Gene symbol
Pathway
Tumors in which DNA methylation occurs
Familial involvement
BRCA1
DNA repair
Breast, ovarian
Breast, ovarian
CDH1
Cell adhesion
Diffuse gastric, lobular breast
Diffuse gastric, lobular breast
CDKN2A (p16)
Cell-cycle
Widespread includes non-Hodgkin’s lymphoma, gastric, melanoma
Melanoma
DAPK1
Apoptosis
Chronic lymphocytic leukemia, colon, lung, gastric
Chronic lymphocytic leukemia
LKB1
Signal transduction
Colon, lung
Peutz–Jeghers syndrome
MLH1
DNA repair, apoptosis
Colon, endometrium, ovarian
Lynch syndrome
(colon, endometrium, ovarian)
RB1
Cell cycle
Retinoblastoma, breast
Retinoblastoma
VHL
Angiogenesis
Renal, chronic lymphocytic leukemia
von Hippel–Lindau syndrome (renal)
A recent interesting discovery is that some of these driver genes may show constitutional DNA methylation—methylation of a given gene may occur in multiple somatic tissues (often in a mosaic pattern)—in normal individuals. The individual is then predisposed to develop characteristic cancers similar to the spectrum seen when this driver gene is mutated in familial cancer syndromes [142].
Besides the hereditary cancer genes, a large variety of genes that affect every facet of the cancer phenotype are subject to DNA methylation (a large list is given in ref. [148]). In addition to protein-coding genes, hypermethylation can occur at CpG islands associated with non-protein-coding genes, such as microRNAs, which are frequently dysregulated in cancer [149]. It has been estimated in genome-wide studies, that each cancer can harbor several hundreds of aberrantly methylated CpG islands [95, 150].
It is remarkable how many of the DNA methylation changes in cancer occur in genes that are plausibly involved in cancer, although this probably reflects the preponderance of candidate genes that have been studied. It is however certain that many of the genes that are reported to be methylated in cancer are not directly involved in the initiation or progression of the cancer as they are tissue-specific genes such as CALCA and MYOD1 that are not expressed in the normal cells from which the cancer arose.
Many of the genes that become methylated in cancer are restricted to certain types of tumors. In some cases, such as GSTP1 in prostate cancer, DNA methylation of the gene is virtually a hallmark of the cancer. However, for most cancer types DNA methylation of no one single gene is predominant. However, profiling DNA methylation over a large panel of genes reveals patterns that are characteristic of certain types of cancer [151]. Thus, different cancer types can be discriminated by their distinct DNA methylation pattern (epigenetic fingerprint) [150, 152]. Many research groups are trying to identify panels of genes that best characterize subgroups of each major cancer as biomarkers for that cancer [153].
Other methylated genes may be passenger genes (also known as bystander genes). Passenger genes may become aberrantly methylated because they are part of an epigenetically silenced domain. Also, genes that are methylated in cancer may be methylated in the cell of origin of the tumor and escape demethylation. Other genes may become methylated because of aging related incursions of DNA methylation into normally unmethylated regions which then become further methylated in the altered DNA methylation milieu of the cancer [154].
The extent to which a gene promoter region has to be methylated to result in silencing or decreased gene expression is still unknown. DNA methylation patterns are often heterogeneous and show variable degrees of methylation throughout the region analyzed [155–157]. In addition, the amount of DNA methylation of a given promoter tends to increase with cancer evolution [158, 159]. It has been reported that the CDKN2B (p15) promoter CpG island needs to be methylated at 30–40 % of the CpG sites for silencing to occur [160]. It is expected that the minimal DNA methylation level is dependent on the gene and its genomic context.
Two mechanisms have been suggested to explain local promoter associated CpG island hypermethylation in cancer [15, 161]. One mechanism is based on random seeding of DNA methylation marks by an aberrant DNA methylation machinery. The second mechanism proposes that certain regions are prone to aberrant DNA methylation, either intrinsically or by a misrouted DNA methylation machinery. This may also explain DNA hypomethylation in these cases. However, neither concept can entirely explain aberrant DNA methylation levels and patterns.
