointense T2-weighted rim in conjunction with fairly homogeneous diffusion restriction internally. This is opposite the pattern of high-grade glioma, which usually has facilitated diffusion centrally reflecting necrosis and a rim of diffusion restriction peripherally reflecting viable tumor.107 Although hemorrhagic masses in children can be the result of underlying tumor, arteriovenous malformations and cavernous malformations are more likely culprits. The former can often be diagnosed on the basis of abnormal vessels on conventional MRI or MRA, while the latter often has a characteristic “popcorn” appearance of heterogeneous signal on T1-weighted images and a complete, low T2-weighted, hemosiderin rim.108,109 Tumefactive demyelinating lesions can present as intracranial masses in the pediatric population, confounding tumor diagnosis. These are often multiple, typically demonstrate incomplete rim enhancement, and usually decrease in size with serial imaging.110
Although MR imaging cannot replace the histopathologic diagnosis of CNS tumors, there are a few situations in which the combination of clinical and MRI findings may preclude the need for biopsy. In the setting of a child with neurofibromatosis type I, a tumor growing along the optic pathway is consistent with a low-grade glioma, likely pilocytic astroctoma. Pineal or suprasellar masses with elevation of germ cell serum markers can generally be treated without tissue confirmation. Finally, brainstem gliomas, apart from clinical trials, are often diagnosed on the basis of imaging without biopsy. The diffuse expansion of the pons with partial encasement of the basilar artery is considered diagnostic.104
Primary tumors involving the spine are significantly less common than intracranial tumors during childhood. Again, MRI is the primary modality utilized in the assessment of these masses, characterizing them into intramedullary, intradural extramedullary, and extradural locations. Intramedullary tumors comprise only 2% to 4% of pediatric CNS tumors, with pilocytic and low-grade fibrillary astrocytomas making up the majority. Ependymomas and gangliogliomas make up most of the rest. MRI features do not readily differentiate among these tumor types, although ependymomas are more likely to demonstrate hemorrhage, have an eccentric location, and displace white matter tracts.111 Extradural tumors of the spine make up to two-thirds of pediatric spine tumors and demonstrate a variety of histologies. Paraspinous neuroblastoma growing into bone or neural foramina is one of the more common (Fig. 9.1). However, a wide variety of benign tumors and sarcomas can involve the bone or epidural space, including Ewing sarcoma, rhabdomyosarcoma, lymphoma, histiocytosis, osteoid osteoma, osteoblastoma, and aneurysmal bone cyst (Fig. 9.11). CT is often complementary in assessing bone involvement in these tumors. The most common intradural extramedullary neoplasm in children is of course leptomeningeal metastasis from an intracranial primary (Fig. 9.10). Myxopapillary ependymoma is a strong consideration for an intradural extramedullary expansile mass about the cauda equina. Schwannomas and neurofibromas are characteristic in the setting of the neurofibromatosis.112
Finally, CNS leukemia, the most common childhood tumor, can involve the leptomeninges, brain parenchyma, or cerebral vasculature at presentation. Abnormal enhancement of the meninges and nerve roots in a child with leukemia suggests leptomeningeal involvement, even if CSF cytologic studies are negative. Small intraparenchymal hemorrhages are occasionally the initial manifestation of acute childhood leukemia.113
Extracranial Head and Neck Tumors
Most neck masses in children are of a congenital or inflammatory nature rather than neoplastic, and the optimal imaging approach depends on the most likely etiology of the mass, as determined by
clinical evaluation. The location of the mass and the nature of the mass as solid or cystic are key factors in formulating an appropriate differential diagnosis. Ultrasound is particularly useful in determining whether a mass is cystic or solid. In the case of a suspected neoplasm in the neck, CT and/or MRI are utilized to assess for lymphadenopathy, airway compression, vascular encasement, perineural spread, bone involvement, and intracranial or intraspinal extension.
clinical evaluation. The location of the mass and the nature of the mass as solid or cystic are key factors in formulating an appropriate differential diagnosis. Ultrasound is particularly useful in determining whether a mass is cystic or solid. In the case of a suspected neoplasm in the neck, CT and/or MRI are utilized to assess for lymphadenopathy, airway compression, vascular encasement, perineural spread, bone involvement, and intracranial or intraspinal extension.
