Once released into the circulation by megakaryocytes, circulating platelets can undergo rapid activation at sites of vascular injury and resist unwarranted activation, which can lead to heart attacks and strokes. Historically, the signaling mechanisms underlying the regulation of platelet activation have been approached as a collection of individual pathways unique to agonist. This review takes a different approach, casting platelet activation as the product of a signaling network, in which activating and restraining mechanisms interact in a flexible network that regulates platelet adhesiveness, cohesion between platelets, granule secretion, and the formation of a stable hemostatic thrombus.
Key points
- •
Circulating platelets possess the ability to undergo rapid activation at sites of vascular injury and resist unwarranted activation, which can lead to heart attacks and strokes.
- •
The signaling mechanisms underlying the regulation of platelet activation have been approached as a collection of individual pathways unique to agonist.
- •
This review takes a different approach, casting platelet activation as the product of a signaling network, in which activating and restraining mechanisms interact in a flexible network that regulates platelet adhesiveness, cohesion between platelets, granule secretion, and the formation of a stable hemostatic thrombus.
Introduction: the contribution of platelets to the hemostatic response
Platelets have evolved to be the primary cellular component of the hemostatic response to injury, replacing the nucleated thrombocytes found in most nonmammalian species. After maturing from megakaryocyte-derived proplatelets, platelets circulate in an essentially quiescent state for 10 days in humans, and about half of that in mice. During that time, the quiescent state is maintained by extrinsic regulators such as nitrogen monoxide (NO) and prostacyclin (PGI 2 ) by the presence of healthy endothelium, which separates platelets from activators within the vessel wall; and, perhaps most critically, by the absence of physiologic platelet activators such as collagen, thrombin, and adenosine diphosphate (ADP). Hemostatic thrombi form when the vessel wall has been breached. Pathologic thrombi form in response to vessel wall disease in the arterial system, stasis and inflammation in the venous system, and the inappropriate presence of platelet activators anywhere in the vascular system. Even in a healthy vascular system, differences in local conditions within arteries and veins affect platelet activation. Because pressures and flow rates are higher, hemostasis in the arterial system requires activated platelets to form the initial obstacle to blood loss, accelerate the production of thrombin, and provide a stable base and protected environment in which fibrin can accumulate. However, platelet activation is not limited to the arterial system; it occurs in the venous system and in capillaries as well and contributes to the hemostatic response in those locations. Patients with marked thrombocytopenia develop petechiae because of small leaks in the microcirculation that are otherwise prevented by platelets.
The primary goal of the hemostatic response is to limit blood loss when penetrating injuries occur. Many of the attributes of platelets that allow them to sense and respond rapidly to vascular injury are the same attributes that contribute to heart attacks and strokes when misdirected. The hemostatic response is sometimes modeled as occurring in 3 overlapping phases: initiation, extension, and stabilization. Initiation occurs when moving platelets become tethered to von Willebrand factor (VWF)/collagen complexes within the injured vessel wall and remain in place long enough to become activated by collagen. Extension occurs when additional platelets adhere to the initial monolayer, extending it in the lateral and luminal directions. Locally generated thrombin plus ADP and thromboxane A 2 (TXA 2 ) released by platelets play an important role in this step, activating platelets via G protein–coupled receptors (GPCRs). Subsequent intracellular signaling activates the integrin α IIb β 3 on the platelet surface. Activated platelets stick to each other via bridges formed by the binding of fibrinogen, fibrin or VWF to activated α IIb β 3 . Stabilization refers to the events that help to consolidate the platelet plug and prevent premature disaggregation, in part by amplifying signaling within the platelet. The net result of these 3 phases is a hemostatic plug comprising activated platelets and cross-linked fibrin, a structure stable enough to withstand the forces generated by flowing blood in the arterial circulation.
This model for thinking about the hemostatic response can be helpful, because it emphasizes the initial role of collagen and VWF in anchoring platelets to a site of injury and the subsequent roles of thrombin, ADP, and TxA 2 . Platelets require some form of initial tethering to remain in place long enough to become fully activated. Moving platelets are pushed to the periphery of the blood stream by the flow properties of blood and the presence of red cells at the center of the blood stream. In the first seconds after injury, plasma VWF joins VWF already present in the vascular wall in becoming anchored to collagen. Flow forces working on the anchored VWF expose cryptic binding sites for platelet glycoprotein (GP) Ibα, allowing platelets to become tethered without requiring prior activation. In the absence of functional VWF, platelets can still be activated by collagen, thrombin, ADP, and TxA 2 , but in vivo, they are unable to remain at the site of injury long enough for the initial layers of the hemostatic thrombus to form. Hence, VWF deficiency or dysfunction results in a bleeding disorder, as do disorders affecting secretion. As platelet activation progresses, additional VWF is released locally from platelet storage granules.
However, although useful in some respects, a limitation of thinking about platelet activation as successive waves of initiation, extension, and stabilization is that it does not completely describe what has been observed at sites of injury. Recent studies of platelet activation after penetrating injuries in the microcirculation show that platelet activation is not uniform throughout the hemostatic mass. Instead of a homogeneous mass, there is a core of fully activated, stably adherent platelets overlaid by a shell of less-activated, mostly unstable platelets ( Fig. 1 ). As the response to injury continues, the shell persists even as the stable core expands, suggesting that not all of the platelets associated with the hemostatic response become fully activated. Rather than a homogeneous mass in which all platelets are activated to the same extent and fibrin is dispersed throughout the mass, the hemostatic thrombus retains regional heterogeneity and a hierarchical structure. Fibrin is found primarily in the parts of the thrombus core that are closest to the site of injury. At present, observations such as these are still largely limited to flow chambers and the cremasteric and mesenteric microcirculations in rodent models, but they raise several questions. Do the same events occur when penetrating injuries occur in larger vessels? What normally limits the growth of the hemostatic response and prevents inappropriate vascular occlusion? What goes wrong in pathologic settings such as dyslipidemia or plaque rupture? How is the hemostatic response regulated to produce an optimal outcome?
