Stephen J. Chapman, Adrian V.S. Hill
Human Genetics and Infection
Genetic variation in the host has a substantial influence on the course of infectious diseases caused by many microorganisms. Such interactions have been well-studied in humans because the pathogens and the host genome are well characterized. In recent years, the methodology and techniques available for analyzing human genetic variation have advanced rapidly, leading to the identification of a large number of genes associated with altered susceptibility to infectious pathogens. Entire human genome sequences and millions of mapped single nucleotide polymorphisms (SNPs) provide a powerful resource for molecular analysis of differential susceptibility. Although many genes have already been associated with susceptibility to various diseases, it is likely that these represent only a small fraction of all the relevant genes. Indeed, susceptibility to most infectious diseases in humans is likely to be highly polygenic, and the identification of susceptibility and resistance genes is providing new insights into disease pathogenesis and resistance mechanisms. Conversely, it appears increasingly likely that a substantial proportion of the functional variation in the human genome has evolved to facilitate defense against infectious pathogens, leading to the observed polygenic variation in susceptibility among individuals and different human populations.
Magnitude of the Host Genetic Effect
There are various types of evidence that demonstrate a significant role for host genetics in variable susceptibility to infectious disease. For some infections, such as leprosy, disease has long been known to cluster in families,1 but it is often difficult to assess the relative importance of proximity to an index case and shared genes. Nonetheless, the increased risk of disease in a sibling compared with the general population is a useful measure of the extent of the genetic component in a multifactorial disease and appears to be of the order of 1.5 to 5 for several infectious diseases.2
Apparent interpopulation and racial differences in susceptibility have also been noted.3,4 Particularly striking is the apparently increased susceptibility to viral infections and tuberculosis (TB) in some previously unexposed populations.5 In the Qu’Appelle Indians of Saskatchewan, after some decades of exposure to TB and resulting high mortality, the incidence of disease dropped 50-fold, likely reflecting a very strong selective pressure against TB susceptibility loci.6 The use of malaria therapy for syphilis in nonimmune individuals7 and the accidental vaccination of some children with Mycobacterium tuberculosis rather than the Calmette-Guérin bacillus, in Lübeck, Germany, provided direct evidence of variable susceptibility to these pathogens.
In addition to these observational data, studies of adoptees and of twins have provided more direct quantification of the importance of host genetics in susceptibility to infectious diseases. In a study of more than 900 Scandinavian adoptees, the early death of a biologic, but not an adoptive, parent from an infectious disease was associated with an almost sixfold increase in the risk of an infectious cause of death in the adoptee, consistent with a substantial role for host genetics.8 Also, several twin studies have found higher concordance rates among monozygotic than among dizygotic twin pairs, who share, respectively, 100% and, on average, 50% of their genes. The diseases studied include TB, poliomyelitis, leprosy, persistent hepatitis B virus (HBV) infection, Helicobacter pylori infection, and malaria (Table 10-1).9–14 In general, it has been easier to demonstrate a significant role for host genetic factors in infections in which only a proportion of those infected develop disease, in chronic rather than in acute infections, and in the severity of infectious disease rather than susceptibility to infection per se.
TABLE 10-1
Twin Studies of Selected Infectious Disease Phenotypes*
CONCORDANCE | |||
PHENOTYPE | POPULATION | MZ | DZ |
Tuberculosis | Germany | 65 | 25 |
United States | 62 | 18 | |
United Kingdom | 32 | 14 | |
Poliomyelitis | United States | 36 | 6 |
Leprosy | India | 52 | 22 |
Hepatitis B | Taiwan | 35 | 4 |
Helicobacter pylori infection | Sweden | 81 | 63 |
* All showed significantly higher concordance in monozygotic (MZ) than in dizygotic (DZ) twin pairs.
Approaches
Several different and usually complementary approaches have been taken to identifying genes involved in susceptibility to infectious diseases. The most widely adopted strategy in human studies has been the assessment of candidate genes in case-control association studies. Here, the frequencies of common variants (with a frequency >1%, defined as polymorphisms) of a gene with a suspected role in resistance are compared in individuals with and without the disease. However, the degree of replication between reported association studies of candidate genes is often disappointingly low, in part reflecting limitations in study design. In particular, such studies often have inadequate sample sizes and fail to perform statistical correction for multiple testing of different polymorphisms. Despite these shortcomings, a number of robust genetic associations with infectious disease susceptibility have been successfully identified using the candidate gene approach.