Cervical lymph node enlargement is the most common cause of a palpable neck mass in children. Frequently, this represents reactive hyperplasia or lymphadenitis related to head and neck infection. Ultrasound may be used to confirm cervical lymphadenopathy and evaluate for suppurative changes. However, in the setting of cervical lymph node enlargement in which lymphoma or other neoplasm is a concern, CT or MRI is frequently performed to better define the extent of lymphadenopathy, including possible mediastinal extension.
Vascular malformations and vascular tumors are also a common cause of an extracranial head or neck mass. Vascular malformations are classified on the basis of their endothelial characteristics as arterial, capillary, venous, lymphatic, or combined. Vascular malformations are present at birth and grow commensurately with the child, in some cases causing morbid complications due to impingement on vital structures. Vascular malformations are not restricted to the head and neck and are commonly seen in the axillary regions and extremities. MRI is preferred for the imaging of vascular malformations because of its superior ability to depict lesion extent and distinguish venous from lymphatic malformations, on the basis of enhancement of the fluid-filled spaces of the former but not of the latter after the administration of the intravenous contrast (Fig. 9.13). The finding of phleboliths within a lesion also helps establish a diagnosis of venous malformation. In contrast, hemangiomas of infancy are vascular tumors that appear during early infancy, undergo a proliferative phase, and then involute. Most hemangiomas of infancy occur in the cervicofacial region and have an especially high incidence in premature infants of low birth weight. On MRI or CT, hemangiomas appear as intensely enhancing solid masses with prominent high-flow vessels (Fig. 9.14).
An anterior midline or paramedian cystic mass is typically a thyroglossal duct cyst or dermoid cyst, and the cystic nature can be confirmed with ultrasound. Branchial cleft cysts and thymopharyngeal duct cysts are more laterally located and have characteristic positions in relation to the neck musculature and vasculature. A congenital neck mass with solid and cystic components is most commonly a teratoma.114,115
To avoid unnecessary concern or biopsy, it is important not to confuse fibromatosis colli for a neoplastic mass. Fibromatosis colli
classically presents as a mass along the anterior neck during the first few weeks to months after birth, often in association with torticollis. Sonographic demonstration of a heterogeneously echoic mass-like swelling along the sternocleidomastoid muscle and a normal appearance of the adjacent soft tissues help confirm the diagnosis of fibromatosis colli. Ectopic cervical thymic rests are usually an incidental finding noted on neck imaging, and misdiagnosis of a neoplasm can be avoided by recognition of their characteristic imaging appearance, although an intrathyroidal location can be difficult to distinguish from other more common causes of a thyroid nodule.116
classically presents as a mass along the anterior neck during the first few weeks to months after birth, often in association with torticollis. Sonographic demonstration of a heterogeneously echoic mass-like swelling along the sternocleidomastoid muscle and a normal appearance of the adjacent soft tissues help confirm the diagnosis of fibromatosis colli. Ectopic cervical thymic rests are usually an incidental finding noted on neck imaging, and misdiagnosis of a neoplasm can be avoided by recognition of their characteristic imaging appearance, although an intrathyroidal location can be difficult to distinguish from other more common causes of a thyroid nodule.116
The two most common solid malignant tumors in the extracranial head and neck in children are lymphoma and rhabdomyosarcoma. CT is used to assess lymphoma at presentation, but MRI is preferred to evaluate intracranial extension of solid tumors associated with bony destruction at the skull base or calvarium, including from rhabdomyosarcoma. Parameningeal spread may occur from rhabdomyosarcomas arising in the sinonasal region, pterygopalatine fossa, nasopharynx, or middle ear. During adolescence, in addition to lymphoma and rhabdomyosarcoma as differential possibilities for a nasopharyngeal mass, nasopharyngeal carcinoma is also a consideration. The presence of an asymmetric mass with central skull base invasion, parapharyngeal space invasion, or lateral retropharyngeal lymphadenopathy on images distinguishes malignancy from benign adenoidal hypertrophy117 (Fig. 9.15).
