html xmlns=”http://www.w3.org/1999/xhtml”>
CHAPTER 10
Birds
10.2 DIFFERENCES BETWEEN BIRDS AND MAMMALS AND AMONG BIRD SPECIES
10.5.2 Field Exposure and Mesocosm Studies
10.5.3 Feeding Studies, Including the Proposed Avian Two-Generation Test
10.6 EXAMPLES OF EDC EFFECTS FROM FIELD STUDIES
10.7 PROPOSED TWO-GENERATION TEST
10.8.1 Avian Testing in a Tiered Risk Assessment Approach
10.1 INTRODUCTION
Since the early 1990s, public and regulatory concern about the potential effects of chemicals on the endocrine systems of wildlife [1] and humans [2] has increased. In 2000, the European Parliament adopted a resolution on endocrine disrupters that mandated application of the precautionary principle and called for the European Commission (EC) to identify substances for immediate action. In 2004, the EC presented an update on implementation of the strategy that, among other recommendations, included an adaptation/amendment of current legislation to consider potential effects of endocrine disrupters. In particular, Regulation No. 793/93 of the European Economic Community (EEC) on risk assessment and Directive 67/548/EEC on classification of dangerous substances have been promulgated. In the United States, legislation such as the Safe Drinking Water Act Amendments of 1995 and the Food Quality Protection Act of 1996 have been promulgated and require development, validation, and application of screening methods for potential endocrine-disrupting (ED) properties of chemicals. It has been difficult to develop the necessary screening tools because there are so many potential effects that could lead to endocrine disruption. In fact, any stressor, chemical or otherwise, that forces an organism out of its normal homeostatic range could be defined as an endocrine disruptor.
The U.S. federal Endocrine Disruptor Screening and Testing Advisory Committee (EDSTAC) recommended that chemicals be screened as agonists or antagonists of estrogen receptors (ERs), androgen receptors (ARs), and thyroid hormone receptors (ThR) [3]. Much of the popular press and research has focused on the effects of direct-acting chemicals that mimic hormones as agonists for hormone receptors, in particular, early concern focused on the ER. One of the first studies to investigate the effects of chemicals that act as weak estrogen agonists, such as bisphenol A (BPA) and alkylphenols, was conducted by Dodds and Lawson [4]. Although more evidence for the ED effects of these compounds was found in the 1970s [5], major health concerns were raised when BPA and nonylphenol were found to modulate the growth of Estrogen-dependent human breast cells [6,7]. In the book Our Stolen Future, Colborn et al. [8] promoted the idea that chemicals that mimicked estrogens, such as some dichlorodiphenyltrichloroethane (DDT) metabolites, were responsible for a number of adverse effects in humans and wildlife. The relevance of this finding is questionable since the primary DDT metabolite found in the environment and animal tissues is actually p,p′-dichlorodiphenyldichloroethylene (p,p′-DDE), a relatively weak ER agonist [9]. Effects in birds, such as eggshell thinning, were suggested to be due to the estrogenic effects of DDT. Also, changes in the sex ratios of birds were reported and linked to ED effects [9]. Regardless, much of the initial research, public interest, and legislation focused on hormone mimics and in particular those that were ER or AR [10,11] agonists or antagonists. Structure activity models to predict ER binding [12] as well as ER binding assays [13] were developed to screen for these estrogenic effects. More recently, several eukaryotic cell-based expression assays have been developed where an endogenous or exogenous reporter gene is expressed under control of the ER [13,14] or AR receptor [15,16]. In addition, in vitro systems based on yeast cells [17] have been developed. Together these systems have made possible identification of many environmental contaminants that might act by binding directly to hormone receptors. However, although some chemicals have been shown to modulate the endocrine system as direct receptor agonists or antagonists [18], other chemicals can cause effects by other non–receptor-mediated mechanisms [19,20].
