Enteric hormones orchestrate appetite, food-seeking behaviors, intake, physical and chemical digestion, and the absorption of the nutrients, intricately linking the brain, the intestines, and multiple endocrine organs to sustain the energy balance of an organism. In 1902, William Maddox Bayliss and Ernest Henry Starling demonstrated that the gastrointestinal (GI) system is the body’s largest endocrine organ. Their work with secretin establishes roles of the GI system beyond digestion and nutrition and includes systemic signaling between regions of the GI system and the central nervous system (CNS) to coordinate appetite, satiety, digestion, and absorption.
Work by John Sidney Edkins in 1902 led to discovery of “gastric secretin,” now known as gastrin. Secretin and gastrin were thought to be the primary chemical mediators of digestion until the 1920s, when cholecystokinin (CCK) was added to the list. Until the 1970s, these three mediators remained the central dogma of GI hormonal signaling. The 1970s, however, yielded an explosion in GI hormone research and the discovery of over 100 additional hormones. The GI system produces exclusively peptide hormones.
Enteric hormones are of great evolutionary importance. Many human enteric hormones can be isolated in similar forms from other species and arise from common precursor tissue-specific mechanisms, known as prohormone processing, yielding a variety of end products from a common precursor. These “alternative splicing” mechanisms create unique mediators with specific functions. Precursor hormones and their derivatives are referred to as a hormone family. These enteric hormone “families” include the gastrin, secretin, and somatostatin (SST) families, among others (Table 10-1). These examples represent conservation of precursors over centuries of species’ evolution. Despite the unique chemistry derived from the tissue-specific processing, similar signaling may occur at receptor sites within/between these families.
In addition to roles in nutrient digestion, absorption, and satiety, many of the GI hormones, including CCK, gastrin, and glucagonlike-peptide (GLP-1), provide a “trophic” signal to organs of the GI system. This trophic effect helps maintain tissue in the GI tract; however, if pathologic concentrations are present, trophism can lead to overproduction of tissue, such as hypertrophied gastric mucosa in gastrinomas.
Enteric hormone regulation involves negative feedback loops, presence of products of digestion, direct hormonal signaling, and neurotransmission. One example of a negative feedback loop involves reduction of gastrin secretion in the stomach due to low pH encountered in the small intestine. Partially digested proteins yield polypeptides, which, when sensed in the small intestine, stimulate secretion of several hormones, including the incretins. Additional mechanisms for regulation of GI hormones include a hormonal “off” signal; for example, secretion of SRIH from the pancreas and other cells halts secretion of several secondary hormones including CCK, gastrin, and secretin. The CNS plays a significant role in the regulation of the GI system, and vice versa. Neuropeptides such as vasoactive intestinal peptide (VIP), substance P, and CCK are used by the brain to regulate GI system activity, including, but not limited to, motility, secretion, and secondary signaling mechanisms of primary hormones.
The “brain-gut axis” consists of the CNS, the GI system, and enteric nervous system (composed of the myenteric and submucosal plexuses). Mediators of communication are neuropeptides that act directly via neuralinputs or circulate systemically to interact with distant targets. Recent studies of the brainstem and nearby nuclei (paraventricular nucleus, supraoptic nucleus, and arcuate nucleus) demonstrate the existence of a modified blood-brain barrier allowing GI hormones such as glucagon-like peptide-1 (GLP-1) to affect the CNS directly impacting hunger, satiety, and vagal nerve signaling.1 Additionally, the CNS affects the enteric nervous system in many ways. A common example is the anxious individual who develops abdominal pain and loose stools during emotional stress. The study of brain-gut interactions is relatively new and continues to reveal new and exciting pathways in endocrine physiology.
The process of physical and chemical digestion and nutrient absorption of carbohydrates involves energy-dependent complex interplay among several anatomical structures, including the CNS, multiple endocrine organs, the liver, and intestines as well as the microanatomy including the enteroendocrine cells, brush border enzymes, namely the disaccharidases, and transporters. Embedded in the apical membrane of enterocytes lining the duodenal villi are brush border enzymes and nutrient transporters. The enzymes are often embedded via protein anchors permitting easier access to luminal contents. The brush border enzymes are highly specific for their unique ligand, be it a disaccharide, tripeptide, or a proenzyme requiring conversion to its active form. Enzymatic activity occurs in proximity to specific transmembrane protein transporters, also embedded in the apical membrane. These transporters are also highly specific for their ligand and may use active transport, secondary active transport, or facilitated diffusion to bring nutrients into the cell. Together, enzymes and transporters digest and efficiently absorb nutrition. Each macronutrient is described in greater depth in the following text, reviewing its unique brush border enzymes and absorption systems.