5.2.2 Factors Influencing Methylation Changes in the Epigenome
The accumulation of alterations to the normal epigenome (epimutations) can be a step in cancer development analogous to the accumulation of DNA sequence changes (mutations). The underlying causes of and contributions to alterations to the epigenome remain largely elusive. Nevertheless, there is strong evidence, that the aging process and nutritional history have an impact on DNA methylation as well as changes in histones and chromatin structure [162–164]. These parameters are not easily investigated separately and are usually interwoven with other parameters, such as environmental exposures, which may also influence the epigenome alterations to a certain extent [163, 165, 166]. For example, heavy metals (e.g., nickel and arsenic) [167] as well as toxins (e.g., aflatoxin B1) [120] are well recognized substances capable of altering the epigenome.
Aging is associated with a global loss of DNA methylation as well as a local increase in DNA methylation of usually unmethylated CpG islands in normal tissues [168]. Initially, it was reported that the CpG island-associated with the estrogen receptor (ER) gene showed increasing level of DNA methylation in colorectal mucosa samples in an age dependent manner [169]. Subsequently, multiple other genes were shown to display age-related DNA methylation in normal colorectal mucosa [154, 170, 171]. Aberrant expression of DNMT1, DNMT3a, and DNMT3b may be responsible for deregulated DNA methylation patterns in aging cells [172–174]. In cultured human fibroblasts, DNMT1 expression decreases during the aging process and this is probably associated with global DNA hypomethylation [175]. Furthermore, the increased expression of the DNMT3b gene may lead to aberrant DNA methylation at single-locus genes [175].
The genetic background can also determine susceptibility to alterations cellular DNA methylation . The one-carbon pathway is involved in the synthesis of the methyl group donor, S-adenosyl-l-methionine (SAM), which is utilized by DNMTs to establish DNA methylation. In particular, variants in two members of this pathway, MTHFR (5,10 methylenetetrahydrofolate reductase) and MS (methionine synthase), influence the 5-methylcytosine content of the cell.
TT homozygous and CT heterozygous individuals of the MTHFR variant C677T were shown to cause a genome-wide reduction of DNA methylation level in normal tissue [176–178]. The C to T substitution leads to thermolabile MTHFR protein, with reduced catalytic activity [179]. The AA homozygotes of a second MTHFR variant, A1298C, were also associated with a lower content of DNA methylation [176, 180]. This MTHFR protein variant shows also a decreased enzymatic activity [181]. GG homozygotes at the A2756G variant of the MTR gene (coding for 5-methyl tetrahydrofolate-homocysteine methyltransferase) had less methylation at tumor suppressor genes in their cancers than the other genotypes [178].
Genetic variation in promoter regions may also alter their propensity to become methylated with time. The T allele of the common germ-line polymorphism c.-56C > T (rs16906252) in the promoter region of the MGMT gene is associated with aberrant DNA methylation at this locus in colorectal cancer [182] and malignant mesothelioma [183]. Furthermore, gain of DNA methylation in normal tissues such as colonic mucosa [184] and peripheral blood [185] is also correlated with the presence of the T allele. So far, the underlying mechanism for this predisposition is unknown. As not every individual with the T allele has detectable methylation, and the amount of methylation is highly variable, trans-acting factors must also be involved. Susceptibility for aberrant DNA methylation associated with single nucleotide polymorphisms seems to be widespread and the importance of this in cancer predisposition remains to be fully understood [186, 187].
5.2.3 CpG Island Methylator Phenotype (CIMP)
The CpG island methylator phenotype (CIMP) has been widely used to describe cancers with extensive CpG island methylation. This phenotype was first proposed in 1999 for colorectal cancers [188]. Cancers are scored as CIMP-positive if the majority of a small panel of CpG promoter regions are methylated in a manner analogous to the scoring for microsatellite instability. Analysis of a large panel of CpG islands has subsequently confirmed that CIMP is a distinct phenotype in colorectal cancer [189].
Classifying cancers as CIMP-positive is not always straightforward. Alongside the original gene panel of CDKN2A, MINT1, MINT2, MINT31, and MLH1 [188], several other gene panels have been proposed. Weisenberger et al. [189] chose a panel of CACNA1G, IGF2 (a non-imprinted CpG island at this locus), NEUROG1, RUNX3, and SOCS1, while Ogino et al. recommended a minimal panel of RUNX3, CACNA1G, IGF2, and MLH1 [190]. In addition to the different gene panels, different methods have been used to detect DNA methylation. Quantitative approaches are superior to qualitative approaches (methylation-specific PCR) as they allow for the identification and subsequent exclusion of genes with low-level DNA methylation, which are probably biologically not significant [191]. Further difficulties in defining CIMP-positive cancers arise by using different CIMP cut off values [192, 193] and different CIMP grading systems [189, 192, 194].