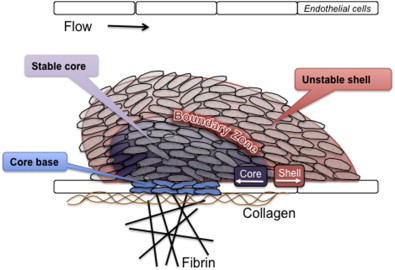
Introduction: the contribution of platelets to the hemostatic response
Platelets have evolved to be the primary cellular component of the hemostatic response to injury, replacing the nucleated thrombocytes found in most nonmammalian species. After maturing from megakaryocyte-derived proplatelets, platelets circulate in an essentially quiescent state for 10 days in humans, and about half of that in mice. During that time, the quiescent state is maintained by extrinsic regulators such as nitrogen monoxide (NO) and prostacyclin (PGI 2 ) by the presence of healthy endothelium, which separates platelets from activators within the vessel wall; and, perhaps most critically, by the absence of physiologic platelet activators such as collagen, thrombin, and adenosine diphosphate (ADP). Hemostatic thrombi form when the vessel wall has been breached. Pathologic thrombi form in response to vessel wall disease in the arterial system, stasis and inflammation in the venous system, and the inappropriate presence of platelet activators anywhere in the vascular system. Even in a healthy vascular system, differences in local conditions within arteries and veins affect platelet activation. Because pressures and flow rates are higher, hemostasis in the arterial system requires activated platelets to form the initial obstacle to blood loss, accelerate the production of thrombin, and provide a stable base and protected environment in which fibrin can accumulate. However, platelet activation is not limited to the arterial system; it occurs in the venous system and in capillaries as well and contributes to the hemostatic response in those locations. Patients with marked thrombocytopenia develop petechiae because of small leaks in the microcirculation that are otherwise prevented by platelets.
The primary goal of the hemostatic response is to limit blood loss when penetrating injuries occur. Many of the attributes of platelets that allow them to sense and respond rapidly to vascular injury are the same attributes that contribute to heart attacks and strokes when misdirected. The hemostatic response is sometimes modeled as occurring in 3 overlapping phases: initiation, extension, and stabilization. Initiation occurs when moving platelets become tethered to von Willebrand factor (VWF)/collagen complexes within the injured vessel wall and remain in place long enough to become activated by collagen. Extension occurs when additional platelets adhere to the initial monolayer, extending it in the lateral and luminal directions. Locally generated thrombin plus ADP and thromboxane A 2 (TXA 2 ) released by platelets play an important role in this step, activating platelets via G protein–coupled receptors (GPCRs). Subsequent intracellular signaling activates the integrin α IIb β 3 on the platelet surface. Activated platelets stick to each other via bridges formed by the binding of fibrinogen, fibrin or VWF to activated α IIb β 3 . Stabilization refers to the events that help to consolidate the platelet plug and prevent premature disaggregation, in part by amplifying signaling within the platelet. The net result of these 3 phases is a hemostatic plug comprising activated platelets and cross-linked fibrin, a structure stable enough to withstand the forces generated by flowing blood in the arterial circulation.
This model for thinking about the hemostatic response can be helpful, because it emphasizes the initial role of collagen and VWF in anchoring platelets to a site of injury and the subsequent roles of thrombin, ADP, and TxA 2 . Platelets require some form of initial tethering to remain in place long enough to become fully activated. Moving platelets are pushed to the periphery of the blood stream by the flow properties of blood and the presence of red cells at the center of the blood stream. In the first seconds after injury, plasma VWF joins VWF already present in the vascular wall in becoming anchored to collagen. Flow forces working on the anchored VWF expose cryptic binding sites for platelet glycoprotein (GP) Ibα, allowing platelets to become tethered without requiring prior activation. In the absence of functional VWF, platelets can still be activated by collagen, thrombin, ADP, and TxA 2 , but in vivo, they are unable to remain at the site of injury long enough for the initial layers of the hemostatic thrombus to form. Hence, VWF deficiency or dysfunction results in a bleeding disorder, as do disorders affecting secretion. As platelet activation progresses, additional VWF is released locally from platelet storage granules.
However, although useful in some respects, a limitation of thinking about platelet activation as successive waves of initiation, extension, and stabilization is that it does not completely describe what has been observed at sites of injury. Recent studies of platelet activation after penetrating injuries in the microcirculation show that platelet activation is not uniform throughout the hemostatic mass. Instead of a homogeneous mass, there is a core of fully activated, stably adherent platelets overlaid by a shell of less-activated, mostly unstable platelets ( Fig. 1 ). As the response to injury continues, the shell persists even as the stable core expands, suggesting that not all of the platelets associated with the hemostatic response become fully activated. Rather than a homogeneous mass in which all platelets are activated to the same extent and fibrin is dispersed throughout the mass, the hemostatic thrombus retains regional heterogeneity and a hierarchical structure. Fibrin is found primarily in the parts of the thrombus core that are closest to the site of injury. At present, observations such as these are still largely limited to flow chambers and the cremasteric and mesenteric microcirculations in rodent models, but they raise several questions. Do the same events occur when penetrating injuries occur in larger vessels? What normally limits the growth of the hemostatic response and prevents inappropriate vascular occlusion? What goes wrong in pathologic settings such as dyslipidemia or plaque rupture? How is the hemostatic response regulated to produce an optimal outcome?