A different approach has been to search for genetic linkage to, rather than association with, an infectious disease in family studies. Identification of a chromosomal region genetically linked to susceptibility indicates that there is a susceptibility gene (or genes) somewhere in that region. Limitations of the linkage approach have been the difficulty in recruiting multicase families as well as a lack of study power. Such family-based linkage approaches have been successfully used, however, to map and identify genes underlying rare monogenic susceptibility phenotypes. For example, mutations in the gene for the interferon-γ (IFN-γ) receptor chain 1 were found to cause some rare cases of susceptibility to weakly pathogenic mycobacteria.15,16 Extensive sequencing of candidate susceptibility genes has been used to identify the molecular basis of a large number of rare monogenic syndromes that increase susceptibility to particular infections. These rare syndromes are reviewed elsewhere17–19 and include mutations in the Toll-like receptor (TLR) pathway genes IRAK4 (interleukin-1 receptor-associated kinase 4) and MyD88 (myeloid differentiation primary response gene 88), increasing susceptibility to pyogenic bacteria; variants of genes involved in TLR3 signaling, conferring susceptibility to herpes simplex encephalitis; and mutations in members of the interleukin-12 (IL-12)/IL-23/IFN-γ signaling pathway, conferring susceptibility to atypical mycobacteria and salmonellae. Identification of these rare mutations has advanced understanding of human immunology but has been of limited value in identifying gene variants that affect susceptibility to common infectious diseases at the population level. Rare mutations in genes leading to primary immunodeficiency states are described in other chapters.
More recently, the availability of millions of SNPs mapped onto the human genome sequence has allowed the development of microarrays of typically 0.5 to 1 million SNPs, allowing markers across the entire human genome to be studied for possible disease association in a single experiment. This approach, known as a genome-wide association study (GWAS), uses tagging SNPs to identify markers that may be causally associated with susceptibility. A major advantage of the genome-wide approach is that, unlike the use of candidate genes, it is not dependent upon existing biologic hypotheses and so has the potential to uncover novel, previously unsuspected susceptibility genes and pathways. A drawback is that very large sample sizes (typically comprising thousands of individuals) are needed to provide statistical power to detect disease associations after correction for the large number of polymorphisms tested. Collecting such large sample sizes poses a major challenge for many infectious diseases, particularly diseases with acute presentations in resource-poor countries. Despite this, the recent application of GWASs to selected infectious diseases has been successful in both replicating genes implicated from candidate and linkage approaches and identifying novel susceptibility genes.19
Diseases
Malaria
More genes have been implicated in the differential susceptibility to malaria than to any other disease of humans or other animals (Table 10-2). This in part reflects the early success in identifying the relevance of the sickle hemoglobin polymorphism and the geographic distribution of some malaria resistance alleles.20 Early evidence of differential susceptibility to malaria in nonimmune subjects came from studies of the use of malaria therapy in the management of syphilis.7 Marked differences in susceptibility to the same dose and strain of malaria parasite were observed among individuals. Numerous studies of sickle hemoglobin and glucose-6-phosphate dehydrogenase (G6PD) deficiency provided clear-cut evidence of their protective relevance against Plasmodium falciparum malaria.21,22 Hemoglobin C (HbC),23,24 which is common in parts of West Africa, and HbE,25,26 which is widely distributed in Southeast Asia, are also protective. A few studies have demonstrated protection associated with heterozygosity for β-thalassemia and various α-thalassemia genotypes.27–29
TABLE 10-2
Examples of Genes That Confer Resistance to Plasmodium falciparum Malaria
GENE | VARIANT |
β-Globin | Sickle, thalassemias |
α-Globin | Thalassemias |
Erythrocyte band 3 | 27-bp deletion* |
Duffy chemokine receptor | Promoter variant† |
Glycophorin C | Exon 3 deletion |
Glucose-6-phosphate dehydrogenase | Deficiency variants |
ABO glycosyltransferases | Blood group O |
* Associated with Plasmodium vivax and Plasmodium falciparum resistance.
† Associated with P. vivax resistance.
There have been relatively few useful interpopulation comparisons of malaria susceptibility. However, a study of different ethnic groups in Mali (West Africa), found significant differences in immune responses to P. falciparum and in malaria susceptibility that appear to be genetic in origin.3 The differences could not be explained by the known malaria resistance alleles.