The focus of in vitro and in vivo testing strategies in the early and mid-1990s was predominantly on chemicals that have potential to agonistically or antagonistically interact with nuclear hormone receptors; since the late 1990s, however, there has been increasing concern about alternate modes of endocrine action. Recognizing the complex nature of the issue of endocrine disruption, the U.S. Environmental Protection Agency (EPA) and the Organisation for Economic Cooperation and Development (OECD) initiated high-priority activities to revise existing, and to develop new, test guidelines for screening and testing of potential ED chemicals (EDCs). This resulted in the inclusion of additional end points, such as effects on production of the sex steroids testosterone (T) and estradiol (E2) [20] and aromatase activity, which converts testosterone to estradiol, (www.epa.gov/oscpmont/oscpendo/pubs/aromatase_isr.pdf) into current endocrine disruptor screening programs.
The utility of in vitro assay systems for identification of novel mechanisms of endocrine disruption was demonstrated by the observation that some chemicals are able to alter production of enzymes involved in steroid hormone production [19,21,22]. In particular, chemicals that alter the expression of steroidogenic enzymes have the potential to change hormone production rates as well as absolute and relative concentrations of hormones in blood and tissues of exposed organisms [21,23].
The endocrine system can be affected in many ways, and alterations in these functions can result in modulation of a range of other biological functions. Much of the concern about EDCs, however, has focused on hormone mimics and chemicals that act directly as agonists or antagonists of steroid hormones. In fact, the real question is not whether a chemical can modulate the endocrine system but what the critical concentration is to cause adverse effects on ecologically relevant end points, such a survival, growth, or reproduction. It is always useful to know the mechanism of action in order to classify chemicals for grouping in risk assessments or for developing effective biomarkers, but if chemicals have adverse effects, it is not particularly relevant whether they are through ED effects or through other mechanisms of action. The real issues are whether there can be subtle, cumulative effects of chronic exposures to small concentrations of chemicals. Effective tests can be developed to answer these questions relative to birds. Although there are many stressors that can force an animal out of homeostasis or affect corticosteroids or mineralocorticoids, here we focus on those chemicals that can affect steroid or “sex” hormones that are generally of concern to regulatory agencies. In particular, we focus on direct-acting estrogens and androgens and compounds that block or enhance the activities of these hormones or their synthesis. Even with this restriction, effects can be either direct, as hormone agonists or antagonists, or indirect, by affecting concentrations of endogenous hormones through effects on steroidogenesis [22] or degradation, or through modulation of steroid receptor expression and function. Here we focus on some specific characteristics of birds relative to effects on determination of gender and or gender-specific behavior that are regulated by hormones and can be affected by chemical stressors.
In all animals, the neuro-endocrine system is balanced and regulated to provide a means of controlling a wide range of physiological functions that maintain homeostasis. Although the avian endocrine system has many similarities to those of other higher vertebrates [24], there are life cycle characteristics, such as egg laying, that distinguish birds from model laboratory species, such as rats and mice [25]. These characteristic life cycle stages mean that birds, and indeed other oviparous vertebrates, can be uniquely sensitive to the impacts of some chemical contaminants [9]. At the same time, these characteristic life stages have provided unique opportunities to study regulated development of embryos [26] and potential impacts of exposure to chemicals on the embryo [27].
Advances in understanding of the avian endocrine system have been made, but the actual mechanism of sex determination in birds has still not been determined. Indeed, it is still uncertain whether all avian species use the same mechanism of sex determination [28]. It is anticipated that the recent sequencing of the chicken (Gallus gallus) [29] and zebra finch (Taeniopygia guttata) [30] genomes will provide better insights into some of these most fundamental functions of the avian endocrine system. These advances are also complemented by the recent development of techniques for isolation of avian stem cells [31] and germ cell progenitors [32].
Avian models offer unique opportunities to study mechanisms of adverse effects and are also needed to ensure protection of the unique life histories of birds. For example, the avian egg has long been used as a model system to study embryonic development since systems have been developed to allow continuous observation of the developing embryo until the time of hatch [33]. With respect to effects of (EDCs), avian embryos in ovo are uniquely isolated from influences of the hormone systems of the mother and siblings compared to mammalian models, where the embryo is exposed in utero to both maternal hormones and those produced by siblings [34].
In this chapter we review current methods available for assessing effects of EDCs on birds and discuss the best strategy for integrating these methods into an integrated tiered system for the assessment of potential impacts of EDCs on the environment and humans.