Despite the nonstandardized use of different marker panels and methodologies, CIMP-high colorectal cancers share multiple clinicopathological and molecular characteristics. CIMP-high colorectal cancers are usually proximally located [188, 195–197] and are associated with an older patient age [193, 196]. They often exhibit microsatellite instability as the DNA mismatch repair gene MLH1 is frequently methylated [193, 195–198]. CIMP cancers that are methylated for MLH1 have a different profile of genetic changes to those that are not methylated for MLH1 [195]. They are mainly TP53 wild-type [193, 195, 197].
CIMP cancers show a very high mutation frequency in the BRAF gene (V600E) [189, 191, 194–196, 199, 200]. The occurrence of CIMP and the oncogenic BRAF mutation has been linked to the serrated pathway of colorectal carcinogenesis. The BRAF mutation and CIMP are observed in over 75 % of sessile serrated adenomas which are precursor lesions associated with the serrated neoplasia pathway [194]. Hereditary non-polyposis colorectal cancers caused by germ-line mutations in the DNA mismatch repair genes MLH1, MSH2, MSH6, or PMS2 [201] do not belong to the serrated neoplasia pathway and do not show either CIMP or BRAF mutations.
A significant problem in understanding CIMP is that the mechanism underlying the phenotype in colorectal cancer has not been identified. Existing theories encompass a genetic and/or environmental cause [202, 203]. An interesting hypothesis raises the possibility that chemotherapy with DNA damaging agents might drive or select for cancers associated with CIMP [204]. It is also important to note that in melanoma, the BRAF V600E mutation does not appear to be associated with high levels of aberrant promoter methylation [205].
Since the first report of CIMP in colorectal cancers, other solid tumors and leukemias have been suggested to be CIMP-positive [205, 206]. It is unclear whether the drivers of CIMP in these cancers are related to the drivers of CIMP in colorectal cancer. In glioma, it has been shown that mutation of a single gene, isocitrate dehydrogenase 1 (IDH1), establishes the CIMP phenotype but this is unlikely to drive more than a subset of CIMP-positive colorectal cancers [207].
5.2.4 Histone and Chromatin Changes in Cancer
Repressive histone marks and DNA methylation are often found together [208]. However, the contribution of DNA methylation and histone modifications to transcriptional regulation varies from gene to gene. Whereas for certain genes, DNA methylation plays the predominant role, the expression for other gene loci may depend more on the presence of histone modifications.
Cancer cells also exhibit mechanisms of silencing, which are independent of promoter-associated DNA methylation. The establishment of trimethylated H3-K27 histone marks by the polycomb group protein EZH2, was shown to be the major cause for silencing certain genes, such as ESR1 and PGR, as observed for the PC3 prostate cancer cell line. Most of the genes bearing this histone mark were found not to be associated with the presence of DNA methylation, whereas genes harboring DNA methylation did not show the trimethylated H3-K27 histone modification [209].
Two examples of genes that are silenced without DNA methylation are CDKN1A (p21 CIP1/WAF1 ) and gelsolin (GSN). Expression of the cell cycle kinase inhibitor CDKN1A is frequently induced after treatment of various cell lines with HDAC inhibitors [210]. The treatment renders the CDKN1A gene open to transcription, at least in part, by increasing the amount of acetylation of histones H3 and H4 at this locus [211]. Gelsolin, an actin binding protein, is also often upregulated after treatment with HDAC inhibitors in cell lines [212–214]. Altered DNA methylation seems to play only a minor role in GSN gene expression [213].
Many of the different enzymes involved in histone-modifying and chromatin-modifying activities have been shown to be deregulated in cancer. Aberrant expression, mutations and translocations are frequently the cause for their malfunction in cancer. Good overviews and the presentation of some examples in greater detail are provided in the literature [215–217].