The platelet signaling network: the forest and the trees
The molecular mechanisms that underlie the hemostatic response have been the subject of intense scrutiny for many years, reflecting the relevance of this process to clinical medicine and the lives of humans. To the extent that space allows, the remainder of this article summarizes what has been learned. However, before diving into the trees, it is worth considering the forest. Largely for experimental reasons, it has generally been more practical to tease apart platelet activation 1 molecule at a time. Studies with genetically modified mice have helped this process considerably, even although mouse platelets turn out to be different from human platelets in several critical ways. The results make it possible to draw models such as the one in Fig. 2 , which summarizes key steps in platelet activation. Knowledge of platelet signaling pathways has aided the development of several antiplatelet agents that have entered clinical practice or are in clinical trials.
However, Fig. 2 has important limitations. One limitation is that it has little to say about the events that lead to granule secretion in activated platelets. Resting platelets store and activated platelets secrete numerous molecules that support platelet activation and the hemostatic response. Some of these molecules also help to drive events such as wound healing and angiogenesis. Patients lacking storage granules have a clinical bleeding disorder. The presence of both proangiogenic and antiangiogenic factors within platelet storage granules has fueled recent debates about differential selectivity in either the storage or the release of these molecules in vivo.
A second limitation of models such as the one shown in Fig. 2 is that it falls short in answering questions that are critical for understanding platelet activation. Why does platelet activation require such complexity? Why are there so many platelet agonists and so many signaling pathways? If they are merely redundant, why does it seem that genetic or pharmacologic ablation of so many individual molecules has a meaningful effect on platelet function? An answer to some of these questions may come from thinking about signaling networks rather than signaling pathways.
One way of illustrating such networks is shown in Fig. 3 . In a network-centric view of platelet activation, instead of responding to a single agonist via a single signaling pathway, platelets accumulating at a site of injury respond to the mixture of inputs (agonists) to which they are exposed. This mixture varies over both time and space, creating agonist concentration gradients. Positive and negative feedback in the form of soluble molecules (some released by platelets, others by damaged cells) plus contact-dependent interactions between adjacent platelets play an important role in modulating platelet reactivity. Information flow within the platelet sums the effects of these inputs and nodal points in the signaling network provide links between them. This view of platelet activation can be tied to the structure shown in Fig. 1 by considering, first, how declining gradients of different platelet agonists originating near the point of injury differentially affect the platelet activation state as the distance from the point of injury grows and, second, how the closer proximity of platelets in the thrombus core facilitates the binding of cell surface ligands to receptors on the surface of adjacent platelets.
Normal platelet activators in vivo
Once vascular injury has occurred, platelets are principally activated by locally exposed collagen, locally generated thrombin, platelet-derived TXA 2 , and ADP that has either been released from damaged cells or secreted from platelet dense granules. The flow pattern of circulating red cells facilitates platelet adhesion to collagen by pushing platelets closer to the vessel wall, allowing GPIbα on the platelet surface to be snared by the A1 domain of VWF bound to collagen. Human platelets express 15,000 to 25,000 copies of GPIbα in a multiprotein complex with GPIbβ, GPIX, and GPV. Mutations in GPIbα that prevent its expression on the platelet surface or impair its receptor function produce an inherited recessive bleeding disorder (Bernard-Soulier syndrome) because platelet adhesion to the vessel wall is impaired. Macrothrombocytopenia arises in this disorder in part from a failure to form the normal linkages between GPIbα complex and filamin in the platelet membrane cytoskeleton.
Once platelets are captured at the damaged vessel wall, the primary drivers for platelet activation are collagen, thrombin, ADP, TXA 2 , and, to a more limited extent, epinephrine (see Fig. 2 ). With the exception of collagen, each of these agonists signals through 1 or more members of the GPCR superfamily. Properties that are common to this class of receptors make them well suited for their tasks in platelets. Most bind their ligands with high affinity and, because they act as exchange factors, each occupied receptor can theoretically activate multiple G proteins, amplifying the initial signal. Binding studies show that agonist GPCRs are expressed on the platelet surface in low numbers, ranging from a few hundred (ADP and epinephrine) to a few thousand (thrombin) per cell. The duration of GPCR signaling is subject to receptor internalization, receptor desensitization, and the accelerated inactivation of G proteins by members of the RGS (regulator of G protein signaling) family. This multiplicity of mechanisms allows for tight regulation of platelet activation.
Human platelets express at least 10 heterotrimeric G proteins, including at least 1 G s , 4 G i (G i1 , G i2 , G i3 and G z ), 3 G q family members (G q , G 11 and G 16 ), and 2 G 12 family members (G 12 and G 13 ). Much has been learned about the critical role of these G proteins by knocking out the genes encoding their α subunits in mice. Less is known about the G βγ isoforms that are expressed in platelets and the selectivity (if any) of their individual contributions to platelet activation.
Most of the time agonist-initiated platelet activation begins with the activation of a phospholipase C (PLC) isoform, which by hydrolyzing membrane phosphatidylinositol-4,5-bisphosphate (PIP 2 ) produces the second messenger (inositol-1,4,5-trisphosphate [IP 3 ]) needed to increase the cytosolic Ca 2+ concentration. This situation leads to integrin activation via an intracellular pathway that includes a Ca 2+ -dependent exchange factor (CalDAG-GEF), a switch (predominantly Rap1b), an adaptor (RIAM), and proteins that interact directly with the integrin cytosolic domains (kindlin and talin). Which isoform of PLC is activated depends on the agonist. Collagen activates PLCγ 2 using a mechanism that depends on scaffold molecules and protein tyrosine kinases. Thrombin, ADP, and TxA 2 activate PLCβ using G q as an intermediary. The α subunit of G q binds directly to the phospholipase, activating it.
