35.1
Skeletal muscle anatomy and physiology: an overview
Chapters providing details about bone biology can be found in Section 35.3 . Here we will briefly review skeletal muscle anatomy and physiology. Skeletal muscles (also known as striated muscles because of their striped appearance) are attached to bones by tendons and help control skeletal movement. Each muscle is comprised of individual multinucleated cells known as muscle fibers. Muscle fibers run the entire length of the muscle and are surrounded by a plasma membrane that contains satellite cells, myofibrils, and cytoplasm. This cytoplasm, referred to as sarcoplasm, includes an extensive network of transverse and longitudinal tubules, and significant amounts of glycosomes and myoglobin. Each muscle fiber contains several hundred to several thousand myofibrils and is made up of contractile elements known as sarcomeres. Stacked end to end, sarcomeres contain filaments of two motor proteins known as actin and myosin. Provided sufficient stimulus from the α-motor neuron, bioavailable calcium, and energy (in the form of ATP), myosin will bind to and drag actin toward the center of the sarcomere, shortening its length and generating force in what is referred to as a “power stroke.” Repeated power strokes cause the filaments to slide past one another (known as the sliding filament theory) to create a muscle contraction. This entire sequence, beginning with a motor nerve impulse and resulting in muscle contraction, is known as excitation–contraction coupling. The skeleton, in turn, provides the levers on which skeletal muscles exert force, and working together (along with connective, vascular, and nervous tissue) produce locomotion.
The close coupling between muscle and bone systems has primarily been discussed in the context of the functional muscle–bone unit proposed within the mechanostat theory . Coined by Frost over 30 years ago , the mechanostat theory posits that mechanical loading is a primary driver of bone biology. In this negative feedback system, bone continually adapts its morphology and strength based on external loads arising from gravitational loading and internal loads generated by muscular activity. This mechanical signal is then detected by cells within the bone (presumably fluid shear stress sensed within osteocytes), which in turn generates secondary biologic signals governing the modeling/remodeling response. A recent paper reviews the cellular and molecular mechanisms underlying mechanosensation in the context of muscle–bone interactions; more information is included in Part IV of this book. Isolating the effects of muscle contraction forces versus gravitational loading on bone, however, is difficult. Even in spaceflight and microgravity conditions, the muscular requirement for antigravity work decreases. While it is largely presumed that muscle places the largest load on the skeleton, differing views exist .
35.2
Muscle–bone interactions
35.2.1
Mechanical interaction between muscle and bone
Prenatally, muscle and bone tissues originate from a common mesenchymal progenitor, with myogenesis and skeletogenesis occurring in close physical and temporal proximity. Although tightly orchestrated by various pleiotropic factors , several lines of evidence suggest that muscle forces play a key role in regulating bone shape for optimal load-bearing capacity during embryogenesis. For instance, the absence of functional skeletal muscles in muscle-less or paralyzed mice alters bone and joint development . In contrast, external limb manipulation in mouse embryos with abnormal muscle development can partially compensate for the lack of mechanical stimuli in skeletogenesis . Further, case study reports of congenital disorders resulting in decreased fetal movement or immobilization also describe a fragile bone phenotype .
Evidence also supports a continued dominant role of muscle over bone in coordinating postnatal growth and homeostasis . During puberty, muscle mass accretion precedes gains in bone mass , with both tissues increasing proportionally until approximately the third decade of life . Muscle mass is a predictor of peak bone mineral density (BMD) , and as in young adulthood, muscle mass appears to be lost before bone mass, with muscle parameters correlating tightly with BMD loss . Effects of exercise and disuse also suggest that changes in muscle drive changes in bone. For example, exercise physiology studies show major differences in bone size and density in dominant versus nondominant arms in lifetime tennis players . In addition, bones in paralyzed lower extremities lose or lack over 40% of their normal strength and mass ; similarly, spinal cord injury resulting in immobilization is associated with rapid and profound muscle loss prior to bone loss .
35.2.2
Biochemical interaction between muscle and bone
Although mechanical forces are important in regulating bone and muscle homeostasis, the sensitivity of the mechanostat is regulated by nonmechanical stimuli . For example, estrogen alters the set point at which bone responds to mechanical load (described below and in Chapter 79 : Long-term bisphosphonate treatment: continuation and interruption). Additional evidence in support of a nonmechanical role of muscle on bone health comes from the clinical observation that muscle flaps can be the source of local trophic factors and/or stem cells for bone, independent of biomechanical forces . Even muscle contraction via exercise, which induces a highly site-specific mechanical stimulus, affects distant sites in the skeleton , suggesting that muscle affects bone formation via nonmechanical and systemic mechanisms. Finally, the mechanostat theory alone cannot fully explain the rapidity of bone loss in advanced age, or the failure of weight regain to restore weight loss–associated bone loss —two clinical scenarios that we will discuss in subsequent sections. Given their proximity, it certainly is plausible that muscle and bone are interacting via paracrine signaling, and likely that factors secreted by contracting muscle can synergize with mechanical loading to regulate bone mass. Below we discuss major biochemical signaling factors secreted by muscle (myokines) and bone (osteokines) that provide cross-talk to bone and muscle, respectively ( Fig. 35.1 ).

35.2.3
Myokines influencing bone
Muscle is an active source of “myokines,” or peptides that are produced and released by muscle cells (typically during contraction), several of which influence bone growth and development. Over 600 proteins that potentially interact with bone have been identified, including 35 growth factors, 40 cytokines, and 36 metallopeptidases, several of which are discussed in the following subsections.