Chromatin remodeling complexes such the SWI/SNF complex can modulate transcriptional activity and alterations in these complexes are associated with cancer development [218, 219]. SMARCB1 (SNF5/INI1) codes for a key component of the SWI/SNF chromatin remodeling complex. This gene is frequently deleted or mutated and its malfunction is thought to be the underlying cause for rhabdoid tumors, a rare pediatric cancer [220]. Interestingly, these cancers do not show genomic instability and it seems that the disruption of the epigenetic machinery can substitute for genetic alterations [221].
5.2.5 Long-Range Epigenetic Silencing (LRES)
The concept of aberrant long-range epigenetic silencing (LRES) originated from genetic studies in model organisms of events such as position effect variegation where de novo silencing occurs along a length of chromatin after it is translocated next to heterochromatin [222]. Studies of gene expression in cell culture systems also revealed cases where adjacent genes underwent coordinate changes in gene expression [223, 224]. An important example of LRES in normal development is the inactive X-chromosome in females which is silenced virtually across its entire length. It shows all the hallmarks of silencing, methylation of promoter region associated CpG islands, heterochromatic appearance and late replication of its DNA. It is considered that the DNA methylation appears after the initial silencing to lock the silent phenotype into place [225].
De novo LRES in cancer was first described in colon cancer [226]. The first chromosomal region found to be silenced by LRES spans 4 Mb and comprises three distinct segments of contiguous CpG islands on chromosome 2q14 associated with cancer-specific DNA hypermethylation of EN1, SCTR, and INHBB. These segments were flanked by CpG islands, which were unmethylated in cancer cells as well as normal cells. Importantly, regardless of the CpG island methylation status, the whole region was associated with the repressive histone mark dimethylated H3-K9, leading to transcriptional suppression of the entire region [226]. Since then, several studies showed LRES in other cancer types. LRES affects large chromosomal regions of approximately 100 kb up to 5 Mb and results in silencing of several contiguous genes. LRES functionally resembles large genetic deletions resulting in silencing of long stretches DNA with the important exception that LRES may be reversible [161].
Another LRES region was found in bladder cancer on chromosome 3p22 and comprises 130 kb [227]. This region encompasses four contiguous genes (VILL, PLCD1, DLEC1, and ACAA1) that are coordinately downregulated in cancer cells. Again, the cancer-specific inactivation is characterized by the occurrence of trimethylated H3-K9 in the absence of DNA methylation. This same region is a part of a 1.1 Mb LRES region in the majority of microsatellite-unstable colorectal cancers associated with aberrant MLH1 DNA methylation [228]. This segment comprises the genes AB002340, EPM2AIP, MLH1, LRRFIP2, GOLGA4, ITGA9, CTDSPL, PLCD1, and DLEC1, most of which show methylated promoter-associated CpG islands and the H3-K9 dimethylation repressive histone mark) . Interestingly, LRRFIP2, GOLGA4, and CTDSPL escape aberrant DNA methylation but despite being associated with an active chromatin structure are also transcriptionally repressed in cancer samples.
LRES is not restricted to a particular cancer type and many different regions throughout the genome are subject to LRES [229–231]. Indeed, LRES is now recognized as a common event in cancer, affecting multiple regions throughout the compromised cancer cell genome. Genome-wide studies in prostate cancer [232] and neuroblastoma [233] revealed the presence of 47 and 63 cancer-related LRES clusters, respectively. Interestingly, the neuroblastoma study suggested that LRES regions are more often located at chromosome ends [233].
5.2.6 Is Cancer a Genetic Disease or an Epigenetic Disease?
That cancer is a genetic disease is an often repeated truism. However, our current knowledge of epigenetics must make us raise the question of whether cancer is as much or more of an epigenetic than a genetic disease [234–236]. The most compelling evidence for the latter argument is that at least some cancer cells can be reprogrammed into taking part in normal differentiation despite the presence of activated oncogenes [237]. The number of epigenetic changes in a cancer genome also greatly outnumbers the number of genetic changes in a cancer genome [235, 238] although deciphering which of these changes are critical in cancer development remains a challenge. Sequencing of the entire genome of one case of acute myeloid leukemia (AML ) with a normal karyotype revealed that only very few mutations were present and suggested that the changes driving the carcinogenic process in this case were principally epigenetic [239]. Intriguingly, it was subsequently discovered that one of these mutations was a premature stop codon in the DNMT3a gene [240]. Indeed, some of the strongest evidence for an epigenetic basis to cancer comes from the leukemias where one of the fusion partners in a leukemogenic translocation often codes for an epigenetic modifier. In many cases, the translocation serves to maintain the leukemic cells in a stem-like or progenitor-like state. Chief among these fusion partners is the MLL gene which is involved in numerous translocations [241]. MLL has diverse functions including the establishment of trimethylated H3-K4 histone marks [242].
