results. Sometimes, the mutated proteins are not properly produced, secreted into the blood, or located in the physiologic site. When they do circulate in substantial amounts, the variant protein often circulates with impaired function. When a single base change is phenotypically silent, this is commonly referred to as a single nucleotide polymorphism (SNP). Outside the actual coding region, it is even more likely that nucleotide substitutions are silent. Notable exceptions are mutations in binding sites for transcription factors that regulate gene activity, mutations that affect splicing of the immature RNA, and mutations that may alter the stability of the RNA.
50% of patients, genetic risk factors such as heterozygosity for mutations in coagulation inhibitors or cofactors are found.16
Table 10.1 Characteristics of human coagulation factor genes | ||||||||||||||||||||||||||||||||||||||||||||||||||||||||||||||||||||||||||||||||||||||||||||||||||||||||||||||||||||||||||||||||||||||||||||||||||||||||||||||||||||||||||||||||||||||||||||||||||||||||||||||||||||||||||||||||||||||||||||||||||||||||||||||||||||||||||||||||||||||||||||||||||||||||
---|---|---|---|---|---|---|---|---|---|---|---|---|---|---|---|---|---|---|---|---|---|---|---|---|---|---|---|---|---|---|---|---|---|---|---|---|---|---|---|---|---|---|---|---|---|---|---|---|---|---|---|---|---|---|---|---|---|---|---|---|---|---|---|---|---|---|---|---|---|---|---|---|---|---|---|---|---|---|---|---|---|---|---|---|---|---|---|---|---|---|---|---|---|---|---|---|---|---|---|---|---|---|---|---|---|---|---|---|---|---|---|---|---|---|---|---|---|---|---|---|---|---|---|---|---|---|---|---|---|---|---|---|---|---|---|---|---|---|---|---|---|---|---|---|---|---|---|---|---|---|---|---|---|---|---|---|---|---|---|---|---|---|---|---|---|---|---|---|---|---|---|---|---|---|---|---|---|---|---|---|---|---|---|---|---|---|---|---|---|---|---|---|---|---|---|---|---|---|---|---|---|---|---|---|---|---|---|---|---|---|---|---|---|---|---|---|---|---|---|---|---|---|---|---|---|---|---|---|---|---|---|---|---|---|---|---|---|---|---|---|---|---|---|---|---|---|---|---|---|---|---|---|---|---|---|---|---|---|---|---|---|---|---|---|---|---|---|---|---|---|---|---|---|---|---|---|---|---|---|---|---|---|---|---|---|---|---|---|---|---|---|---|---|---|---|---|
|
Table 10.2 Genetic models of thrombosis and hemostasis | ||||||||||||||||||||||||||||||||||||||||||||||||||||||||||||||||||||||||||||||||||||||||||||||||||||||||||||||||||||||||||||||||||||||||||||||||||||||||||||
---|---|---|---|---|---|---|---|---|---|---|---|---|---|---|---|---|---|---|---|---|---|---|---|---|---|---|---|---|---|---|---|---|---|---|---|---|---|---|---|---|---|---|---|---|---|---|---|---|---|---|---|---|---|---|---|---|---|---|---|---|---|---|---|---|---|---|---|---|---|---|---|---|---|---|---|---|---|---|---|---|---|---|---|---|---|---|---|---|---|---|---|---|---|---|---|---|---|---|---|---|---|---|---|---|---|---|---|---|---|---|---|---|---|---|---|---|---|---|---|---|---|---|---|---|---|---|---|---|---|---|---|---|---|---|---|---|---|---|---|---|---|---|---|---|---|---|---|---|---|---|---|---|---|---|---|---|
|
bleeding phenotype. Mutations associated with this phenotype include frameshift, splice site, promoter, and missense mutations, with the total number of mutations now reaching more than 110.76 The situation is less clear for mutations associated with measurable levels of factor VII activity, as many are asymptomatic.
sequence and two partial deletions of the factor VIII gene were described.140 The finding of four distinctly different mutations in the factor VIII gene of otherwise similar patients was the first hint that a large variety of mutations exist in the factor VIII gene in patients with hemophilia.