35.2.3.1
Transforming growth factor-β superfamily
The transforming growth factor (TGF)-β superfamily includes over 40 proteins, generally divided into three subfamilies: TGF-βs, bone morphogenetic proteins (BMPs), and activins/inhibins. The most studied TGF-β superfamily molecule with regard to bone is myostatin, a powerful negative regulator of muscle mass and function . Myostatin is mainly expressed in skeletal muscle. The active form of myostatin binds to the activin receptor IIB, which downregulates the expression of differentiation-inducing genes, such as myogenin, myogenic regulatory factor 5 (Myf5), and myoD, and inhibits protein kinase B (Akt) phosphorylation to ultimately prevent myoblast differentiation and proliferation.
Inhibition of Akt phosphorylation results in the dephosphorylation of Forkhead box-O1 (FOX-O1) and FOX-O3, leading to the upregulation of the ubiquitin–proteasome proteolytic pathway. The loss of myostatin (observed in knockout models or following spontaneous mutations) produces hyperplasia and gross hypertrophy in the musculature, along with enhanced muscular function via increased fiber cross-sectional area (CSA) and fiber number. Studies in animals and humans show that exercise training can reduce myostatin expression within muscles and blood .
Although most studies have focused on myostatin’s role in skeletal muscle regulation, more data are emerging regarding the effects of myostatin signaling on bone and cartilage . Though myostatin can work indirectly on bone by increasing the load forces coming from muscles, there is evidence that it also may play a direct role. Myostatin can bind to the activin receptor located on osteoblasts and chondroblasts to decrease osteogenic differentiation of bone marrow–derived mesenchymal stem cells . In mice devoid of myostatin, bone mass accretion and bone turnover are increased in the limbs and spine . Myostatin also appears to be a direct regulator of osteoclast differentiation. Myostatin inhibition leads to reduced osteoclast formation and less bone erosion in animal models of rheumatoid arthritis . These data suggest that myostatin is a negative regulator of bone formation, but a positive regulator of bone resorption.
Other TGF-β superfamily molecules produced by skeletal muscle may also influence osteogenesis. Although primarily produced by bone, BMPs are also expressed in skeletal muscle. BMP-2, -4, -5, -6, and -7 each has strong osteogenic capacity, which is critical for bone and cartilage development . In addition, activins, particularly activin-A, are expressed abundantly in bone and to a lesser extent in skeletal muscle. In muscle, activins consistently induce skeletal muscle catabolism in animal models . Conflicting evidence exists concerning their role in bone; however, most emerging evidence suggests that activins act as negative regulators of bone mass.
Novel drug therapies aimed at inhibiting myostatin and/or activin receptors are under investigation. In a recent trial, ACE-03, a myostatin inhibitor, improved lean body mass and BMD in patients with Duchenne muscular dystrophy . However, adverse events, including telangiectasias (spider veins) and epistaxis (nosebleeds), contributed to early discontinuation of the study. Because myostatin inhibitors are injected into the circulation, their effects are not tissue-specific; thus there is concern they may weaken blood vessels and cause internal bleeding. Myostatin inhibition as a therapeutic option remains promising, but more work is needed to address safety concerns.
35.2.3.2
Follistatin
Activities of TGF-β superfamily molecules can be regulated by follistatin, a glycoprotein with a high affinity to the activin IIB receptor. Although mainly produced by the liver, skeletal muscle also expresses follistatin, especially after exercise. Follistatin is an antagonist of myostatin and activin-A activity that enhances muscle and bone growth and may be useful in improving musculoskeletal health, although more research is needed.
35.2.3.3
Irisin
Irisin is an exercise-induced myokine activated via the peroxisome proliferator-activated receptor-γ coactivator-1-α (PGC1-α) pathway. Irisin is important to metabolic regulation, including protection against insulin resistance and cardiovascular disease. In muscle, irisin increases myogenic differentiation and myoblast fusion by activating satellite cells, enhancing protein synthesis, and reducing protein degradation to induce hypertrophy and rescue denervation-induced atrophy . In bone, some studies show irisin increases osteoblast differentiation and suppresses osteoclast formation ; however, a recent study by Kim et al. identified a potentially unfavorable effect of chronically high irisin levels on osteocytes, which suggests that irisin may exert both anabolic and catabolic effects on bone depending upon tissue exposure time and irisin concentration. Though better assays are needed to accurately quantify irisin, it shows promise as a biomarker for muscle and bone disorders, as well as a potential therapeutic target. For example, in a murine model of hind-limb suspension-induced muscle wasting, irisin treatment prevented bone loss when administered during suspension and induced recovery of bone mass when administered after bone loss .
35.2.3.4
Interleukins
Numerous interleukins (ILs) are produced by muscle tissue and exert effects on both muscle and bone. Contracting muscles secrete IL-6, which increases systemic IL-6 concentrations. IL-6 enhances early-stage osteoblast differentiation, plays a dominant role in osteoclast formation, and is implicated in the pathogenesis of postmenopausal osteoporosis . Further, deficiency in ciliary neurotrophic factor, part of the IL-6 family, is associated with larger bone mass and activated osteoblast activity in animals .
In addition to IL-6, IL-5, IL-7, IL-8, and IL-15 also may influence bone turnover. IL-5 overexpression mediates bone formation by mobilizing marrow-derived osteogenic progenitors and inhibiting recruited osteoclasts , while overexpression of IL-7 and IL-8 induces osteoclastic bone resorption . Some evidence suggests that IL-7 and IL-8 also may inhibit bone formation . The specific role of IL-15 on bone health is multifaceted. Its overexpression causes increased muscle and bone mass by directly and indirectly stimulating differentiation and activation processes of osteoclasts , and by triggering osteoclast apoptosis; the net result is decreased bone erosion .