The finding of an intensely enhancing and hypervascular mass on CT or MRI centered about the posterior nasal cavity and with involvement of the sphenopalatine foramen or vidian canal in an adolescent boy is highly suggestive of juvenile nasopharyngeal angiofibroma.118
Primary parotid tumors in children can be well localized with MRI, with characterization however reserved for the pathologist. The most common benign parotid tumor, as in adults, is a pleomorphic adenoma.119 The most common malignant tumor is mucoepidermoid carcinoma, also noted for its propensity as a second malignancy in leukemia patients.120
Thyroid nodules or goiter can present as a neck mass. Ultrasound is the preferred modality for evaluating the thyroid gland (Fig. 9.16). Tiny cysts within the thyroid are frequently seen and usually represent benign colloid cysts. Nearly 70% of solid solitary thyroid nodules represent follicular adenomas. However, thyroid nodules cannot be reliably distinguished as benign or malignant on the basis of sonographic findings. A combination of clinical and sonographic features guides assessment and follow-up.121 Neck irradiation for a childhood malignancy is a well-established risk factor for development of thyroid carcinoma.
Prompt diagnosis and treatment of ocular or orbital tumors is crucial because these lesions threaten vision. The most common tumor of the globe is retinoblastoma, which usually presents in the first few years of life and is associated with a mutation in the RB1 tumor suppressor gene. Retinoblastomas calcify in approximately 95% of cases, a useful distinction because other causes of leukocoria calcify less frequently and less vigorously. CT is particularly sensitive for detecting these calcifications (Fig. 9.17). However,
MRI is now the preferred imaging modality, assessing optic nerve invasion, extraocular extension, and intracranial spread.122
MRI is now the preferred imaging modality, assessing optic nerve invasion, extraocular extension, and intracranial spread.122
![]() Figure 9.17 Retinoblastoma. Axial computed tomography image of the head shows a calcified soft-tissue mass (arrow) in the left globe, which is characteristic of retinoblastoma. |
As many as half of all cases of acute leukemia involve ocular manifestations, and the most frequent finding is retinal hemorrhage. Orbital chloromas (granulocytic sarcoma, extramedullary leukemia) are seen primarily with acute myelogenous leukemia and can be a presenting sign preceding the blast phase. Other extraocular intraorbital malignancies include rhabdomyosarcoma, optic nerve glioma, and orbital bony involvement by neuroblastoma metastases and Langerhans cell histiocytosis. Most extraocular intraorbital masses in childhood are benign; these include hemangiomas, vascular malformations, retro-orbital colobomatous cysts, and (epi) dermoid cysts. These lesions often have characteristic imaging features that allow distinction by CT or MRI.123
Thoracic Tumors
Most pediatric malignancies involving the lungs are metastases from extracranial solid tumors and are discussed in the portion of this chapter devoted to tumor staging. Primary malignancies of the lung parenchyma or airways bronchi, such as pleuropulmonary blastoma, inflammatory myofibroblastic tumor, bronchial carcinoid (Fig. 9.18), mucoepidermoid carcinoma, and bronchogenic carcinoma, are very rare in children. Affected children can be asymptomatic or manifest with a wide range of respiratory symptoms and signs, including respiratory distress, fever, cough, hemoptysis, or wheezing related to mass effect or airway involvement. Paraneoplastic syndromes, including Cushing syndrome from ectopic ACTH production, can occur with carcinoids. Primary pediatric lung tumors are typically diagnosed by conventional radiography and CT imaging, and the appearances will vary with tumor type. The cystic form of pleuropulmonary blastoma can be indistinguishable from the large cyst form of congenital cystic adenomatoid or pulmonary airway malformations on imaging and gross pathology (Fig. 9.19), and many previous reports of malignancy developing in a congenital lung cyst most likely represented progression of cystic pleuropulmonary blastoma. A substantial proportion of pleuropulmonary blastomas are associated with a susceptibility syndrome in the patient (or family) for certain dysplastic or neoplastic conditions, including cystic nephromas,
ovarian stromal sex cord tumors, seminomas, and intestinal polyps. Cystic pleuropulmonary blastomas are characterized by multifocality and spontaneous pneumothorax, and excision of congenital lung cysts associated with these conditions during infancy is advocated because of the probability that they represent pleuropulmonary blastoma.124 Juvenile-onset recurrent respiratory papillomatosis caused by human papilloma virus is associated with airway papillomas and occasional pulmonary involvement manifesting as cavitary lung nodules and an elevated risk of squamous cell bronchogenic carcinoma at an early age125 (Fig. 9.20). The initial imaging appearance of round pneumonia can be confused with a primary or metastatic pulmonary tumor; however, round pneumonia is usually readily distinguished on clinical grounds, though follow-up imaging is warranted if the clinical presentation or course is not typical.