It is the binding of bivalent fibrinogen to α IIb β 3 that enables platelets to stick to one another, forming an aggregate. Other proteins that can substitute for fibrinogen include fibrin, VWF, and fibronectin. Average expression levels of α IIb β 3 range from approximately 50,000 per cell on resting platelets to 80,000 on activated platelets. Mutations in α IIb β 3 that prevent its expression or suppress its function produce a bleeding disorder (Glanzmann thrombasthenia) because platelets are unable to form stable aggregates. Antiplatelet agents such as eptifibatide (Integrilin), tirofiban (Aggrastat), and abciximab (ReoPro) take advantage of this situation by blocking α IIb β 3 .
Platelet Activation by Collagen
Under static conditions, collagen can activate platelets without the assistance of cofactors, but under arterial flow conditions, VWF plays an essential role. Collagen polymers are better platelet agonists than collagen monomers. Platelets can adhere to monomeric collagen but require the more complex structure found in fibrillar collagen for optimal activation. Four collagen receptors have been identified on human and mouse platelets. Two bind directly to collagen (integrin α 2 β 1 and GPVI); the other 2 (α IIb β 3 and GPIbα) bind to collagen via VWF ( Fig. 4 ). Of these receptors, GPVI is the most potent signaling receptor. The structure of the GPVI extracellular domain places it in the immunoglobulin superfamily. Its ability to generate signals rests on its constitutive association with the immunoreceptor tyrosine-based activation domain (ITAM)-containing Fc receptor γ-chain (FcRγ). Platelets from mice that lack either GPVI or FcRγ have impaired responses to collagen, as do platelets in which GPVI has been depleted or blocked. Loss of FcRγ impairs collagen responses in part because of loss of a necessary signaling element and in part because of its role in helping GPVI reach the platelet surface. α 2 β 1 supports adhesion to collagen and acting as a source of further signaling after engagement. Human platelets with reduced expression of α 2 β 1 have impaired collagen responses, as do mouse platelets that lack β 1 integrins when the ability of these platelets to bind to collagen is tested at high shear.
Signaling through GPVI can be studied in isolation with the snake venom protein, convulxin, or with synthetic collagen-related peptides, both of which bind to GPVI, but not to other collagen receptors. Each of these entities causes clustering of GPVI, leading to the phosphorylation of FcRγ by Src family tyrosine kinases constitutively associated with a proline-rich domain in GPVI. Phosphorylation creates an ITAM motif recognized by the tandem SH2 domains of the tyrosine kinase Syk. Association of Syk with the GPVI/FcRγ-chain complex activates Syk, produces signaling complexes based on the scaffold proteins LAT and SLP-76, and leads to the activation of PLCγ 2 . Loss of Syk impairs collagen responses. PLCγ 2 hydrolyzes PIP 2 to form IP 3 and diacylglycerol (DAG). IP 3 opens Ca 2+ channels in the platelet dense tubular system, increasing the cytosolic Ca 2+ concentration through passive efflux. Depletion of the Ca 2+ stores within the dense tubular system triggers Ca 2+ influx across the platelet plasma membrane. The changes in the cytosolic Ca 2+ concentration that occur when platelets adhere to collagen under flow can be visualized in real time. Diacylglycerol activates the more common protein kinase C (PKC) isoforms that are expressed in platelets, allowing the serine/threonine phosphorylation events that are needed for platelet activation.
Collectively, collagen receptors support the capture of fast-moving platelets at sites of injury, cause activation of the captured platelets, and stimulate the cytoskeletal reorganization that allows the previously discoid platelets to flatten out and adhere more closely to the exposed vessel wall. As noted earlier, VWF supports this process. It increases the density of potential binding sites for collagen per platelet, because the number of copies of GPIbα and α IIb β3 greatly exceeds the number of copies of GPVI and α 2 β 1 . Because VWF is highly multimeric, it also increases the number of binding sites per collagen molecule. This situation increases the likelihood that platelets encounter an available binding site and, once bound, are able to increase the number of contacts with collagen by bringing additional receptors into play. GPVI and GPIbα are able to bind collagen and VWF without prior platelet activation, but once activation begins, α 2 β 1 and α IIb β 3 are able to bind their respective ligands as well. Some of the integrin-activating signaling occurs downstream of GPVI, but there is evidence that the GPIb-IX-V complex can signal as well, as can α 2 β 1 and α IIb β 3 once they are engaged.
Platelet Activation by Thrombin
Thrombin is able to activate platelets at concentrations as low as 0.1 nM. Although other platelet agonists can also cause phosphoinositide hydrolysis, none seems to be as efficiently coupled to PLC as thrombin. Within seconds of the addition of thrombin, the cytosolic Ca 2+ concentration increases 10-fold, triggering downstream Ca 2+ -dependent events, including the activation of phospholipase A 2 and integrin activation via the Rap1b-mediated pathway. All of these responses, but not shape change, are abolished in platelets from mice lacking G qα . Thrombin also activates Rho in platelets, leading to rearrangement of the actin cytoskeleton and shape change, responses that are greatly reduced or absent in mouse platelets that lack G 13α . Thrombin is able to inhibit adenylyl cyclase activity in human platelets, either directly (via a G i family member) or indirectly (via released ADP).