35.2.3.5
Matrix metalloproteinases
Matrix metalloproteinases (MMPs) are degrading enzymes with a pivotal role in extracellular matrix remodeling. In skeletal muscle, MMPs are important in the homeostasis and maintenance of myofiber functional integrity via breaking down extracellular matrix, thereby facilitating satellite cell migration and differentiation . How muscle-derived MMP affects bone metabolism remains unknown; however, in the bone, MMPs regulate osteoblast/osteocyte differentiation, bone formation, and osteoclast recruitment and migration . Mutations in genes encoding MMP-2 are associated with severe muscle and bone abnormalities in humans, including multicentric nodulosis, arthropathy with joint erosion, and osteolysis . Significant effort has been expended in attempts to design effective inhibitors of MMP activity, with the goal of curbing the joint destruction seen in arthritis.
35.2.3.6
Newly discovered myokines
The myokines discussed previously that influence bone are not all inclusive. Indeed, several recent reviews of cross-talk between muscle and bone tissue identified other myokines that may have a biochemical influence of muscle on bone . Some myokines identified as potential therapeutic biomarkers of bone formation include fibroblastic growth factors, brain-derived neurotrophic factor, musclin, osteonectin, monocyte chemoattractant protein-1, and leucine-rich proteoglycans such as decorin and osteoglycan. However, little is currently known about their influence on bone.
35.2.4
Osteokines influencing muscle
Although less is known about the paracrine effect of bone on muscle, bones are increasingly recognized as biochemical communicators . A clinically relevant example of bone–muscle cross-talk is osteogenesis imperfecta ( Chapter 55 : Relationship between periodontal disease, tooth loss, and osteoporosis), a congenital bone disorder leading to collagen-deficient and brittle bones, which coincides with muscle weakness. Part II of this book has a detailed discussion of “osteokines”; here, we touch on the growing list of bone cell-secreted factors possibly involved in muscle growth and development.
35.2.4.1
Prostaglandins
Prostaglandins are metabolites of polyunsaturated fatty acids, such as arachidonic acid. Prostaglandin E2 is the most widely produced prostaglandin and is produced by osteocytes in response to mechanical load. Prostaglandin E2 stimulates both bone resorption and bone formation, but favors bone formation, resulting in increased bone mass and strength . Newer in vitro evidence suggests that cross-talk exists with prostaglandin E2 that affects myogenic differentiation of myoblasts . However, side effects, including diarrhea and lethargy , make prostaglandin E2 an unacceptable musculoskeletal treatment.
35.2.4.2
Wnt signaling pathway
Osteocyte-like cells (i.e., MLO-Y4) and primary osteocytes secrete factors that activate the Wnt signaling pathway . Wnt signaling plays a role in many biological processes important to tissue homeostasis, including renewal of stem cells, stimulation of preosteoblast replication, induction of osteoblastogenesis, and inhibition of osteoblast and osteocyte apoptosis . Wnt signaling also influences the expression of Myfs to directly activate the Akt pathway and is involved in regulation of satellite cell differentiation and self-renewal to induce muscle hypertrophy and improve strength. Although still being explored in animal models, the Wnt signaling pathway is a promising therapeutic target to improve bone and muscle mass and quality because it is associated with reduced fibrosis and adipogenic infiltration .
35.2.4.3
Sclerostin
Sclerostin is produced by osteoblasts, osteocytes, and articular chondrocytes. When it binds to its receptors on the cell surface of bone and cartilage tissues, a downstream cascade of intracellular signaling results in inhibited osteoblastic bone formation and muscle cell signaling. Clinical genetic skeletal disorders associated with low sclerostin levels, such as sclerosteosis and van Buchem disease, are associated with a high BMD phenotype and lower fracture risk . In older adults with obesity, the increased sclerostin often observed with weight loss can be modulated by exercise training. Sclerostin increases are associated with hip BMD and muscle mass loss , possibly by affecting the Wnt signaling pathway , suggesting that sclerostin is a negative regulator of bone and skeletal muscle. Romosozumab, a monoclonal antibody that binds sclerostin, has recently been approved by the Food and Drug Administration (FDA) following successful studies showing lower vertebral fracture risk in postmenopausal women with osteoporosis . Other drug trials aimed at inhibiting sclerostin are underway and are a promising approach for osteoporosis treatment.
35.2.4.4
Osteocalcin
Osteocalcin is among the most abundant proteins in bone and is produced exclusively by osteoblasts. Osteocalcin binds to Gprc6a, a G protein–coupled receptor, on osteoblasts to inhibit bone mineralization and formation. Osteocalcin may have a broader role in regulating whole-body metabolism since Gprc6a is expressed in several tissues, including the β cells of the pancreatic islets, skeletal muscle, and adipose tissue. Observational studies in human cohorts have identified an association between osteocalcin and muscle mass , as well as muscle strength . Further, exercise can increase systemic osteocalcin, in association with increased insulin sensitivity and muscle strength . These data indicate that osteocalcin produced by bone actively communicates with other organ systems and exerts a positive effect on glucose metabolism and skeletal muscle regulation.
35.2.4.5
Newly discovered osteokines
Additional osteokines that may influence bone and muscle are being identified rapidly. For example, bone marrow mesenchymal stromal cells appear to stimulate myoblast proliferation through vascular endothelial growth factor , while deletion of connectin 43, expressed in osteoblasts, osteocytes, and osteoclasts, leads to impaired muscle formation in mice . Further, the hedgehog signaling pathway, particularly the Sonic and Indian hedgehog variants, is essential in wound healing and is involved in regulation of cartilage development, bone formation, and synovial joint formation. Newer evidence also suggests that it has therapeutic implications for muscle development and repair .
35.2.5
Systemic factors influencing both muscle and bone
Muscle and bone also respond to systemic influences, and many overlapping systemic signaling pathways present in muscle and bone development exert similar functions in both tissues. We will focus on major endocrine pathways affecting both muscle and bone, including sex hormones, growth hormone (GH), and insulin-like growth factor-1 (IGF-1) (see Table 35.1 ), and will touch on novel markers warranting further investigation.