ovarian stromal sex cord tumors, seminomas, and intestinal polyps. Cystic pleuropulmonary blastomas are characterized by multifocality and spontaneous pneumothorax, and excision of congenital lung cysts associated with these conditions during infancy is advocated because of the probability that they represent pleuropulmonary blastoma.124 Juvenile-onset recurrent respiratory papillomatosis caused by human papilloma virus is associated with airway papillomas and occasional pulmonary involvement manifesting as cavitary lung nodules and an elevated risk of squamous cell bronchogenic carcinoma at an early age125 (Fig. 9.20). The initial imaging appearance of round pneumonia can be confused with a primary or metastatic pulmonary tumor; however, round pneumonia is usually readily distinguished on clinical grounds, though follow-up imaging is warranted if the clinical presentation or course is not typical.
Primary tumors of the mediastinum are best defined by the compartment in which they arise. Tumors arising in the posterior mediastinum are largely of neurogenic origin, with neuroblastoma being the most common malignant tumor (Fig. 9.1). Rib erosion, widening of spinal neural foramina, and calcification of the mass are conventional radiographic signs that suggest neuroblastoma. Although the differential diagnosis is extensive, common benign lesions have radiographic features that usually differentiate them from neuroblastoma, ganglioneuroma, and ganglioneuroblastoma. Neurenteric cysts, for instance, are associated with congenital anomalies of the thoracic spine, whereas neurofibromas are frequently associated with acute-angle scoliosis and multiple small paraspinous masses at several levels along the spine. Calcifications are also absent in these two benign conditions.
Cross-sectional imaging is essential in the evaluation of a possible posterior mediastinal neuroblastoma. CT is often performed because of logistical issues and is frequently sufficient, although MRI is the best test for detecting nodal or chest wall involvement and intraspinal extension. CT can strongly suggest neuroblastoma by depicting calcification within the mass (90% of neuroblastomas are calcified on CT, but only 50% are calcified on conventional
radiographs). MRI is less sensitive than CT for detecting intratumoral calcification.
radiographs). MRI is less sensitive than CT for detecting intratumoral calcification.
The middle mediastinum or hilar regions may be involved by lymphadenopathy secondary to leukemia or lymphoma, but this rarely occurs in the absence of anterior mediastinal lymphadenopathy or thymic infiltration. Plexiform neurofibromas and inflammatory myofibroblastic pseudotumors may involve the middle mediastinum or hila as large infiltrating masses. Although nonmalignant, these entities can grow aggressively and cause significant morbidity or mortality due to involvement of the tracheobronchial tree, esophagus, or great vessels. CT and/or MRI are the desired imaging modalities for evaluation of the middle mediastinum and hila. In CT evaluation of the mediastinum, intravenous contrast should be used when feasible to distinguish vascular structures from enlarged nodes or other soft tissue masses. MRI has the advantage of being able to distinguish vascular structures from nodes or other soft tissue masses without the use of intravenous contrast. MRI is particularly useful in depicting and sometimes differentiating benign and malignant cardiac and paracardiac masses, and advances in MRI technology have made MRI superior to echocardiography in the evaluation of many cardiac masses.126 CT is preferable to MRI when evaluation of the lungs is needed.
The anterior mediastinum is the mediastinal compartment most frequently involved by malignant disease in children. Anterior mediastinal tumor involvement may present as an anterior mediastinal soft tissue mass, lymphadenopathy, or thymic infiltration. Lymphoma and leukemia are by far the most common anterior mediastinal neoplasms in children, followed by germ cell tumors, histiocytosis, and thymoma.
Analysis of anterior mediastinal masses in children by conventional chest radiographs is difficult because of the wide range of normal thymic size. The thickness of the thymus decreases with age, and normal pediatric thymus size standards for CT,127 MRI,128 and ultrasound129 have been published. Identifying malignancy within the thymus is complicated because the normal gland can be quite large, especially in infants and young children. Conversely, the gland can be involved by malignancy without being overtly enlarged. Often, the diagnosis of intrathymic neoplasm is simplified when a prominent thymus is seen in conjunction with lymphadenopathy in other areas of the mediastinum or hila. In the absence of associated lymphadenopathy, the finding of loss of the normal contour or loss of the normal homogeneous parenchymal architecture of the thymus suggests neoplastic infiltration.