Platelet responses to thrombin are mediated by members of the protease-activated receptor (PAR) family of GPCRs. There are 4 members of this family, 3 of which (PAR1, PAR3, and PAR4) can be activated by thrombin. PAR1 and PAR4 are expressed on human platelets; mouse platelets express PAR3 and PAR4. Receptor activation occurs when thrombin cleaves the extended N terminus of each of these receptors, exposing a new N terminus that serves as a tethered ligand. Synthetic peptides based on the sequence of the tethered ligand domain of PAR1 and PAR4 are able to activate the receptors, mimicking at least some of the actions of thrombin. PAR3 was originally identified after gene ablation studies showed that platelets from mice lacking PAR1 were still fully responsive to thrombin. Approximately half of PAR1 –/– mice die in utero, but this seems to be caused by loss of receptor expression in the vasculature, rather than in platelets. Whereas human PAR3 has been shown to signal in response to thrombin, PAR3 on mouse platelets primarily serves to facilitate PAR4 cleavage. Activation of PAR4 requires higher concentrations of thrombin than are required for activation of PAR1, apparently because it lacks the hirudinlike sequences that can interact with the anion-binding exosite of thrombin and facilitate receptor cleavage. Kinetic studies in human platelets suggest that thrombin signals first through PAR1 and subsequently through PAR4.
Considerable evidence shows that PAR family members are sufficient to activate platelets. Peptide agonists for either PAR1 or PAR4 cause platelet aggregation and secretion. Conversely, simultaneous inhibition of human PAR1 and PAR4 abolishes responses to thrombin, as does deletion of the gene encoding PAR4 in mice. However, there are differences between mice and humans: optimal thrombin responses in human platelets require both PAR1 and PAR4, whereas those in mouse platelets are mediated by PAR3-facilitated cleavage of PAR4. PAR1 and PAR4 are coupled to at least G q and G 13 . There is conflicting evidence about whether G i -dependent signaling in thrombin-activated platelets is entirely mediated by secreted ADP or occurs in part by a direct interaction between PARs and G i2 . However, given the ability of PAR1 to directly couple to G i2 in cells other than platelets, it is likely that both occur. This hypothesis is consistent with a recent observation that expression of an RGS-resistant variant of G i2α enhances thrombin responses in mouse platelets, even when the contribution of secreted ADP is blocked. On the other hand, a requirement for PAR family members does not preclude the involvement of other participants in platelet responses to thrombin. GPIbα has a high affinity thrombin binding site within residues 268 to 287. Deletion or blockade of this site reduces platelet responses to thrombin, particularly at low thrombin concentrations, and impairs PAR1 cleavage on human platelets. PAR1 antagonists have been developed and are undergoing clinical trials. The early results show that blocking PAR1 may have some clinical usefulness, but can also increase bleeding risk in patients receiving other antiplatelet agents.
Platelet Activation by ADP
ADP is stored in platelet dense granules and released on platelet activation. It is also released from damaged cells at sites of vascular injury, serving as a stimulus for activating tethered platelets and stabilizing the hemostatic plug. Aggregation studies show that all of the other platelet agonists are dependent to some extent on released ADP to elicit maximal platelet aggregation, although this dependence varies with the agonist and is dose related. Drugs that block platelet ADP P2Y 12 receptors have proved to be effective antiplatelet agents despite the fact that ADP, when added alone, is a less potent platelet agonist than thrombin.
When added to platelets in vitro, ADP causes an increase in cytosolic Ca 2+ , TXA 2 formation, protein phosphorylation, shape change, aggregation, and secretion. It also inhibits cyclic adenosine monophosphate (cAMP) formation. These responses are half-maximal at approximately 1 μM ADP. However, even at high concentrations, ADP is a comparatively weak activator of PLC. Instead, its usefulness as a platelet agonist rests more on its ability to activate other pathways. Human and mouse platelets express 2 distinct receptors for ADP, denoted P2Y 1 and P2Y 12 . Both receptors are members of the purinergic class of GPCRs. P2Y 1 receptors couple to G q . P2Y 12 receptors couple to G i family members. Optimal activation of platelets by ADP alone requires activation of both receptors, but the potentiation by ADP of platelet responses to other agonists seems to be mainly caused by P2Y 12 . Knockouts of P2Y 1 and P2Y 12 in mice produce effects consistent with those predicted by pharmacologic studies on human platelets. A third purinergic receptor on platelets, P2X 1 , is an adenosine trisphosphate (ATP)-gated Ca 2+ channel. Platelet dense granules contain ATP as well as ADP, and studies suggest that there are conditions in which P2X 1 activity is essential for platelet activation. P2X 1 is also functional on megakaryocytes.
When P2Y 1 is blocked or deleted, ADP is still able to inhibit cAMP formation, but its ability to cause an increase in cytosolic Ca 2+ , shape change, and aggregation is greatly impaired, as it is in platelets from mice that lack G qα . P2Y 1 –/– mice have a minimal increase in bleeding time and show some resistance to thromboembolic mortality after injection of ADP, but no predisposition to spontaneous hemorrhage. Primary responses to platelet agonists other than ADP are unaffected and when combined with serotonin, which is a weak stimulus for PLC in platelets, ADP can still cause aggregation of P2Y 1 –/– platelets. Taken together, these results show that platelet P2Y 1 receptors are coupled to G qα and responsible for activation of PLC. P2Y 1 receptors can also activate Rac and the Rac effector, p21-activated kinase (PAK), but do not seem to be coupled to G i family members.
P2Y 12 was independently identified by 2 groups. As had been predicted by inhibitor studies and by the phenotype of a patient lacking functional P2Y 12 , platelets from P2Y 12 –/– mice do not aggregate normally in response to ADP. P2Y 12 –/– platelets retain P2Y 1 -associated responses, including shape change and PLC activation, but lack the ability to inhibit cAMP formation in response to ADP. The G i family member associated with P2Y 12 seems to be primarily G i2 , because platelets from G i2α −/− mice have an impaired response to ADP, whereas those lacking G i3α or G zα do not. Conversely, expression of a G i2α variant that is resistant to the inhibitory effects of RGS proteins produces a gain of function in mouse platelets stimulated with ADP. Absence of P2Y 12 produces a hemorrhagic phenotype in humans, albeit a mild one. Deletion of either P2Y 1 or P2Y 12 in mice prolongs the bleeding time and impairs platelet responses not only to ADP but also to thrombin and TXA 2 , particularly at low concentrations. Because the receptors for thrombin and TXA 2 can cause robust activation of PLC, the contribution of ADP when thrombin or TXA 2 are present seems to be largely because of its ability to activate G i2 . Downstream effectors for G i2α include Src family members as well as PI3 kinase and Rap1b.