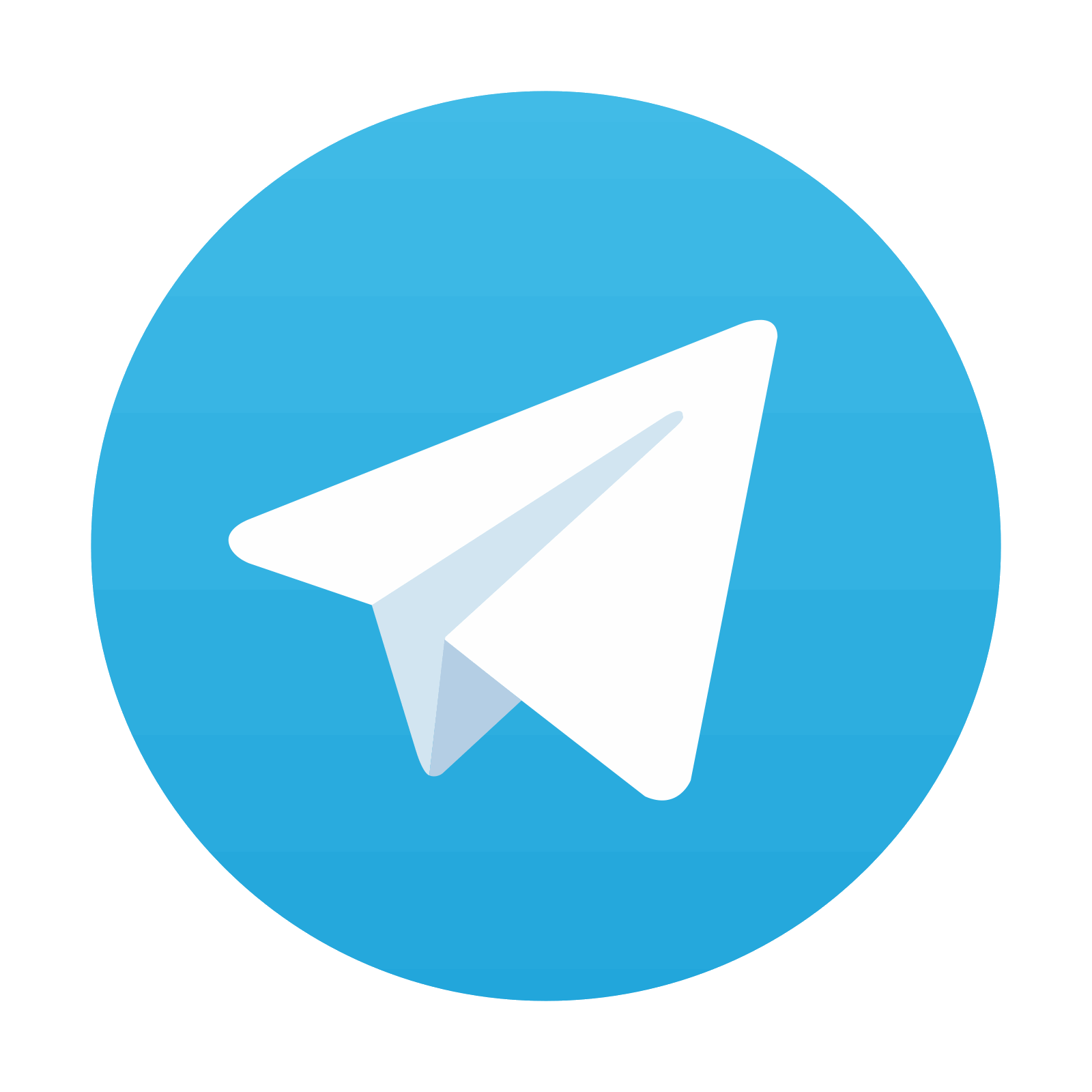
Stay updated, free articles. Join our Telegram channel
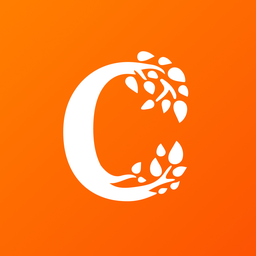
Full access? Get Clinical Tree
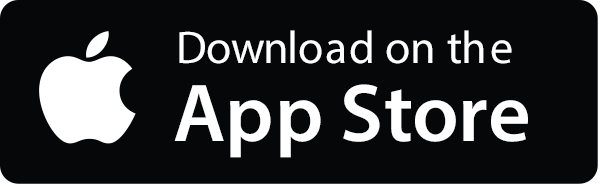
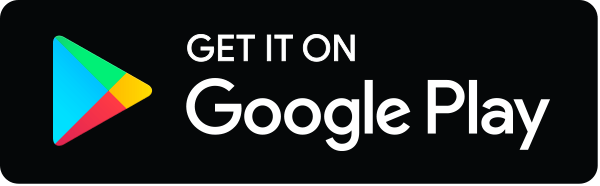
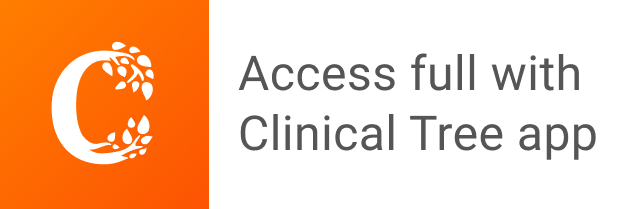