Digestion occurs in multiple steps to slowly and methodically breaking down food both chemically and physically. The highly regulated process ensures adequate absorption of nutrition. Breakdown in any one of several areas causes pathology and subsequent malnutrition. Digestion can be viewed in “phases” that have distinct physiological characteristics. These stages, as elucidated here, include the cephalic phase (anticipatory priming of the GI system), gastric phase (acid secretion), and completion with the intestinal phase (inhibition of acid secretion with increased intestinal, pancreatic, and biliary secretion).
The cephalic phase of digestion is the “priming” phase in anticipation of the delivery of nutrition to the GI tract, probably evolved as a consequence of human’s eating patterns that are less like the grazing seen in other species and occur in more discrete meals.1 It is the discrete nature of these meals that mandates the GI system to be “primed” to digest and absorb efficiently. The cephalic phase is orchestrated by the vagus nerve (hence “cephalic”) and its mediating neurotransmitter, acetylcholine (Ach). Additional mediators include secretin and ghrelin. By contrast, leptin and amylin inhibit appetite. Stimulation of appetite occurs with the thought, sight, and/or smell of food, and it is sufficient to induce neural firing and priming of the GI system. In 1902, Pavlov famously proved the effect of conditioning responses in dogs; however, he was less appreciated for actually having proven the cephalic phase of digestion by proving dogs salivate in anticipation of food. Vagal stimulation mediates the breakdown and absorption of nutrition. Beginning in the mouth, Ach stimulation increases salivation and enzyme production while the stomach produces increasing levels of acid and gastrin. The endocrine pancreas begins to elevate basal insulin levels while its exocrine portion begins production and secretion of its digestive enzymes as well as bicarbonate. The liver and gallbladder increase bile production and delivery to the small intestine lumen under the influence of Ach. Intestinal motility also begins to increase. Many of the subsequent hormones listed in this chapter also are increased in response to intestinal stimulation, adipocyte stimulation, and gastric stimulation by the vagus nerve. The cephalic phase increases the efficiency of the digestive process.2
The gastric phase of digestion is initiated when the masticated, partially digested bolus of food enters the stomach. The goal of the gastric phase of digestion is to increase the stomach’s acidity to advance chemical digestion while physical digestion dramatically increases. Partially digested nutrients and distension of the stomach stimulate gastric G cells by way of direct stimulation as well as by vagovagal reflex arcs. The majority of hydrochloric acid secretion and gastrin secretion occurs during this phase.
Entrance of the chyme (partially digested food) into the small intestine is a highly regulated process and marks the beginning of the intestinal phase of digestion. The bulk of chemical digestion begins in the duodenum with enzymes that are exquisitely sensitive to pH, osmolality, and other parameters. With entry of the chyme into the duodenum, mucosal production of secretin, gastric inhibitory polypeptide (GIP), and CCK help slow motility to allow time for digestion and absorption while also inhibiting the stomach’s production of acid. Pancreatic secretion is also stimulated for release of additional digestive enzymes.
The enteric hormones, listed in Table 10-2, have multiple sites of action, both within the GI tract and the CNS, linking hunger and satiety with the biochemical and hormonal processes of digestion (Figure 10-1). Within the GI system, these hormones are secreted by specialized neuroendocrine cells (Figure 10-2).