Platelet Activation by TXA 2
TXA 2 is produced from arachidonate in platelets by the aspirin-sensitive cyclooxygenase 1 (COX-1) pathway. When added to platelets in vitro, stable TXA 2 analogues such as U46619 cause shape change, aggregation, secretion, phosphoinositide hydrolysis, protein phosphorylation, and an increase in cytosolic Ca 2+ and have little, if any, direct effect on cAMP formation. Similar responses are seen when platelets are incubated with exogenous arachidonate. TXA 2 can diffuse across the plasma membrane and activate nearby platelets. Like secreted ADP, release of TXA 2 amplifies the initial stimulus for platelet activation and helps to recruit additional platelets. This process is effective locally, but is limited by the brief (∼30 second) half-life of TXA 2 in solution, helping to confine the spread of platelet activation to the original area of injury.
Only 1 gene encodes TXA 2 receptors, but 2 splice variants are produced that differ in their cytoplasmic tails. Human platelets express both. Loss of G qα abolishes U46619-induced IP 3 formation, but does not prevent shape change, which can be blocked by knocking out G 13α . Although in cells other than platelets, TXA 2 receptors have been shown to couple to G i family members, in platelets the inhibitory effects of U46619 on cAMP formation seem to be largely mediated by secreted ADP. These observations have previously been interpreted to mean that platelet TXA 2 receptors are coupled to G q and G 12/13 , but not to G i family members. However, the enhanced response observed in mouse platelets carrying an RGS protein-resistant G i2α variant suggests that this is still an open issue.
The biological relevance of TXA 2 to normal platelet function is supported by genetic and pharmacologic evidence. TP −/− mice have a prolonged bleeding time. Their platelets are unable to aggregate in response to TXA 2 agonists and show delayed aggregation with collagen, presumably reflecting the role of TXA 2 in platelet responses to collagen. A group of Japanese patients with impaired platelet responses to TXA 2 analogues have proved to be either homozygous or heterozygous for an R60L mutation in the first cytoplasmic loop of TP. However, the most compelling case for the contribution of TXA 2 signaling in human platelets comes from the successful use of aspirin as an antiplatelet agent. When added to platelets in vitro, aspirin abolishes TXA 2 generation. Aspirin also blocks platelet activation by arachidonate and impairs responses to thrombin and ADP. The defect in thrombin responses appears as a shift in the dose/response curve, indicating that TXA 2 generation is supportive of platelet activation by thrombin, but not essential.
Platelet Activation by Epinephrine
Compared with thrombin, epinephrine is a weak activator of human platelets when added on its own. Nonetheless, there are reports of human families in which a mild bleeding disorder is associated with impaired epinephrine-induced aggregation and reduced numbers of catecholamine receptors. Epinephrine responses in platelets are mediated by α 2A -adrenergic receptors. In both mice and humans, epinephrine is able to potentiate the effects of other agonists so that the combination is a stronger stimulus for platelet aggregation than either agonist alone. Potentiation is often attributed to the ability of epinephrine to inhibit cAMP formation, but as is discussed later, there are clearly other effects as well. In contrast to other platelet agonists, epinephrine has no detectable direct effect on PLC and does not cause shape change, although it can trigger phosphoinositide hydrolysis indirectly by stimulating TXA 2 formation. Taken together, these results suggest that platelet α 2A -adrenergic receptors are coupled to G i , but not G q or G 12 family members. Human and mouse platelets express 4 members of the G i family: G i1 , G i2 , G i3 , and G z . Of these members, G zα has the slowest rate of intrinsic guanosine triphosphate (GTP) hydrolysis and is the only one that is not a substrate for pertussis toxin. Knockout studies show that epinephrine responses in platelets are abolished when G zα is deleted. Loss of G i2α or G i3α has no effect. G z also seems to be responsible for the ability of epinephrine to activate Rap1b. Therefore, it seems that in mouse platelets, α 2A -adrenergic receptors couple to G z , but not G i2 or G i3 .
Critical events
Thus far, this review has focused on platelet activation mechanisms from the perspective of individual agonists, many of which trigger a common set of pathways. In this section, some of those pathways are considered.
Phosphoinositide Hydrolysis
With the exception of epinephrine, platelet activation begins with the activation of PLC which, by hydrolyzing membrane phosphatidylinositol-4,5-bisphosphate (PIP 2 ), produces IP 3 and (DAG), the second messengers needed to increase the cytosolic Ca 2+ concentration and activate some of the PKC isoforms found in platelets. As already noted, how PLC is activated varies with the agonist. Collagen activates PLCγ 2 using adaptor molecules and tyrosine kinases. Thrombin, ADP, and TXA 2 activate PLCβ isoforms using G qα and (less efficiently if at all) G βγ derived from G i . Regardless of which PLC isoform is activated, the subsequent increase in the Ca 2+ concentration triggers downstream events, including integrin activation and TXA 2 formation. Thrombin provides a robust stimulus for phosphoinositide hydrolysis and causes the largest and fastest increase in cytosolic Ca 2+ . Collagen and ADP are more dependent on the synthesis and release of TXA 2 to achieve a maximal response.