Human leukocyte antigen (HLA) class I and class II alleles were found to influence malaria susceptibility in Africa in large case-control studies.30,31 However, there is some evidence that the particular alleles showing such associations differ among populations. Interpopulation heterogeneity can result from many causes, but a prominent one in malaria is likely to be the marked polymorphism of immunodominant malaria antigens. Indeed, HLA has been found to influence the strain of malaria parasite associated with clinical malaria, and complex interactions among parasite strains may lead to further variability in HLA associations.32 It seems likely that the predominant immune protective mechanisms against malaria vary geographically with transmission patterns.
Malaria parasites vary in their capacity to form rosettes with uninfected erythrocytes and to sequester in capillary beds, and both of these phenotypes have been implicated in increased malaria severity.33,34 Polymorphic host receptors involved in parasite sequestration, such as CD36 and intercellular adhesion molecule 1 (ICAM-1), may influence susceptibility to severe malaria, but findings are conflicting.35–38 ABO blood groups affect the ability of parasitized red cells to rosette, and there is increasing evidence that blood group O is associated with resistance to severe malaria.39
Two large GWASs of severe malaria have been reported to date. The first, performed in the Gambia, replicated the association with sickle hemoglobin as well as identifying putative novel associations with the genes SCO1, encoding a protein involved in cytochrome oxidase function, and DDC, encoding dopa decarboxylase, an enzyme involved in dopamine and serotonin synthesis.40 A second GWAS again confirmed the protective effects of sickle hemoglobin as well as blood group O but also identified two novel associations, with ATP2B4, which encodes the primary calcium pump of erythrocytes, and with a polymorphism on chromosome 16q22 close to the gene MARVELD3, which encodes a tight-junction protein expressed on endothelial cells.41 Future research is expected to shed light on the functional effects of these variants on parasitic infection of erythrocytes and endothelial adherence.
Although most genes that influence susceptibility to infectious disease probably have relatively small effects, a few malaria resistance genes are exceptions. The strength of the protective effect of heterozygosity for HbS is one, with about 90% reduction in the risk of severe malaria.30 The protection from Plasmodium vivax afforded by the Duffy-negative blood group is complete because this parasite is almost always unable to invade Duffy-negative erythrocytes.42 Finally, Melanesian carriers of a 27-bp deletion in the erythrocyte band 3 gene appear to be very strongly protected from P. falciparum,43 maintaining the deletion at frequencies of up to 0.35 even though homozygous fetuses do not survive gestation.44 This is an example of a balanced polymorphism, where the lethal consequences of homozygosity are offset by heterozygote advantage, and such selective pressure leads to deviation of the genotype frequencies from those expected under Hardy-Weinberg equilibrium.
Malaria provides perhaps the best example of population variation in susceptibility and resistance genes. For example, in West Africa, sickle hemoglobin, the A− allele of G6PD, HLA-B53, and an HLA-DR13 allele were associated with protection, but all of these alleles are either rare or absent in Southeast Asia and Melanesia. In the latter regions, HbE, ovalocytosis, other G6PD alleles, and both α- and β-thalassemia are more prevalent.