It is ultimately futile to put genetic and epigenetic mechanisms in opposition to each other as alternate hypotheses for cancer development as they both play pivotal roles and are often interrelated [133, 243]. Silencing of DNA repair genes will establish a mutator phenotype which will accelerate the rate of mutational change and provide tumor evolution with additional raw material [244]. For example, silencing of the promoter of the DNA repair gene MLH1 results in microsatellite instability which further results in mutations at a variety of genes which have an effect on the cancer cell phenotype [240, 245].
On the other hand, the underlying causes of epigenetic instability or methylator phenotypes such as CIMP may arise from mutations in an as yet unidentified gene. Mutations in oncogenes such as RAS have been reported to destabilize the epigenetic machinery and initiate DNA methylation changes in the cell [246, 247]. In FOS-transformed cells, it was noted that DNMT1 protein expression was elevated resulting in a 20 % increase of cellular levels of 5-methylcytosine. DNMT1 seemed moreover to play a fundamental role in FOS transformation as blocking of DNMT1 gene expression resulted in reversion of transformation [248]. This is consistent with other reports that blocking DNMT1 gene expression can suppress tumorigenesis [249, 250].
A deregulated histone code and altered chromatin remodeling can also lead to increased mutation rates by altering the accessibility of the DNA repair machinery to the genomic DNA. Proper acetylation of H3-K56 in mammals is important to prevent spontaneous DNA damage probably during DNA replication [251]. Therefore, this histone modification participates in maintaining genomic stability and in an appropriate DNA damage response. In yeast, disrupted H3-K56 acetylation sensitizes the cells to compounds that cause DNA strand breaks during replication [252]. Impaired access to certain damage sites or an impaired DNA damage response will further contribute to the genomic instability of the cell, which further promotes its dysregulation.
Aberrant DNA methylation can also target components of the epigenetic machinery. The RIZ1 (PRDM2) promoter is frequently silenced by DNA methylation in several cancer types [253–257]. RIZ1 is one of the two different proteins encoded by the RIZ (Retinoblastoma protein interacting zinc finger gene) gene. RIZ1, which contains a SET related PR domain, acts as a histone methyltransferase, which methylates H3-K9 [258]. RIZ is a common target in cancer as loss of heterozygosity, frameshift mutations and missense mutations also contribute to genetic alterations of the RIZ gene [258, 259].
5.3 Epigenetic Changes as Tools for Diagnostics and Targets for Therapy
5.3.1 DNA Methylation-Based Biomarkers
DNA methylation has aroused considerable interest because it generates large numbers of potential biomarkers [260]. Biomarkers fall into several classes. Some are useful because they are present in nearly all cancers of a given type and therefore can be used for disease detection and monitoring. These do not necessarily play a key role in cancer pathogenesis. Others are prognostic biomarkers that predict patient outcome. One of the key advantages of DNA methylation-based biomarkers is that methylation is stable after biopsies are removed. This stability gives it a major advantage over RNA-based expression biomarkers [260].
Methodology is a critical issue when locus-specific DNA methylation is used as a biomarker. DNA methylation assays should be used, which provide a quantitative or at least a semiquantitative result. In many cases, they need to be highly sensitive as well. Although, methylation-specific PCR (MSP) is a widely applied method to detect DNA methylation [261], the results obtained by this method are not quantitative. Methods for quantitative DNA methylation measurement have been based on bisulfite pyrosequencing [262–264], MethyLight [265], or SMART-MSP [266]. A useful semiquantitative approach is given by the methylation-sensitive high-resolution melting (MS-HRM) methodology [267]. It must also be considered that quantitative interpretation can be compromised by the presence of heterogeneous DNA methylation [268].