Cytosolic Ca 2+ and Integrin Activation
Resting platelets maintain their cytosolic free Ca 2+ concentration at approximately 0.1 μM by (1) limiting Ca 2+ influx and (2) pumping Ca 2+ out of the cytosol across the plasma membrane or into the dense tubular system (smooth endoplasmic reticulum). This action consumes ATP. In activated platelets, [Ca 2+ ] i can spike 10-fold to greater than 1 μM with potent agonists like thrombin. Measurements made of large populations of platelets suggest that this increase occurs rapidly and uniformly in response to potent agonists such as thrombin and less potently with agonists such as ADP and collagen. However, observations made with single platelets suggest that the Ca 2+ response is heterogeneous, occurring to a different extent in different platelets, with some showing spiking behavior rather than a sustained increase and some not responding at all. The molecular basis of this heterogeneity is not fully understood, although simulation studies suggest that platelet size is a factor.
The increase in cytosolic Ca 2+ that occurs during platelet activation derives from 2 sources. The first part is caused by the IP 3 -mediated release of Ca 2+ from the platelet dense tubular system. The second part occurs when depletion of the dense tubular system Ca 2+ pool triggers store operated Ca 2+ entry through a conformational change in STIM1, a protein in the dense tubular system membrane, binding of STIM1 to Orai1 in the plasma membrane, allowing an influx of plasma Ca 2+ . These events can be separated by chelating extracellular Ca 2+ , thereby precluding Ca 2+ influx. The increase in [Ca 2+ ] i activates the GTP-binding protein, Rap1b, via the Ca 2+ -dependent guanine nucleotide exchange factor (GEF), CalDAG-GEF. Activated Rap1b has been shown to bind to RIAM, bringing it to the plasma membrane, where it can bind talin. This situation allows talin to bind to the cytoplasmic domain of α IIb β 3 , triggering integrin activation and exposing a binding site for fibrinogen.
TXA 2 Production
The initial events of platelet activation lead to the activation of phospholipase A 2 , which cleaves phosphatidylcholine and other membrane phospholipids, liberating arachidonate from the C2 position of the glycerol backbone. Arachidonate can be transformed into many bioactive compounds, but in platelets, TXA 2 is the key product. TXA 2 synthesis begins with COX-1, which forms prostaglandin (PG) G 2 and PGH 2 from arachidonate in a 2-step process (see Fig. 4 ). The PGH 2 is then metabolized to TXA 2 by thromboxane synthetase. Evidence suggests that phospholipase A 2 activation in platelets can occur in more than 1 way. It can clearly happen in response to an increase in cytosolic Ca 2+ , because the addition of a Ca 2+ ionophore is sufficient to cause phospholipase A 2 activation. There is also evidence that mitogen-activated protein kinase pathway signaling activates phospholipase A 2 , although not necessarily by direct phosphorylation of phospholipase A 2 . TXA 2 formation is promoted by platelet aggregation and may, therefore, also occur as a consequence of integrin signaling, although this has not been tested directly. TXA 2 can diffuse out of the platelet, activating nearby receptors in an autocrine or paracrine fashion before it is hydrolyzed to inactive TXB 2 . Even although TXA 2 is a potent platelet activator, its half-life in aqueous solution is short (about 30 seconds), which has implications for both its duration of action and its impact downstream from a growing thrombus. This situation may be especially relevant for the model of the hemostatic response shown in Fig. 1 , which postulates that declining gradients of soluble platelet agonists originating in the thrombus core contribute to the heterogeneity in platelet activation that we and others have observed within the hemostatic mass.
Shape Change and Rearrangement of the Actin Cytoskeleton
Activated platelets lose their characteristic discoid shape, either assuming a globular shape with filopodial extensions if they are activated in suspension or flattening out and developing lamellopodia if they are activated on a suitable surface. Shape change reflects loss of the circumferential microtubule ring and rearrangement of the actin cytoskeleton of the platelet, a process mediated by monomeric G proteins in the Rho and Rac families. When platelets in suspension are activated by soluble agonists, shape change precedes platelet aggregation. The soluble agonists (thrombin, ADP, and TXA 2 ) that trigger shape change typically act through receptors that are coupled to members of the G q and G 12 families. Epinephrine is unable to cause shape change, because its receptors are coupled solely to G z .
At least 2 pathways are involved in the reorganization of the actin cytoskeleton: Ca 2+ -dependent activation of myosin light-chain kinase downstream of G q family members and activation of Rho family members downstream of G 13 . Several proteins having both G α -interacting domains and GEF domains can link G 12 family members to Rho family members, including p115RhoGEF. With the exception of ADP, shape change persists in platelets from mice that lack G qα but is lost when G 13α expression is suppressed, alone or in combination with G 12α . A combination of inhibitor and genetic approaches suggests that G 13 -dependent Rho activation leads to shape change via pathways that include the Rho-activated kinase (p160ROCK) and LIM kinase. Activation of these kinases results in phosphorylation of myosin light-chain kinase and cofilin, helping to regulate both actin filament formation and myosin. ADP, on the other hand, depends more heavily on G q -dependent activation of PLC to produce shape change and is able to activate G 13 only as a consequence of TXA 2 generation; hence, the loss of ADP-induced shape change when G q signaling is suppressed.