Mycobacterial Diseases
Genetic susceptibility studies of mycobacterial diseases have been relatively common for several reasons. Familial clustering of leprosy and TB has long been recognized, and leprosy was regarded by some as a genetic disorder before Mycobacterium leprae was identified.1 An accident in Lübeck, Germany, in which children immunized with M. tuberculosis rather than Calmette-Guérin bacillus experienced a range of clinical manifestations, from asymptomatic to severe infection and death, provided early evidence for variable susceptibility to TB.45 This was substantiated by several large twin studies that found higher concordance rates among monozygotic compared with dizygotic twin pairs (see Table 10-1),9 although a reanalysis of the most recent of these studies found less clear evidence of a genetic effect.46 A large twin study of leprosy in India also reported higher concordance rates in monozygotic twins but was inconclusive on the question of genetic susceptibility to leprosy type.11 Observations on the introduction of TB to some populations previously free of the infection suggested that the decline in frequency of the disease over time might in part reflect some natural selection for resistance genes.6 In contrast to malaria, there is evidence that blacks may be more susceptible to infection with M. tuberculosis than whites. The clearest data were obtained in a comparison of rates of skin test conversion among socioeconomically matched nursing home residents in the United States.4 Candidate gene approaches to TB and leprosy susceptibility have implicated a number of genes (Table 10-3), including the HLA region, natural resistance–associated macrophage protein-1 gene (SLC11A1), vitamin D receptor, the signaling adaptor protein Mal/TIRAP (MyD88 adaptor-like protein/Toll–interleukin-1 receptor [TIR] domain adaptor protein), the C-type lectin CD209, and the chemokine macrophage chemoattractant protein-1 (MCP-1/CCL2), although the level of replication between studies has been generally low.47–60,61,62 Genes with larger effects have been sought by genome-wide linkage studies of multicase TB families without clear identification of any major loci.63,64 Similar family studies in leprosy found evidence of linkage to the coregulated genes PARK2 (Parkin 2) and PACRG (Parkin 2 coregulated) on chromosome 6 and to a region encoding the mannose receptor gene on chromosome 10.65,66 Leprosy association studies in particular populations have implicated functional variation in the Toll-like receptor 1 gene (TLR1) and in the gene encoding the cytokine lymphotoxin-α (LTA).67–69
TABLE 10-3
Examples of Susceptibility and Resistance Genes Implicated in Bacterial Diseases and Related Phenotypes
GENE | DISEASE | EFFECT |
HLA-DR-DQ | Leprosy | Susceptibility |
TLR1 | Leprosy | Susceptibility |
NOD2 | Leprosy | Susceptibility |
TNFSF15 | Leprosy | Susceptibility |
RIPK2 | Leprosy | Susceptibility |
IL23R | Leprosy | Susceptibility |
RAB32 | Leprosy | Susceptibility |
ABO (glycosyltransferases) | Cholera | Susceptibility |
MBL | Pneumococcal disease | Susceptibility |
PTPN22 | Pneumococcal disease | Susceptibility |
Mal/TIRAP | Pneumococcal disease, bacteremia, tuberculosis | Resistance |
CFH-CFHR3 | Meningococcal disease | Resistance |
IL1 | Gastric cancer complicating Helicobacter pylori infection | Susceptibility |
CFH, complement factor H; CFHR3, CFH-related protein 3; HLA, human leukocyte antigen; IL1, interleukin-1 (gene); IL23R, interleukin-23 receptor (gene); Mal, myeloid differentiation primary response gene 88 (MyD88) adaptor-like protein; MBL, mannose-binding lectin; NOD2, nucleotide-binding oligomerization domain containing 2; PTPN22, protein tyrosine phosphatase nonreceptor, type 22; RAB32, member RAS oncogene family; RIPK2, receptor-interacting serine-threonine protein kinase 2; TIRAP, Toll-interleukin-1 receptor (TIR) domain adaptor protein; TLR1, Toll-like receptor 1 gene; TNFSF15, tumor necrosis factor (ligand) superfamily member 15.
More recently, GWASs of both TB and leprosy have advanced understanding of their genetic basis. The first GWAS of TB recently reported a susceptibility locus within a gene-poor region on chromosome 18q11.2; the causative variant and its functional consequences are currently unknown, although a regulatory effect on a gene elsewhere on the chromosome is likely.70 More recently, a second GWAS identified a TB resistance locus on chromosome 11p13 in an intergenic region downstream of WT1.71 This gene encodes Wilms tumor 1 and has been shown to interact with the vitamin D receptor, which was previously implicated in TB pathogenesis.
The first GWAS of leprosy susceptibility reported robust associations with polymorphisms in the loci HLA-DR-DQ, RIPK2 (receptor-interacting serine-threonine kinase 2), TNFSF15 (tumor necrosis factor [ligand] superfamily member 15), C13orf31 (chromosome 13 open reading frame 31, recently renamed laccase (multicopper oxidoreductase) domain protein 1, LACC1), CCDC122 (encoding coiled-coil domain protein 122), and NOD2 (nucleotide-binding oligomerization domain containing 2), as well as a weak association with LRRK2 (leucine-rich repeat kinase 2).72 Current understanding of the functions of C13orf31/LACC1 and CCDC122 remains incomplete, but interestingly the other associated loci RIPK2, TNFSF15, and NOD2 as well as TLR1 each encode proteins known to be involved in the innate immune response. A second GWAS of leprosy identified two further susceptibility genes, IL23R and RAB32.73 Genome-wide approaches have been highly successful in the study of leprosy, perhaps reflecting the relatively conserved nature of this pathogen, and these findings may further understanding of the biology of other diseases in addition to leprosy. For example, there is a striking overlap between genes underlying susceptibility to leprosy and Crohn’s disease: NOD2, TNFSF15, LRRK2, C13orf31/LACC1, CCDC122, and IL23R are each associated with Crohn’s disease,74 raising the intriguing possibility of a shared immunologic basis for these two conditions.