5.3.2 Early Detection and Monitoring of Cancers After Therapy
The early detection and treatment of most cancer types is considered the best prerequisite for a good outcome. Various body fluids or excretions, such as blood, sputum, saliva, urine, and stool are relatively noninvasive samples, which can potentially be used for the early detection of cancer. In other cases, ductal lavages or bronchial washes can be used. Because of the relatively low frequency of cancers in the groups being screened, even assays with high specificity are likely to produce more false-positives than cancer cases. Thus, it is important to target such screening to individuals already at high risk of developing a certain type of cancer.
Detection of aberrant promoter DNA methylation of the SEPT9 gene in plasma samples was shown to be correlated to the presence of colorectal cancer [269, 270] and precancerous colorectal lesions [271]. This epigenetic biomarker is relatively specific for colon cancer which shows SEPT9 methylation level differences dependent on disease staging.
DNA methylation of the PITX2 promoter, which regulates the expression of transcript variants A and B was shown to be a predictive epigenetic biomarker in breast cancer. DNA hypermethylation of PITX2 was associated with an increased risk of recurrence in node-negative, steroid hormone-receptor positive breast cancer patients treated by adjuvant tamoxifen monotherapy after surgery [272, 273]. The presence of PITX2 methylation was also associated with poor patient outcome for node-negative, steroid hormone-receptor positive breast cancer without any systemic adjuvant therapy and node-positive, estrogen receptor-positive, HER-2/neu-negative breast cancer treated by adjuvant anthracycline-based chemotherapy, respectively [274, 275]. Furthermore, methylation of PITX2 is also a prognostic biomarker in prostate cancer. Increased methylation levels were associated with a higher risk of clinical relapse in patients with prostate cancer after radical prostatectomy [276, 277].
The GSTP1 gene encodes for the pi class glutathione S-transferase enzyme, which is involved in cellular detoxification processes. The presence of DNA methylation at the GSTP1 associated CpG island was detected in more than 90 % of prostate cancer and about 70 % of high-grade prostatic intraepithelial neoplasia [278, 279]. On the other hand, DNA methylation of GSTP1 is rare in benign biopsies and normal prostate tissue. GSTP1 methylation appears to be an early event in cancer progression and could be used as a diagnostic biomarker [280, 281]. Furthermore, methylation of GSTP1 in serum of prostate cancer patients was associated with an elevated risk of clinical relapse after surgery [282].
5.3.3 Targeting Individual Epigenetic Changes—Epigenetics and Personalized Medicine
Biomarkers that predict patient responses to particular therapies are critical to personalized therapy. While considerable attention has been focused on gain and loss of function mutations in oncogenes, epimutations because of their greater frequency are likely to determine or influence therapeutic response.
Currently, cancer is usually treated according to the presumed tissue of origin of the cancer. This approach probably works because similar cancer subsets share similar profiles of genetic and epigenetic changes. Nevertheless, there are intrinsic subtypes within each cancer grouping as has been shown by large-scale gene expression studies [283, 284]. Among cancers that are similar in both clinicopathological features and molecular profiles, there is still heterogeneity when individual genes are examined; each cancer is thus a unique entity. As alterations in the expression or function of many of the individual genes can predict the responses to particular therapies, there will be considerable scope for using this molecular information to guide the treatment of the patient. When epigenetic changes in cancers lead to the silencing of genes and where the gene products are involved in sensitivity or resistance to therapeutics, the DNA methylation status of key genes can act as a predictive biomarker. Thus, the information we can extract from the altered epigenome of a cancer will enable us to understand how to treat the cancer. This has been termed pharmacoepigenomics by analogy to pharmacogenetics.
The DNA repair machinery is repeatedly the target of epigenetic change in cancer [244] as it often also is in cancer predisposition syndromes. This machinery is responsible to repair the multiple forms of damage, which is constantly inflicted upon the genome by endogenous (e.g., replication errors, reactive oxygen species) and exogenous sources (e.g., radiation). Many enzymes and proteins participate directly or indirectly in the important processes to repair and maintain a stable genome. Altering or silencing the gene expression of a given DNA repair gene will result in unrepaired damage setting up a mutator phenotype. The mutator phenotype generates multiple sequence variants. Natural selection then acts on the variants that arise, enabling cancer evolution. However, the repair deficiency is also a therapeutic target in the cancer. This underlies the susceptibility of many cancers to one or other form of chemotherapy. Currently, the best example of a gene whose DNA methylation has predictive value is the MGMT gene [285]. Its protein product, O 6 -methylguanine DNA methyltransferase, directly removes alkyl groups from the O 6 -position of guanine residues. If the alkylation damage is left unrepaired, it increases the probability of a G to A transition mutation during replication [286]. Thus, a failure in repairing this damage will lead to an accumulation of mutations over time and constant development of new cell clones in the cancer.