Factor | Effect |
---|---|
Estrogen | + |
Testosterone | + |
Growth hormone | + |
Insulin-like growth factor-1 | + |
Vitamin D | + |
Thyroid hormone | + |
Glucocorticoids | +/− |
Adipokines | +/− |
Insulin | + |
35.2.5.1
Sex hormones
Estrogen and testosterone play fundamental roles in skeletal growth and bone homeostasis in both men and women. Estrogen and testosterone levels are correlated with greater BMD and muscle strength, while deficiency of each is characterized by loss of bone and muscle tissue . Muscle and BMD loss accelerate with aging, particularly following menopause in women, by causing an imbalance between muscle protein synthesis and degradation, and bone resorption and formation. This accelerated loss of muscle and bone may be prevented by androgen replacement therapy . However, studies suggest that androgen replacement therapy might increase the risk of prostate and breast cancer, sleep apnea, and stroke .
35.2.5.2
Growth hormone/insulin-like growth factor-1
GH is released into the blood from the anterior pituitary gland in response to neurosecretory nuclei of the hypothalamus. While GH can have direct effects on a number of tissues, including skeletal muscle, bone, and the epiphyseal growth plate , the best-characterized effects occur through stimulation of IGF-1 production, mainly by the liver. Phosphorylation of IGF-1 receptors in skeletal muscle activates the Akt pathway to upregulate protein synthesis and inhibits protein degradation. Phosphorylation of IGF-1R on chondrocytes, osteoblasts, and osteocytes and in skeletal muscle can promote bone and muscle health by causing phosphorylation of Akt and subsequent activation of downstream substrates, including the Forkhead group of transcriptional factors. When activation of this pathway is localized to muscle fibers along the muscle–bone interface in animal models, it can play a role in myoblast proliferation and differentiation and cause muscle hypertrophy. It also affects the functions of differentiated osteoblasts to promote chondrogenesis and increase bone formation , supporting a cross-talk between these two tissues.
35.2.5.3
Other novel factors
The list of other endocrine factors linked to both bone and muscle health is extensive. Recent reviews have examined the impact of several of these factors, including adipokines, vitamin D, thyroid hormone, glucocorticoids (i.e., cortisol), and insulin . Their future consideration as therapeutic biomarkers is warranted. Lastly, although largely outside the scope of this review, several genetic and neural factors regulate both muscle and bone growth and homeostasis and may represent unique therapeutic intervention targets .
35.3
Sarcopenia, osteopenia, and related conditions
35.3.1
Age-associated changes in muscle and bone
Both muscle and bone mass peak in the third decade of life, and then gradually decline. Muscle mass decreases by 3%–8% per decade after age 30, accelerating after the sixth decade of life. This decrease is thought to be driven by denervation and preferential loss of type II muscle fibers , as well as the involution of total body mass in advanced age. Accordingly, reduced mechanical load exacerbates age-associated declines in the rate and amount of bone deposited within each remodeling cycle , and bone loss follows suit. Both sexes experience a 0.5%–1% annual reduction in BMD after the age of 40 (with accelerated losses observed in women during the menopausal transition). As a result, by 75 years of age, less than 60% of the young–adult bone mass remains . Ferrucci et al. comprehensively reviewed the literature on the progressive loss of muscle and bone with age. Ultimately, reductions in muscle and bone predispose older individuals to falls and fracture.
Age-associated pathologic deterioration of bone tissue is diagnosed clinically as osteoporosis . To this, we introduce the term sarcopenia—derived from the Greek word sarx (meaning flesh) and penia (meaning poverty)—coined by Irwin Rosenberg in 1989 to describe age-related reduction in muscle mass . Although sarcopenia has been recognized by geriatricians for several decades, only recently was it identified as a separate disease condition and assigned an ICD-10 code (M62.84) . This section covers the etiology and evolving definitions of sarcopenia, as well as its clinical utility; we also discuss the confluence of osteopenia/osteoporosis and sarcopenia (henceforth termed “osteosarcopenia”) and the emerging concept of osteosarcopenic obesity.
35.3.2
Sarcopenia
Sarcopenia is considered a geriatric syndrome because it has multiple contributing factors including inactivity; inadequate nutrition (energy and/or protein); changes in muscle morphology, muscle protein turnover, and hormone levels and sensitivity (e.g., insulin resistance, decreased GH, loss of estrogen or androgen hormones); chronic inflammation (particularly increased IL-6); oxidative stress; and neuromuscular degeneration . Baumgartner et al. proposed the first operational definition of sarcopenia based on relative muscle mass [lean mass in the arms and legs (i.e., appendicular lean mass, or ALM) divided by height squared] less than two standard deviations below the sex-specific mean of a reference population of young adults . This is similar to the method used to define osteoporosis. Several years later, Newman et al. proposed that sarcopenia might be better operationalized by adjusting for body fat mass and height [i.e., body mass index (BMI)], since obese individuals with both higher lean and fat mass may not appear to be sarcopenic despite inadequate muscle mass for their size . While early operational definitions of sarcopenia included only muscle mass, they now incorporate the loss of muscle function (strength and/or performance). Indeed, some have proposed a separate condition of age-associated loss in muscle strength, also known as dynapenia .