Human Immunodeficiency Virus Infection and Acquired Immunodeficiency Syndrome
Studies of cohorts exposed to human immunodeficiency virus (HIV) infection have identified a small proportion of individuals who, despite repeated exposure from infected sexual partners, remain HIV seronegative.75 Some of these resistant sex workers have immunologic evidence of exposure to the virus. There also is clear evidence that individuals vary in the rate of disease progression to acquired immunodeficiency syndrome (AIDS) once infected, and several genes have now been found to influence this rate (Table 10-4).
TABLE 10-4
Examples of Susceptibility and Resistance Genes Implicated in Viral Diseases
GENE | DISEASE | EFFECT |
CCR5 | HIV infection/progression | Resistance |
CCR2 | HIV progression | Resistance |
FUT2 | Norovirus disease | Resistance |
HLA-B, HLA-C | HIV progression | Resistance |
HLA-DP | HBV persistence | Resistance |
IL28B | HCV persistence | Resistance |
MICB | Dengue shock syndrome | Susceptibility |
PLCE1 | Dengue shock syndrome | Susceptibility |
CCR, chemokine receptor; FUT2, fucosyltransferase 2; HBV, hepatitis B virus; HCV, hepatitis C virus; HIV, human immunodeficiency virus; HLA, human leukocyte antigen; IL28B, interleukin-28B; MICB, major histocompatibility complex class I polypeptide–related sequence B; PLCE1, phospholipase C, epsilon 1.
A large number of studies of HLA type in relation to rate of disease progression have been reported. Although there are marked differences among studies, some alleles have now been associated with susceptibility or resistance in more than one population. HLA class I variation is consistently more important than diversity in HLA class II genes, and HLA-B is the most important class I locus.76 HLA-B35 and the HLA-A1-B8-DR3 haplotype have been associated with more rapid disease progression in several studies.77–79 Similarly, HLA-B27 and HLA-B57 are associated with a lower rate of progression.80,81 Particular combinations of HLA class I and II alleles and variants of the transporter associated with antigen processing (TAP) genes have also been implicated.82 Evidence of linkage of the major histocompatibility complex (MHC) to the rate of decline of CD4-positive T cells in patients with AIDS provides support for the relevance of polymorphism in this region.83
It is possible to cluster HLA class I types into so-called supertypes based on the types of peptides bound by particular molecules; analysis of supertypes also shows convincing association with rate of disease progression.84 The HLA type of the host appears to influence the pattern of diversity of HIV sequences that emerges during an infection, indicating that HLA variation can directly influence virus evolution.85 Genome-wide association studies found evidence that loci other than HLA genes within the major histocompatibility complex may affect the rate of disease progression.86,87 A recent, large GWAS of untreated HIV-1 controllers and progressors identified more than 300 significantly associated polymorphisms, all located in the MHC.88 This study extended previous HLA associations to identify HLA types B*57 : 01, B*27 : 05, and B*14 as protective and C*57 as associated with progression to AIDS. Genes encoding the killer cell immunoglobulin-like receptors (KIR), which modulate natural killer cell activity and interact physically with HLA class I molecules, may also interact genetically or epistatically so that KIR gene variants modulate the risk associated with an HLA type.89
The discovery of the role of chemokine receptors (CCRs) as co-receptors with CD4 for viral entry into macrophages and lymphocytes has given rise to numerous studies of genetic variants of these receptors and their ligands. The CCR5 gene associations with resistance to infection and slower disease progression are now well established (see “Chemokine Receptors”),90 and variants in the CCR2 gene have also been associated with altered disease progression.91 More limited data support a possible role for other genes, such as RANTES (regulated on activation, normal T-cell expressed and secreted),92,93 the secretor polymorphism,94 and MCP-195 in susceptibility to infection or disease progression. Copy number variation in the region of the CCL3 (C-C motif ligand 3; also known as macrophage inflammatory protein-1α [MIP-1α]) gene, leading to variable numbers of copies of the CCL3L1 gene, was also reported to associate with HIV disease progression96 perhaps providing a further example, in addition to the α-globin copy number variants, of the impact of structural variation in the genome on infectious disease risk. However, most of the available information on genetic susceptibility comes from studies of susceptibility to clade B virus in North Americans or Europeans, and little is known about genes determining susceptibility in high-prevalence African and Asian populations, where other clade types are prevalent.