The presence of methylation at the MGMT promoter was shown to function as a DNA methylation-based biomarker for the susceptibility of cancers treated therapeutically with alkylating agents [287]. The therapeutic benefit of alkylating agents for cancer patients with a methylated MGMT promoter was clearly demonstrated in the treatment of glioblastoma with temozolomide [288]. The presence of DNA methylation at the MGMT promoter was also associated with increased survival in diffuse large B-cell lymphoma (B-DLCL) patients being treated with the alkylating drug cyclophosphamide as part of multidrug regimens [289]. Methylation of the MGMT gene is increasingly being used to guide the choice of whether to use alkylating agents in the treatment of glioblastoma patients. This has led to MGMT promoter methylation being the first DNA methylation-based biomarker assay that is being adopted in cancer molecular pathology for glioblastoma. However, MGMT promoter methylation is not currently used to guide treatment decisions for other tumors such as melanoma and colorectal cancer that also can show methylation at the MGMT promoter.
Many assays have been developed to detect DNA methylation at the MGMT promoter utilizing different approaches [267, 290, 291]. In addition, several commercial products are available based on quantitative methylation-specific PCR (qMSP) , bisulfite pyrosequencing and methylation-sensitive high-resolution melting (MS-HRM) . It is critical that quantitative approaches are used as, as previously mentioned, certain individuals have the single nucleotide polymorphism that predisposes to a definite background level of DNA methylation but not one that definitely leads to a methylated cancer [185].
Other DNA repair genes, such as MLH1 [143, 292], WRN [293], as well as members of the BRCA-Fanconi anemia pathway, BRCA1 [146, 294], FANCC [295], FANCF [296], and FANCL [295], also have been reported to show aberrant DNA methylation in several cancer types. These different alterations render cancer cells susceptible to certain chemotherapeutic drugs either directly [297, 298] or through a synthetic lethal approach [299]. Thus knowledge of which of these genes are methylated may help clinicians to choose the appropriate treatment for an individual cancer patient [300].
5.3.4 Clinical Implications of Chromatin Changes in Cancer
The prognostic value of various histone acetylation and methylation alterations was shown in studies of prostate cancer [301–303] lung cancer [304–306], ovarian cancer [307], pancreatic cancer [307, 308], gastric cancer [309], breast cancer [307, 310], and renal cancer [311]. Interestingly, for some cancer types certain histone modifications were found to act as independent prognostic factors, providing additional nonredundant information to the clinicopathological markers currently in use. This was reported for example for acetylated H3-K18 in breast cancer [310] and renal cancer [311] and for trimethylated H3-K27 in breast, ovarian, and pancreatic cancers [307].
One of the most remarkable examples of the clinical implications of epigenetics in cancer is its role in plasticity of the cancer cell phenotype. A cancer is considered to comprise two populations: a pluripotent stem-cell-like population and a more differentiated population. Sharma et al. showed the existence of a highly drug resistant stem-cell-like population in the PC9 lung cancer cell line [312]. The transition between the stem-cell-like population and the more differentiated population seemed to be mediated by the levels of the lysine-specific demethylase 5A (KDM5A, JARID1A). Such epigenetically determined plasticity has major implications for cancer therapy as no single treatment approach is likely to target both populations of cancer cells.