In the past decade, several international groups have proposed operational definitions of sarcopenia (see Table 35.2 ) ; however, there is no consensus and substantial differences remain regarding standardization of muscle mass to body size, the reference population used, and cut points for muscle strength and performance. The European Working Group on Sarcopenia in Older People (EWGSOP) developed an algorithm for diagnosing sarcopenia based on slow gait speed and low grip strength and muscle mass . The International Working Group on Sarcopenia (IWGS) defined sarcopenia as slow gait speed and low muscle mass . The Asian Working Group for Sarcopenia (AWGS), such as EWGSOP, recommended assessing both muscle strength (grip strength) and physical performance (usual gait speed) and, if warranted, measuring muscle mass . However, the cut point values differ due to differences in ethnicity, body size, lifestyle, and cultural background in Asian versus Caucasian populations. Most recently, the EWGSOP issued revised recommendations (EWGSOP2) with an emphasis on muscle strength and low muscle quantity or quality used to confirm the diagnosis of sarcopenia . Low physical performance is used to identify those with severe sarcopenia. To determine which patients should be assessed for sarcopenia, EWGSOP2 recommends administering the SARC-F questionnaire . This five-item questionnaire assesses a patient’s perception of his or her limitations in strength, walking, rising from a chair, climbing stairs, and falls.
Organization | Definition | Low muscle mass a | Cut points for low muscle mass | Low muscle function | Cut points for low muscle function |
---|---|---|---|---|---|
EWGSOP, 2010 | Progressive and generalized loss of muscle mass and function (strength or performance) | ASM/ht 2 | <2 SD below the mean of ASM/ht 2 in young adults (the Rosetta Study ):
| Weakness | Low grip strength as defined by a cutoff yielding the highest sensitivity and specificity in identifying participants with poor mobility :
|
Slow gait speed | ≤0.8 m/s | ||||
IWGS, 2011 | Age-associated loss of skeletal muscle mass and function | ALM/ht 2 | <20th percentile of ALM/ht 2 in Health ABC :
| Slow gait speed | <1 m/s |
FNIH, 2014 | Presence of low muscle mass and weakness | ALM/BMI | ALM/BMI that identified older persons who were weak :
| Weakness | Grip strength that identified older persons who were more likely to have mobility impairment :
|
AWGS, 2014 | Age-related decline of skeletal muscle mass plus low muscle strength and/or physical performance | ASM/ht 2 | <2 SD below the ASM/ht 2 of young reference population:
| Weakness | <20th percentile of the mean grip strength of the study population:
|
Slow gait speed | ≤0.8 m/sec | ||||
EWGSOP2, 2018 | Low muscle strength accompanied by low muscle quantity and/or quality. If physical performance is also poor, sarcopenia is considered severe | ASM or ASM/ht 2 | ASM :
| Low strength | Grip strength <27 kg in men and <16 kg in women |
ASM/ht 2 :
| Repeated chair stand time >15 s | ||||
Low performance | Gait speed ≤0.8 m/s | ||||
SPPB score ≤8 points | |||||
TUG≥20 s | |||||
Unable to complete 400 m walk test or completes 400 m walk test in ≥6 min |
The consensus approaches described previously based cut points for operational definitions of sarcopenia solely on statistical distributions within single populations. They were not validated for their ability to predict clinical outcomes relevant to age-related loss of muscle mass and function . In contrast, the Foundation for the National Institutes of Health (FNIH) Sarcopenia Project used a data-driven approach to identify criteria for clinically relevant weakness and low lean mass based on their ability to predict future mobility impairment . Eight observational cohort studies and six clinical trials with longitudinal measures of muscle mass and function were included, representing a wide range of community-dwelling older adults. Based on this approach, evidence-based cut points for weakness (low grip strength) and low muscle mass were determined (see Table 35.2 ).
The prevalence of sarcopenia varies widely depending on the operational definition used, as well as the age and population under study. In a systematic review of 18 studies that used the EWGSOP operational definition for sarcopenia the prevalence of sarcopenia ranged from 1% to 29% in community-dwelling populations (15 studies), 14% to 33% in long-term care populations (2 studies), and 10% in a hospital setting (1 study) . In a later systematic review and meta-analysis of 35 studies that used the EWGSOP, IWGS, or AWGS operational definitions the overall prevalence of sarcopenia was 10% in both men and women . The FNIH Sarcopenia Project described the prevalence, agreement, and discrepancies among the FNIH, EWGSOP, and IWGS sarcopenia operational definitions . The prevalence of sarcopenia ranged from 1.3% to 5.3% in men and from 2.3% to 13.3% in women; but the positive percent agreement (i.e., agreement on the presence of sarcopenia) between the definitions was low, ranging from 7% to 32% in men and 5% to 19% in women, while the negative percent agreement (i.e., agreement on the absence of sarcopenia) was high (>95%).
Others have also compared the various operational definitions to assess the prevalence of sarcopenia and its association with falls and fractures, and observed large differences . In a 2018 systematic review and meta-analysis of nine studies, sarcopenic participants overall had a 30% increased risk of fractures versus nonsarcopenic participants; however, in subgroup analysis, the association was only significant when using the AWGS sarcopenia operational definition . Sarcopenia has also been associated with other adverse health outcomes. In a systematic review of 17 studies using the EWGSOP criteria, sarcopenia was associated with functional decline (6 studies), falls (2 studies), higher incidence of hospitalization (1 study), and mortality (11 of 12 studies) . Not surprisingly, healthcare costs are higher in sarcopenic versus nonsarcopenic patients .