In children younger than 5 years, the thymus is quadrilateral in shape with convex lateral margins and undulating borders from costal cartilage impressions. Thereafter, the thymus gradually assumes a triangular shape with straight or concave margins. On CT, the normal attenuation of the thymus approximates that of skeletal muscle. On MRI, the signal intensity of normal thymus is lower than that of fat and slightly greater than that of skeletal muscle on T1-weighted images and is greater than that of skeletal muscle and slightly lower than that of fat on T2-weighted images.128 On ultrasound, the echotexture of the pediatric thymus resembles that of liver parenchyma, and its echogenicity is lower than that of thyroid parenchyma.129 Before puberty, the thymic parenchyma appears homogeneous. After puberty, fatty infiltration of the thymus occurs.127 Chemical shift in-phase and opposed-phase gradient-echo MRI can be used to detect the fatty infiltration and possibly distinguish a normal or hyperplastic thymus from neoplastic infiltration.130 The normal thymus exerts little or no mass effect on adjacent structures and can extend superiorly into the thoracic inlet or posteriorly into the posterior mediastinum. In these cases, normalcy can be established by the findings of homogeneous parenchyma, absence of significant compression of adjacent tracheobronchial structures or mediastinal vessels, and continuity with thymus in the anterior mediastinum.
The high avidity of lymphoma for gallium and FDG suggests a possible role for gallium scintigraphy and FDG-PET in distinguishing lymphoma from other causes of thymic enlargement or an anterior mediastinal mass. However, both methods are of limited use for definitive diagnosis