Genome-wide association studies of HIV-1 disease phenotypes have replicated the known associations with HLA class I and CCR5-CCR2 but have failed to identify additional major susceptibility genes. Of interest, the combination of HLA class I and CCR5 accounts for only an estimated 23% of observed variability in HIV-1 control.88 The remaining significant variability remains unexplained but may reflect acquired environmental factors, variation in the virus, specific gene–environment interactions, and/or unidentified large-effect genetic variants that are too rare in frequency to be detected using the GWAS approach.
Persistent Hepatitis
The ability or inability to clear hepatitis B virus (HBV) is one of the most striking immunogenetic dichotomies in medicine, with 1% to 12% of infected individuals becoming chronic carriers. Factors such as age of acquisition significantly impact on the likelihood of developing chronic carriage, although evidence suggests that host genetic influences also play a role. One relatively small twin study in Taiwan provided evidence that susceptibility to HBV chronic carriage, but not to HBV infection itself, is genetically determined.12 After early candidate gene studies implicating HLA class I and II genes in HBV clearance and chronic infection, recent GWASs in independent Asian cohorts have clearly established a role for variants in HLA-DPA1 and HLA-DPB1 in the HLA class II region in protection against chronic HBV infection.97–100 The precise mechanism remains unclear, although it may reflect a specific effect on viral clearance after antigen presentation. Spontaneous clearance of hepatitis C virus (HCV) has also been studied using both candidate gene and GWAS approaches, and clear associations have been described with polymorphisms close to the gene IL28B in European and African populations.101,102 Of interest, the same IL28B polymorphism also associates with response to IFN-α treatment of HCV.101,103–106 IL28B encodes IFN-λ3, also referred to as IL-28B, which binds to a different receptor complex than IFN-α but activates the same Janus kinase (JAK)–signal transducer and activator of transcription (STAT) antiviral pathway.107
Other Diseases
Small studies of the FcγRII immunoglobulin receptor, CD32 (FCGR2A), have been undertaken in recurrent bacterial respiratory infection and in systemic meningococcal infection.108,109 The results suggested that alleles encoding histidine at amino-acid position 132, which are associated with greater opsonic activity, may be less frequent in the disease group, although replication in larger studies is required. Studies of invasive pneumococcal disease revealed an almost threefold higher frequency of individuals homozygous for codon changes in the mannose-binding lectin gene (MBL).110,111 A study of children with a variety of infections also found increased frequencies of MBL-deficiency alleles.112 Several other genes are implicated in invasive pneumococcal disease, including the TLR signaling adaptor Mal/TIRAP61; the T-lymphocyte tyrosine phosphatase gene PTPN22, which is also implicated in several autoimmune diseases113; and the genes NFKBIA, NFKBIZ, and NFKBIL2, each of which encodes inhibitors of the proinflammatory transcription factor nuclear factor kappa B (NF-κB).114–116
In a family study of meningococcal disease, children with first-degree relatives who had low TNF and high IL-10 production were more likely to die, suggesting a role for genes regulating production of these cytokines.117 A key role for complement in immune defense against the meningococcus is now well established, and early evidence that Neisseria meningitidis evades complement-mediated killing by binding host complement factor H (CFH) has been supported by both candidate gene and GWASs demonstrating robust associations between CFH polymorphisms and meningococcal susceptibility.118,119 Rare mutations in complement components are also well described in association with recurrent meningococcal disease.120
A family linkage study of Schistosoma mansoni worm burden in Brazil found evidence of linkage to a region of the long arm of chromosome 5.121 This region may also be relevant in Senegalese families and encodes genes for numerous cytokines, including IL-4, IL-9, and IL-13. The same region has been genetically linked to various manifestations of atopy and asthma, consistent with the speculation that a gene selected for resistance to helminthic infections might predispose to asthma or atopy.
Dengue has recently been subject to study from a GWAS perspective: polymorphisms within the MHC region on chromosome 6 and at PLCE1 (phospholipase C, epsilon 1) on chromosome 10 were identified in association with susceptibility to dengue shock syndrome in a study of Vietnamese children.122 The gene responsible for the MHC association signal has not yet been fully defined, although the strongest signal appears to originate from the gene MICB (MHC class I polypeptide-related sequence B), which encodes an inducible activating ligand that is upregulated in human dengue infection123 and activates antiviral immunity by natural killer and CD8+ T cells.124 Further study is required to robustly identify the exact gene underlying this disease association and to explore its role in the pathogenesis of dengue shock syndrome.