PI3 Kinase Activation
An additional category of critical events needed for robust platelet activation involves activation of the PI3-kinase (PI3K) isoforms expressed in platelets, either by G i family members (PI3Kγ) or by phosphotyrosine-dependent signaling pathways downstream of collagen receptors (PI3Kαβδ). PI3-kinases phosphorylate PI-4-P and PI-4,5-P 2 to produce PI-3,4-P 2 and PI-3,4,5-P 3 , respectively. Among the best-described consequence of PI3K activation in platelets is the activation of the protein kinase, Akt. Knockout and inhibitor studies show that all 3 Akt isoforms are necessary for normal platelet activation. Knockout and inhibitor studies also show a G i /PI3K-dependent mechanism for activating Rap1b, which, given the clinical usefulness of P2Y 12 antagonists as antiplatelet agents, seems to be a mechanism that is important for stabilizing platelet aggregates. However, aside from Rap1b and GSK3β, a kinase the activity of which is negatively regulated by Akt, little is known about Akt-dependent signaling pathways in platelets. To our knowledge, the Akt-dependent GEF for Rap1b has yet to be identified. PI3Kβ has been proposed as a target for antiplatelet agents.
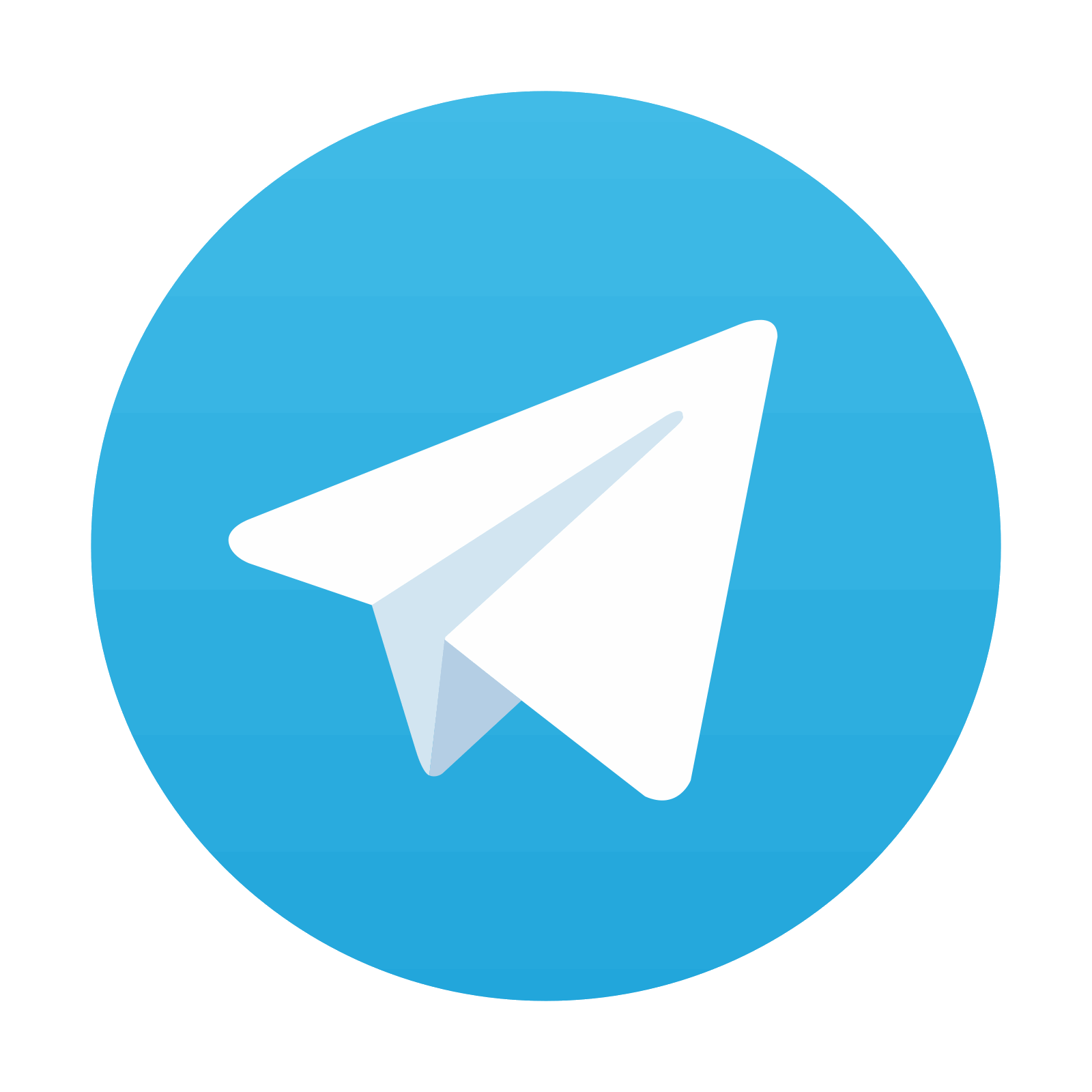
Stay updated, free articles. Join our Telegram channel
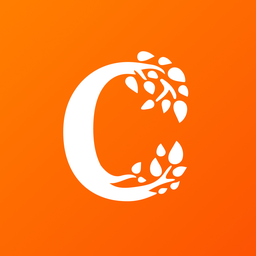
Full access? Get Clinical Tree
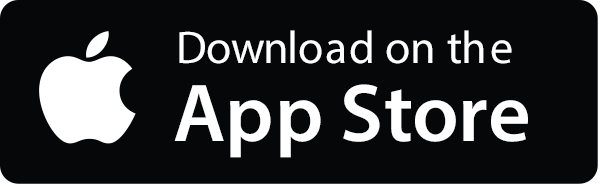
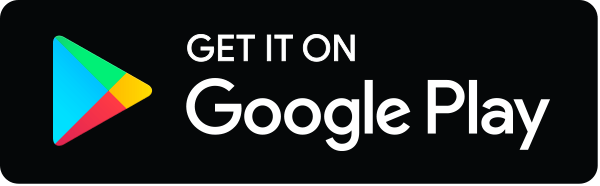