of anterior mediastinal lymphoma due to the nonspecificity of thymic uptake of gallium and FDG. Various infectious and inflammatory conditions of the chest exhibit uptake of FDG.131,132 Thymic uptake of gallium can occur with lymphoma or nonlymphoid neoplasms, particularly in very young children; this uptake may represent a nonspecific immunologic response. The thymus can demonstrate gallium or FDG uptake as a physiologic phenomenon in children or as a result of rebound thymic hyperplasia after chemotherapy, and this uptake should not be mistaken for recurrent or metastatic thymic malignancy.133 The pattern of physiologic thymic FDG uptake consists of mild diffuse uptake, most commonly in an inverted V-shape, whereas lymphomatous involvement of the thymus appears as intense discrete foci of uptake.134 Rebound thymic hyperplasia most characteristically presents in a child or adolescent as an enlarging thymic mass within 6 to 8 months of completion of chemotherapy for lymphoma and may portend a good prognosis related to bolstered cell-mediated immunity135 (Fig. 9.21). In rare cases, it may persist for several years.136 If a child or adolescent has imaging findings compatible with rebound thymic hyperplasia or physiologic thymic radiopharmaceutical uptake, especially if there is no other evidence of recurrence or prior neoplastic involvement of the thymus, observation and imaging follow-up rather than biopsy is advised.135
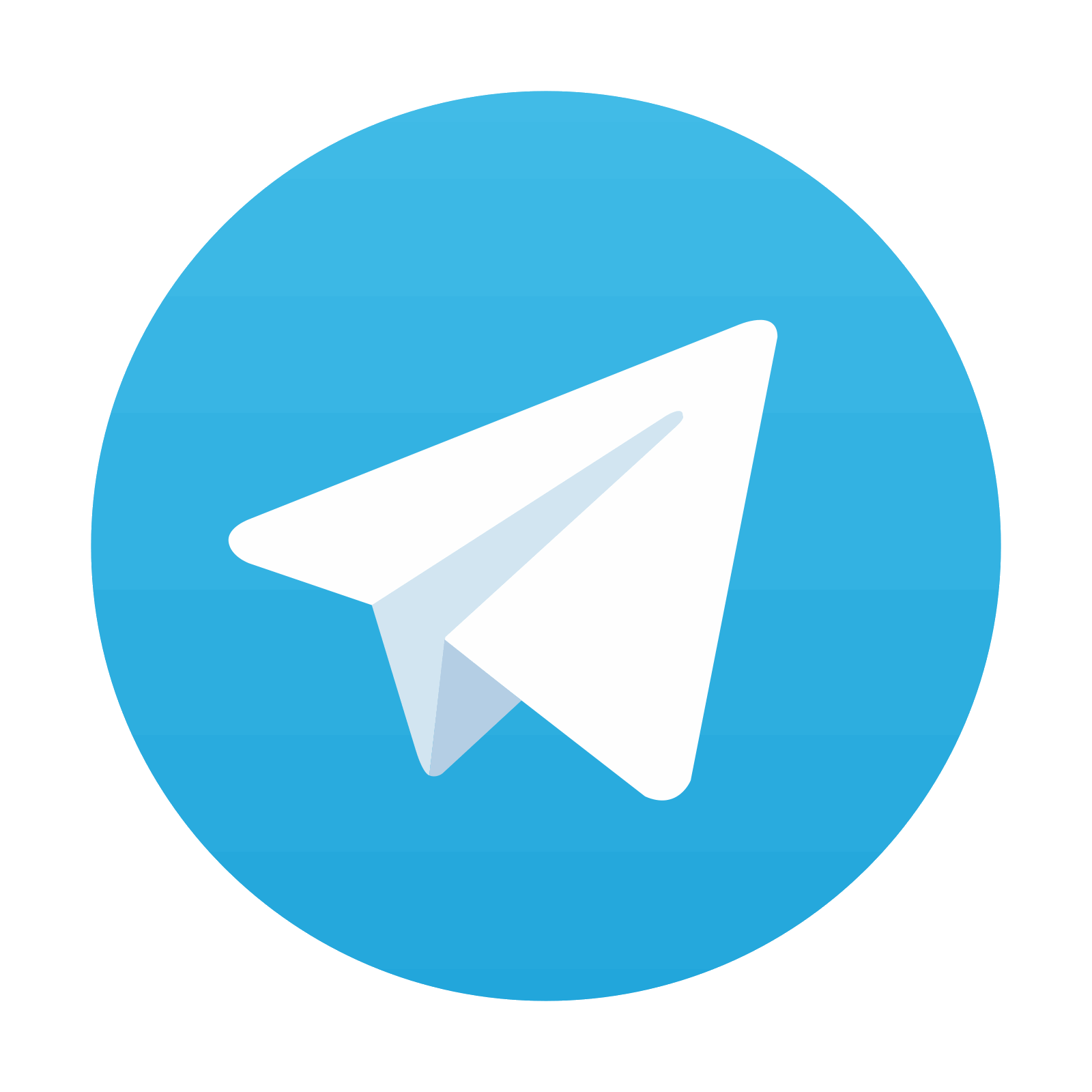
Stay updated, free articles. Join our Telegram channel
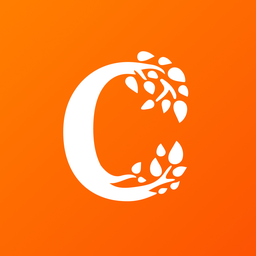
Full access? Get Clinical Tree
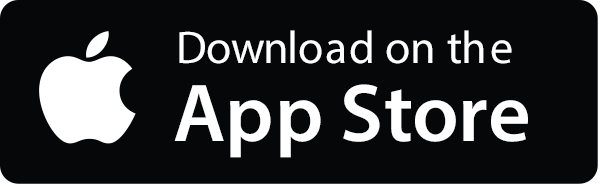
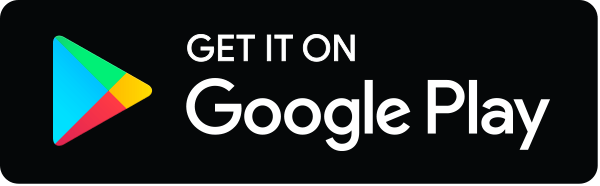
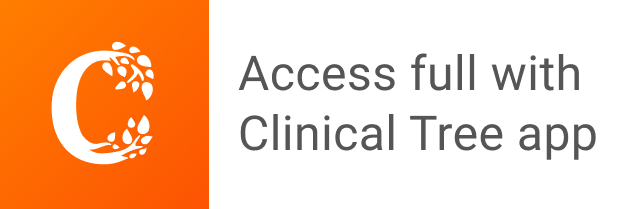