Although recognized as a major contributor to age-related fracture risk , sarcopenia is not yet incorporated into fracture risk prediction algorithms. (See Chapter 73 , Estrogen and estrogen analogs for prevention and treatment of osteoporosis, for a description of the World Health Organization’s Fracture Risk Assessment Tool, or FRAX.) Predictive accuracy of FRAX is improved by adding prior fall history , and recent data suggest discriminatory capacity can be further improved by considering muscle mass and function. For example, in two Chinese longitudinal studies in older community-dwelling adults, AWGS-defined sarcopenia predicts incident fracture, independent of BMD, and improves risk prediction models . Even adding a simple questionnaire of sarcopenic risk (the SARC-F) independently predicts hip fracture and improves FRAX sensitivity (from 58.7% to 76.2% in men and 69.6% to 78.3% in women) . More recent data from the Osteoporotic Fractures in Men study (MrOS) cohort studies (the United States, Hong Kong, and Sweden) demonstrate that parameters of muscle function (i.e., chair stand time, gait speed, grip strength) and muscle mass (i.e., ALM/ht 2 ) predict incident fracture independent of FRAX probability, BMI, and prior falls; however, muscle function—but not muscle mass—predicted fracture risk independent of femoral neck BMD .
35.3.3
Osteosarcopenia
The term “sarco-osteopenia” was coined in 2009 to emphasize that low lean mass and bone mass are often synchronic and compound the risk for fall-related fractures, especially hip fracture, in older adults . Several large ( n >300), cross-sectional studies suggest that sarcopenia coexists with osteopenia and/or osteoporosis ; this association appears to be true for both sexes, across several countries and using various operational definitions of the conditions. For instance, middle-aged and older community-dwelling men with sarcopenia (defined as in Ref. ) in the European Male Ageing Study were three times more likely to have osteoporosis than those with normal skeletal muscle mass . Sarcopenia has also been associated with osteopenia and osteoporosis in Japanese women and Korean men and women . Of note, and as one might expect, the association between sarcopenia and osteoporosis persists postfracture, where the prevalence of both conditions is markedly higher than the general population . Data from a Finnish cohort of women confirm and extend studies demonstrating an association between sarcopenia [using the relative skeletal muscle index (SMI), grip strength, and gait speed] and osteoporosis by implicating grip strength as the clinical sarcopenia criterion linking both conditions . For example, in postmenopausal participants in the Osteoporosis Fracture Prevention study, those in the lowest quartile of handgrip strength were nearly 12 times more likely to have osteoporosis than those in the highest quartile. Notably, this study also showed that sarcopenic women had a two- to threefold increased odds of falls and fractures during the previous year compared to nonsarcopenic women .
Referred to now as osteosarcopenia, this “hazardous duet” is associated with several negative health outcomes, including falls and fractures (detailed below), depression , frailty , and mortality . To date, five studies have examined the risk of incident fracture among those with sarcopenia, low bone mass, or both . Reported prevalence of osteosarcopenia ranged from 2% to 13%, with women consistently more affected than men. In all studies, osteosarcopenia was associated with a two- to threefold increase in the risk of fracture compared to those without either condition, although in only two (both in men) did the risk exceed that of the osteopenic/osteoporotic group . In the first study, community-dwelling men aged 65 years and older were followed for 11 years; fracture risk was 3.5-fold higher when both sarcopenia and osteoporosis were present and significantly higher than either condition alone . Sarcopenia remained an independent predictor of fracture, even after adjustment for BMD. Importantly, among individual components, low grip strength and slow gait speed, but not low appendicular SMI, were significantly associated with higher fracture risk. Similarly, among older men in The MrOS, the risk of nonspine fracture was substantially greater for men with low BMD and sarcopenia (3.8-fold) than for men with only one condition (1.1–1.7-fold) or healthy controls . A borderline significant interaction between the two conditions indicated that the effects of sarcopenia and low BMD on fracture risk may depend on each other.
In older women in the Study of Osteoporotic Fractures the major risk of fracture was due to low BMD . However, three of the four studies showed that sarcopenia alone was not an independent risk factor for fracture in men or women . In the only study to look at osteosarcopenia and incident falls, fall rates among those with osteosarcopenia and those with only sarcopenia were similar (40%–60% increase compared to control) . Clearly, more work is needed; at present, the data suggest that the combination of low bone mass and sarcopenia increases fracture risk (as compared to neither condition) due to reduced BMD. However, limited evidence in men suggests that sarcopenia, and in particular low muscle function, incrementally increases fracture risk. This sex-specific finding is intriguing and warrants further investigation.
35.3.4
Sarcopenic obesity and related conditions
Unlike the involution of muscle and bone mass that begins in middle age, body fat mass increases until roughly the seventh decade of life . Excessive fat accumulation is commonplace among older adults; recent survey data classify over one-third as obese , with a projected increase to 50% by 2030 . Fat distribution is also affected by age, with older adults more likely to store fat in ectopic regions, such as skeletal muscle . In addition to well-known cardiometabolic complications, both increasing overall and intermuscular fat deposition are associated with accelerated lean mass loss and mobility limitations . Potential biologic mechanisms linking excess fat mass to disability risk include increased expression of proinflammatory cytokines, which may inhibit muscle protein synthesis, and downregulation of insulin, testosterone, and GH .
The cooccurrence of obesity and sarcopenia is known as sarcopenic obesity, and a significant subset of older adults present with this high-risk geriatric syndrome. Excellent reviews by Cauley and Batsis and Villareal explore the etiology and epidemiology of sarcopenic obesity. In large community-based cohorts the prevalence of sarcopenic obesity ranges from 0% to 41% among older adults, depending on the definition used . In most cases, rates increase with age and are higher in women than men . For example, among nearly 5000 older adults (ages 60+) participating in the NHANES observational study from 1999 to 2004, nearly 13% and 34% of men and women, respectively, were categorized as having FNIH-defined sarcopenic obesity, with numbers increasing roughly 10% per decade from 60 to 80 years of age .