Hormone | Cell Type | Gene Locus | Receptor |
---|---|---|---|
Secretin | S cell of duodenum and jejunum | SCT gene, 11p15.5 | Gs protein receptor |
SRIH | Pancreatic δ cells, stomach D cells, and intestines | 3q28 | Five subtypes of tissue-specific Gi receptors |
Ghrelin | P/D1-cell stomach, pancreatic ε cells | 3p25.3 | GHS-R, a Gq protein receptor |
CCK | I cell | 3p22.1 | Gs and Gq protein receptor |
Gastrin | G and I cell in stomach and duodenum | GAS gene, 17q21 | CCK2 receptor, a Gq protein |
PP | PP cells | 17q21.31 | Neuropeptide Y type 4 receptor |
VIP | VIP cell | 6q24 | Gs protein receptor |
GLP-1 | L-cell ileum and colon | Glucagon gene, 2q36-37 | Gs protein receptor |
GIP | K-cell duodenum and jejunum | 17q21.3 | Gs protein receptor |
Amylin | β-cell pancreas | 12p12.1 | Calcitonin-type receptor bound to RAMP1, 2, or 3 |
Made by the duodenal S cells as well as the hypothalamus, secretin is the product of the SCT gene located at 11p15.5 and is initially made in the form of a 120-amino acid prohormone, which is then cleaved into a mature peptide of 27 amino acids.3 Secretin has significant homology with glucagon, VIP, and GIP, and has multiple actions throughout the body. Secretin binds to the aminoterminal end of a class B G-protein–coupled receptor, stimulating Gs coupling and the generation of cyclic adenosine monophosphate (cAMP). The secretin receptor, as part of the class B family, shares homology with the receptors for VIP, glucagon, GLP, GIP, growth hormone (GH)–releasing hormone (GHRH), and pituitary adenylate cyclase–activating peptide (PACAP), among others.4 Secretin release is stimulated by an acidic pH (ie, 2-4.5) in the duodenum and protein interaction with intestinal mucosa. Secretin release is inhibited by H2 antagonists.
Within the gut, secretin inhibits gastric acid secretion while stimulating bicarbonate production by the pancreatic centroacinar cells and intercalated ducts to regulate duodenal pH. Secretin enhances CCK and also stimulates insulin release in response to a glucose load. In response to secretin, pepsin is released from chief cells, and the secretion of glucagon, SRIH, and pancreatic polypeptide (PP) increases. The effects of secretin on gastrin are twofold. In normal situations, secretin decreases gastrin release; however, in a patient who has a gastrinoma, secretin paradoxically increases gastrin release.5
The role of secretin beyond the gut is one of osmoregulation through actions at the hypothalamus, pituitary, and kidney. Hyperosmolality stimulates secretin release from the posterior pituitary as well as from the hypothalamus, enhancing vasopressin release. Both secretin and its receptor are found in the paraventricular and supraoptic nuclei of the hypothalamus, where secretin induces c-fos gene expression to increase hypothalamic release of vasopressin in response to changes in osmolality. Additionally, secretin is able to regulate renal water reabsorption independent of vasopressin.6
Secretin deficiency has been purported to predispose patients to gastric ulceration during Helicobacter infection. Deficiency is thought to make an individual vulnerable to gastric acid erosion of mucosa during the infection.7
Somatostatin (SST), also known as somatotropin-releasing inhibitory hormone (SRIH), is a peptide hormone that exists in two bioactive forms: one consisting of 14 amino acids and another of 28 amino acids. The 14-amino acid form is primarily found in the brain and binds SRIH receptors 1-5. SRIH 28, in contrast, is a gut hormone, found particularly in the colon, and has tight affinity for SRIH receptor type 5 and less for receptor types 1, 2, and 3.8 SRIH receptor type 1 is found in the cerebral cortex and amygdala, as well as the GI tract. SRIH receptor type 2 occurs in cerebral cortex and in the pituitary and adrenal glands. SRIH receptor type 3 is located primarily in the cerebellum and pituitary gland. SRIH receptor type 4 is found in the brain, heart, and the islets of Langerhans. SRIH receptor type 5 is found primarily in the hypothalamus and pituitary gland.9 SRIH isoforms are produced from the SRIH gene as the 116-amino acid preprosomatostatin, which is then cleaved to the 92-amino acid prosomatostatin, which is then cleaved to SRIH-14 and SRIH-28. Specific secretion sites are the stomach, intestines, and pancreatic δ cells, as well as the periventricular nucleus of the hypothalamus. SRIH acts via an α-adrenergic G-protein–coupled (Gαi) receptor to inhibit adenylyl cyclase and activate potassium and calcium channels.