A GWAS for susceptibility to visceral leishmaniasis in patients from India and Brazil reported robust association with polymorphisms in the HLA-DRB1-HLA-DQA1 class II region.125 The functional polymorphism within the HLA has not yet been defined, and the extensive linkage disequilibrium across this region makes precise identification of causative variants challenging. Previous linkage and candidate gene studies of leishmaniasis have been underpowered, and their findings were not replicated in this GWAS.
Finally, studies of new-variant Creutzfeldt-Jakob disease (CJD) attributed to infection with the bovine spongiform encephalopathy agent,126,127 sporadic CJD,128 iatrogenic CJD,129 and kuru130,131 have all shown strong associations with variation in the human prion protein (PRNP) gene (see “Other Genes”). The maintenance of this genetic variant over long periods of human evolution has been interpreted as evidence for widespread cannibalism in some early human populations.
Specific Susceptibility and Resistance Genes
For several human genes, there is compelling evidence that one or more genetic variants affect susceptibility to infectious pathogens (see Tables 10-2 to 10-4). Specific genes are discussed in this section.
Blood Groups
ABO blood group associations were investigated in a large number of infectious diseases in early studies. An association of blood group O with increased severity of cholera symptoms was found in several studies.132,133 Blood group O is associated with peptic ulceration, which in turn is connected with H. pylori infection. A possible mechanism for this relationship was suggested by the observation that fucosylation of the Lewis b (Leb) receptor for H. pylori in the gastric mucosa, found in individuals with A or B blood group, impairs binding of the bacteria.134 However, H. pylori infection is not clearly influenced by ABO blood group type.135 Recently, a large study of Africans provided compelling evidence that blood group O associates with reduced risk of severe malaria.39
The ability to secrete blood group substances (such as secretor histo-blood group antigens, HBGA) into saliva and at other mucosal surfaces is genetically determined. Most individuals are secretors, but about 20% of most populations are nonsecretors because of mutation in the fucosyltransferase 2 (FUT2) gene.136 In relatively small studies, nonsecretion was suggested to be associated with susceptibility to some bacterial and fungal infections and with resistance to certain common viral infections.137,138 Nonsecretor status is clearly associated with susceptibility to recurrent urinary tract infection,139 and a possible mechanism for this has been proposed.140 Nonsecretor status was found to protect completely against infection with Norwalk virus in volunteer challenge studies141 and is associated with substantially reduced risk in the general population.142 More recent studies have reported symptomatic norovirus infection in nonsecretors, and in vitro binding studies have demonstrated that binding of noroviruses to HBGA is strain specific, with some norovirus strains evolving different HBGA-binding targets in an attempt to evade genetically determined host resistance.143 However, overall, apart from associations with norovirus and urinary tract infections, there is a need for larger studies to show compelling evidence of association.
The most striking blood group association is of the Duffy blood group with susceptibility to P. vivax malaria. This parasite uses the Duffy blood group antigen as the receptor to invade erythrocytes.144 The Duffy blood group antigen is a promiscuous chemokine receptor. Most sub-Saharan Africans are Duffy blood group negative because of homozygosity for a mutation in the promoter of this gene. They are completely resistant to P. vivax infection. Such individuals also express the Duffy antigen on some other tissues because the promoter mutation that is in the recognition site for an erythroid-specific enhancer is tissue specific.145 It is unclear whether the Duffy genotypes prevented P. vivax from ever entering Africa or whether an earlier and more virulent form of this parasitic infection might have selected the variant in Africa.