Importantly, sarcopenic obesity is often associated with worse clinical outcomes than either condition in isolation. In addition to increased disability risk , sarcopenic obesity is also positively associated with increased risk of falls and fracture . A recent study among nearly 1500 community-dwelling older men reported that EWGSOP-defined sarcopenic obesity is associated with a 66% increased risk of falls over two years. This same study also found that these participants had a significantly increased risk of incident fractures compared with nonsarcopenic obese men . Likewise, in another large study of community-dwelling older adults, sarcopenic obesity (defined as the lowest sex-specific tertile for ALM or lower-limb strength, respectively, and the highest sex-specific tertile for total fat mass) was associated with reduced BMD and an approximate 3.5-fold increased risk of incident fracture in men, compared to nonsarcopenic, nonobese men, or each condition independently . The long-held view that obesity is osteoprotective has been recently challenged , with data showing that many—if not most—osteoporotic fractures occur in older adults who are overweight or obese . A detailed discussion of bone-fat interactions is included in Chapter 41 , Osteoporosis in neurological disorders: Parkinson’s disease, stroke, and multiple sclerosis—July 28, 2019.
A direct extension of work done to define and quantify health risk associated with osteosarcopenia and sarcopenic obesity is the emerging concept of osteosarcopenic obesity, the ultimate impairment of bone, muscle, and fat due to aging . To date, few studies have specifically studied individuals with all three conditions; thus the presumed additive health risk associated with osteosarcopenic obesity is largely based on what is known about each disorder individually . Limited cross-sectional data in community-dwelling older women suggest that the prevalence of osteosarcopenic obesity ranges from 12% to 19%. Associations are beginning to emerge between osteosarcopenic obesity and reduced physical function and poor diet quality . A related condition known as dysmobility syndrome has recently been coined , which integrates fall history risk into osteosarcopenic obesity. As expected, dysmobility syndrome is associated with poorer clinical outcomes, including reduced function , and increased risk of fracture and mortality . Although it remains to be seen which operational construct will be adopted clinically, consideration of osteopenia, sarcopenia, and obesity as inter-related conditions—perhaps even the same malady at a different stage in disease progression—and as they relate to fragility fracture, has major implications for informing prevention and treatment strategies.
35.4
Sarcopenia assessment tools
Nonimaging and imaging techniques have been used to varying degrees to assess osteosarcopenia in research and clinical care. As discussed earlier, bone and muscle deficiencies can present in combination and produce poor patient outcomes; however, different tools are used to diagnose these conditions. Osteoporosis evaluation is covered in Part VIII: Diagnosis and evaluation; here, we focus on muscle and sarcopenia assessment (see Table 35.3 for an overview of each tool). Nonimaging assessments include measures of muscle function, muscle mass, and biochemical markers. Imaging assessments include dual-energy X-ray absorptiometry (DXA) estimates of muscle mass, and ultrasound, computed tomography (CT), and magnetic resonance imaging (MRI) measures of muscle size and quality (e.g., fat infiltration). These measures have varying degrees of acceptance for use in tracking muscle decline and sarcopenia, with muscle function, CT, and MRI being most highly recommended . While DXA is most commonly used for sarcopenia assessment in clinical care, CT and MRI are considered gold standards since they provide direct measures of muscle mass and quality and are less affected by obesity and weight loss.
Assessment | Description | Pros and cons | |
---|---|---|---|
Nonimaging | |||
Muscle function |
|
| |
Muscle mass |
|
| |
Biochemical markers |
|
| |
Imaging | |||
Ultrasound |
|
| |
DXA |
|
| |
CT |
|
| |
MRI |
|
|
a Highly recommended/gold standard for muscle/sarcopenia assessment.
35.4.1
Nonimaging assessment techniques
Nonimaging assessments for sarcopenia have been used widely in clinical care and research, and include measures of muscle function, muscle mass, and biochemical markers.
35.4.1.1
Muscle function
Physical function questionnaires have been used to screen for sarcopenia, such as the SARC-F . SARC-F scores greater than or equal to four have proved valid in detecting sarcopenic individuals at risk for adverse outcomes in large cohorts including NHANES. Although SARC-F has moderate to high specificity, it has low sensitivity in identifying individuals with sarcopenia . Other tools include muscle strength, muscle power, and physical performance measures. Muscle strength can be assessed by handgrip strength or knee flexion/extension. Although lower extremity muscle strength is more relevant for gait and physical function, grip strength measured with a hand-held dynamometer is a good simple measure of muscle strength, particularly in clinical settings. Cut points for low grip strength have been established by various consensus groups, ranging from 26 to 30 kg for men and 16 to 20 kg for women ( Table 35.2 ). Grip strength is correlated with leg strength and is associated with mobility limitations , disability in activities of daily living , and mortality . Muscle power measured from leg press or knee extension high-velocity resistance training is highly predictive of functional status and is potentially more sensitive in detecting muscle physiology change; however, more research is needed to establish normative data and sarcopenia cut points .
Physical performance measures include gait speed, the Short Physical Performance Battery (SPPB), and the Timed Up and Go (TUG), which have established cut points for low muscle function (see Table 35.2 ). Gait speed over short distances (3–6 m) is a quick measure of performance and is increasingly being used in clinical practice . Slow gait speed is associated with disability, falls, and mortality . The SPPB is a composite test of lower extremity function including tests of balance, gait speed, and lower extremity strength and power (chair stand test); lower SPPB scores are associated with disability, nursing home admission, and mortality . The TUG assesses an individual’s ability to stand from a chair, walk a short distance, turn around, walk back to the chair, and sit down; slower times predict fall risk . Although the EWGSOP2 definition includes a 400 m walk as a physical performance outcome , this test requires a 20-m course and is best suited to a research setting.