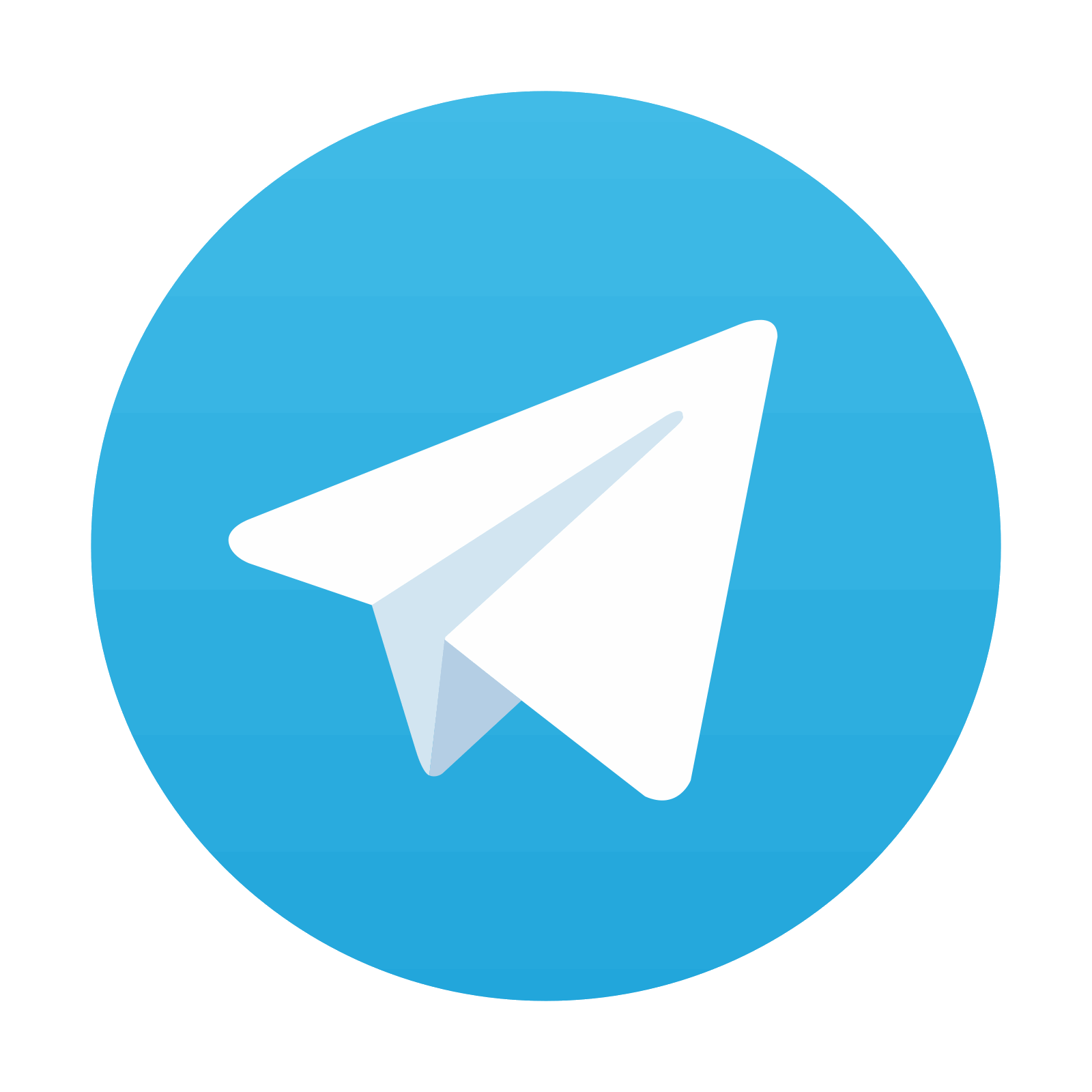
Stay updated, free articles. Join our Telegram channel
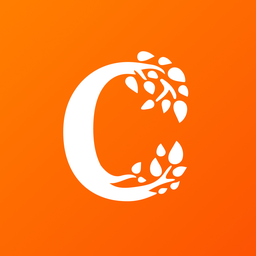
Full access? Get Clinical Tree
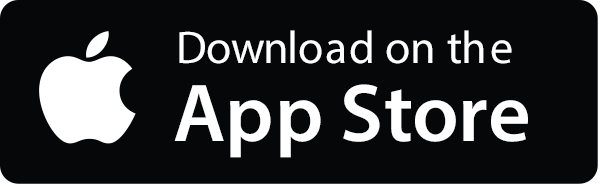
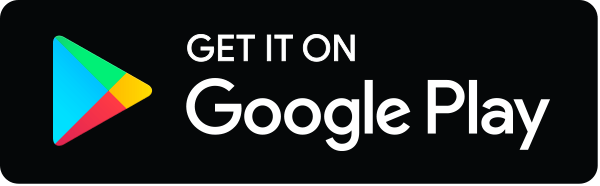