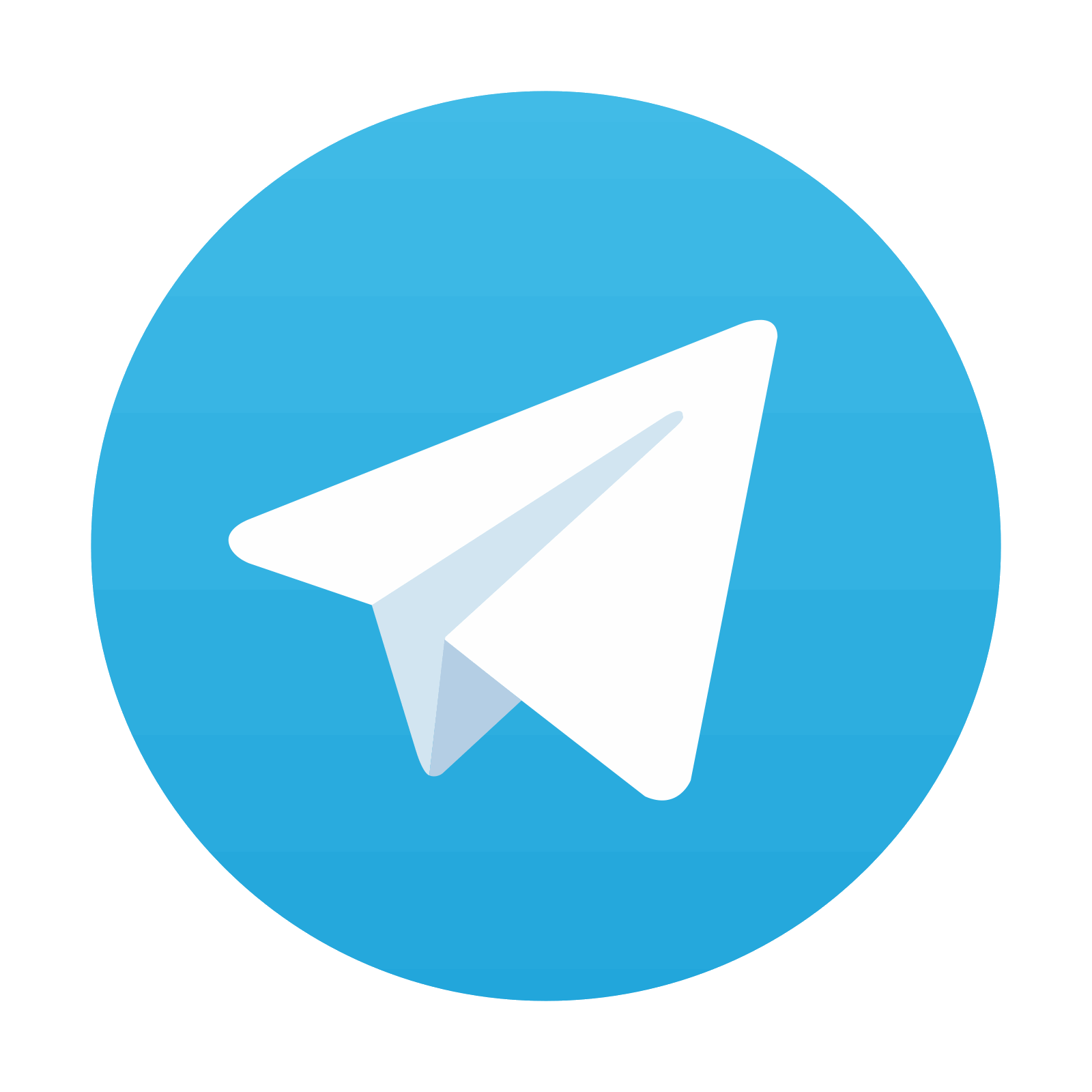
Stay updated, free articles. Join our Telegram channel
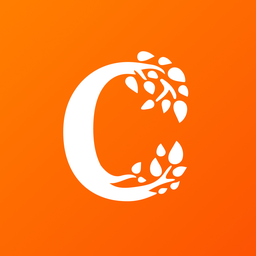
Full access? Get Clinical Tree
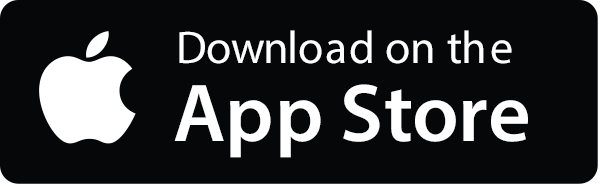
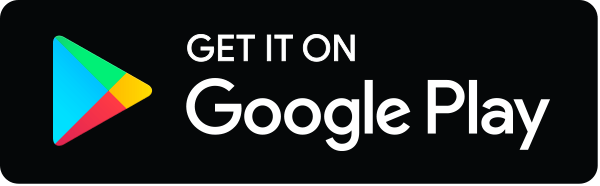