35.4.1.2
Muscle mass
Simple anthropometric measures of muscle mass (BMI, skinfold thickness, and body circumference) are generally not sensitive enough to evaluate sarcopenia. Bioelectrical impedance analysis (BIA) is a technique that applies an electrical current through the body to estimate muscle mass based on the current’s conduction through water, since muscle has the greatest water composition of all body tissues . BIA-based fat-free mass is fairly well correlated with DXA lean mass but can be influenced by body positioning, hydration, edema, diet, temperature, and recent physical activity . BIA is a portable alternative to DXA, and several consensus statements accept BIA as a method for measuring low muscle mass and diagnosing sarcopenia ; however, more research is needed to validate prediction equations for specific populations. Electrical impedance myography (EIM) is a recently described technique that measures impedance for individual muscles and thus is less affected by body positioning and hydration . EIM has reliably detected muscle changes in amyotrophic lateral sclerosis patients , and the primary EIM parameter (phase) declines with age ; however, further research is needed to establish normative data and sarcopenia cut points using EIM.
35.4.1.3
Biochemical markers
Serum and urinary biomarkers associated with skeletal muscle decline include markers of inflammation (e.g., C-reactive protein, IL-6, tumor necrosis factor-α); nutrition (e.g., albumin, hemoglobin); hormones (e.g., dehydroepiandrosterone sulfate, testosterone, IGF-1); oxidative damage (e.g., advanced glycation end-products, protein carbonyls, oxidized low-density lipoproteins); antioxidants (e.g., carotenoids, α-tocopherol); skeletal muscle remodeling (e.g., procollagen type III N-terminal peptide); and neuromuscular junction (e.g., C-terminal agrin fragment). Despite widespread use in research, many are only weakly associated with clinically relevant outcomes , although recent work has aimed to aggregate select biomarkers—such as IL-6, secreted protein acidic and rich in cysteine, macrophage migration inhibitory factor, and IGF-1—into a single risk score for early diagnosis and prognosis of sarcopenia . Newer analytic techniques have allowed the evaluation of circulating microRNAs and metabolites as potential biomarkers of skeletal muscle physiology. Lastly, in recent studies, the deuterated creatine (D 3 -creatine) dilution method is a more direct way to assess muscle mass than DXA . In this method a stable isotope of creatine given orally in water is digested, absorbed, and transported into muscle, where it is metabolized into creatinine and excreted in urine. D 3 -creatinine enrichment in urine is related to skeletal muscle-labeled creatine enrichment and therefore whole-body muscle mass, since 95% of the body’s creatine is located in muscle. Work integrating the D 3 -creatinine method into experimental studies, where its sensitivity to change can be better understood, is in progress. While these biomarkers may correlate with muscle decline, further development of age-, sex-, and race-specific reference data and sarcopenia cut points for these markers is needed.
35.4.2
Imaging assessment techniques
A number of conditions (e.g., orthopedic disorders, neurologic conditions, or medications) can contribute to false-positive results in nonimaging assessments of sarcopenia. Therefore imaging assessment tools are increasingly used for diagnosing sarcopenia, particularly when functional screening tests are positive . Advantages of imaging include its clinical availability and the ability to measure multiple types of tissue composition (e.g., muscle, bone, and fat) in some modalities (e.g., DXA and CT). This is valuable for assessing conditions such as osteosarcopenic obesity, cachexia, or dysmobility syndrome, which involve multiple tissue impairments. Imaging assessments of bone and fracture risk are covered in Chapter 71 , Nutrients beyond calcium and vitamin D to treat osteoporosis; this section focuses on imaging assessments of sarcopenia.
35.4.2.1
Ultrasound
The European Geriatric Medicine Society’s sarcopenia working group proposed using ultrasound to assess muscle thickness, CSA, fascicle length, pennation angle, and echogenicity (muscle quality measure of myosteatosis) . Advantages of ultrasound include its low cost, widespread availability, safety (e.g., no radiation exposure), and relative ease of use, although some technical skill is required regarding the transducer pressure and orientation to collect accurate measures . Precautions are needed to ensure the muscle is in a relaxed state, and to control hydration and recent exercise, both of which can affect accuracy . While ultrasound is a valid technique for measuring muscle size in older adults, further research is needed to validate ultrasound-derived prediction equations of muscle mass .
35.4.2.2
Dual-energy X-ray absorptiometry
DXA, the most commonly used imaging assessment for sarcopenia, uses sarcopenia thresholds for whole-body DXA-derived ALM . DXA is a relatively inexpensive, noninvasive imaging modality, which is widely available in clinical care and research. It can simultaneously measure whole-body lean mass, fat mass, and bone mass, while imparting minimal radiation exposure (~1 mrem). However, DXA indirectly measures lean mass as the summation of all nonbone and nonfat tissues. Thus ALM (sum of the lean mass in the arms and legs) is used for sarcopenia classification (see Table 35.2 ) instead of whole-body lean mass, which includes organ tissues in the trunk, android, and gynoid regions. Because skeletal muscle mass is highly correlated with height , relative ALM is used by dividing ALM by height squared. However, the FNIH sarcopenia operational definition recommends the use of ALM divided by BMI to account for the influence of obesity on the association between lean mass and muscle strength . Whole-body DXA-derived ALM measurements of two obese participants (BMI 33.8 kg/m 2 ) in the Cooperative Lifestyle Intervention Program-II indicated that, based on the FNIH cut points for low muscle mass, the 64-year-old woman was sarcopenic ( Fig. 35.2A ) but the 67-year-old man was not ( Fig. 35.2B ).
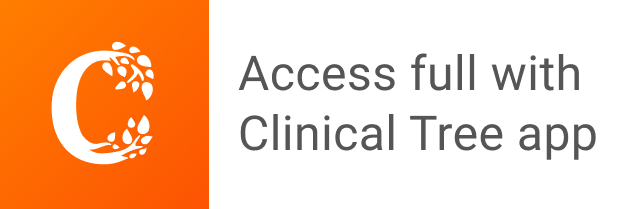