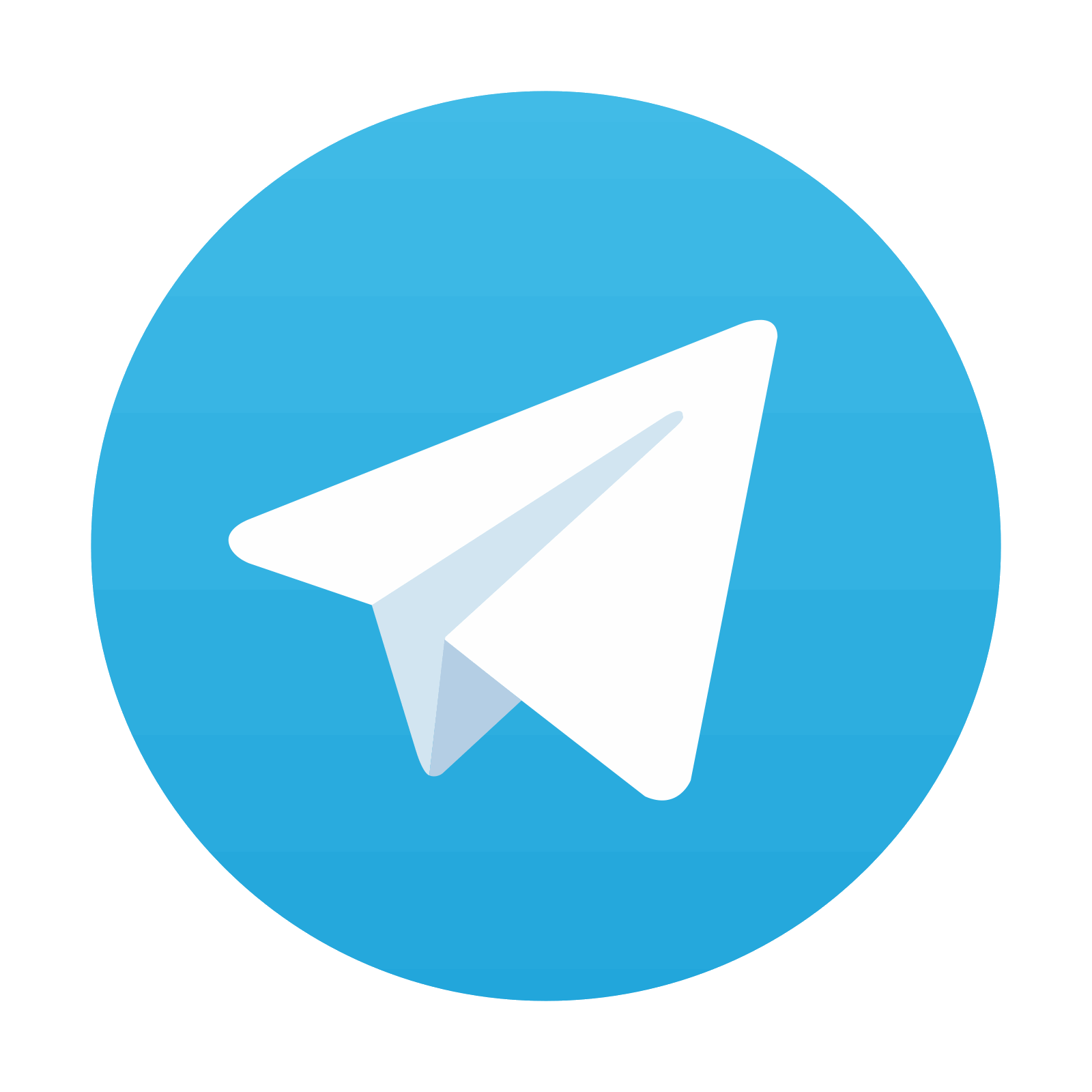
Stay updated, free articles. Join our Telegram channel
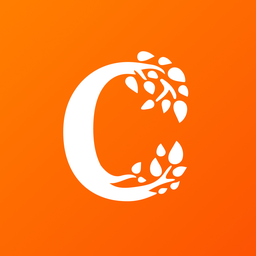
Full access? Get Clinical Tree
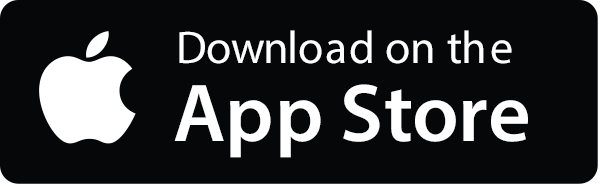
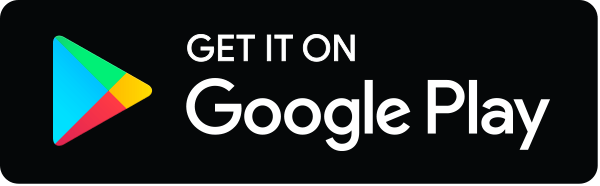