10.2 DIFFERENCES BETWEEN BIRDS AND MAMMALS AND AMONG BIRD SPECIES
In both birds and mammals, early development is characterized by a sexually undifferentiated state [35]. Prior to differentiation the gonads are identical in “male” and “female” embryos/fetuses, with the potential to develop into either ovaries or testes. In both animal classes, the homogametic sex is the default gender, and a differentiating hormone produced due to the presence of the odd chromosome causes the embryo/fetus to differentiate from the default sex. In mammals, the homogametic (XX) default gender is female, while the male is heterogametic (XY). The Sry region of the Y chromosome (the gender-determining region of the Y) encodes for maleness; its gene product is referred to as the H-Y antigen. The differentiating hormone for the mammalian testis is T.
In birds, male (ZZ) is the default homogametic gender, while the heterogametic gender (ZW) is female. In contrast to mammals, where the differentiating hormone is testosterone, in birds, the differentiating hormone for gonadal development is 17β estradiol (E2). Female birds have an H-W antigen, analogous to the H-Y antigen in mammals. This gene product is the differentiation antigen for the heterogametic gonad (the avian ovary). This gene is not W-linked but is present in both males and females and is activated by embryonic exposure to E2 [36]. By administration of E2 to genetically male eggs during the critical period of embryonic development, it is therefore possible to cause gender reversal, from male to female, in birds, including expression of the H-W antigen.
The early avian embryo has both left and right Wolffian (preseminiferous tubules) and Müllerian ducts (preovarian ducts) connecting the primordial gonads to the cloaca [37]. The Wolffian ducts serve as functional nephric ducts throughout embryonic development. During normal development in females, these ducts regress and degenerate posthatch. In males, the Wolffian ducts, influenced by testicular hormones, are incorporated into the reproductive tract. In males, the Müllerian ducts regress in the embryos under the influence of testicular hormones. In females, the right Müllerian duct regresses with only a small caudal remnant remaining posthatch, while the left duct develops into the primordial shell gland. In the absence of E2, both left and right gonads develop into testes, while during normal female development, the left gonad develops into an ovary while the right gonad regresses [38].
In both birds and mammals, the neural circuitry governing behavior differentiates at the same time as or after the gonads [39]. This differentiation is influenced by both organizational and activational effects of hormonal secretions from the gonads. Organizational effects are permanent and determine alterations that establish the fundamental form (male or female) of an organ or adult behavior. They generally occur during critical periods of development. In contrast, activational effects are not permanent, are not limited to any critical period, and typically occur at sexual maturity. While different hormones are involved in sexual differentiation in birds and mammals, E2 is the primary differentiating hormone for behavior in both groups [40]. In male rats, E2 is the active differentiating hormone for copulatory behavior. Estrogen in males is synthesized in the brain from T supplied via the blood. In birds, ovarian estrogens directly feminize and demasculinize adult copulatory behaviors [41].
In birds, administration of sex hormones early in development can have permanent sex-reversing effects on adult behavior [42]. When male quail (Coturnix japonica) embryos were injected with 1 μg E2 or 500 μg T during a critical period of incubation, their behavior was completely sex-reversed. As adults, these males fail to mount, crow, or strut, and their completely demasculinized behaviors are indistinguishable from those of females [43]. This is due to a fundamental change in the neural substrate underlying these behaviors. In quail, early exposure to E2 confers differential responsiveness to the activating effects of T in adulthood: Treatment with T restores copulation in photically castrated adult males but has no effect on copulatory behaviors in similarly castrated females [44]. Female quail treated with an anti-estrogen before hatching can be masculinized and will mount other females as adults [42]. Therefore, during normal development of quail, the absence of E2 causes the embryo to develop as a behavioral male. Testosterone-induced organizational demasculinization would require the aromatization of T to E2; the enzymatic aromatization activity in the embryonic quail brain is likely to be low and thus protects male embryos from endogenous secretions of E2 [45].
Differentiation of sexual behavior has also been studied in the zebra finch, a species exhibiting sexual dimorphism of the brain and of behavior. Normally, only male zebra finch sing, dance, and mount. Specific regions of the brain are essential for the learning and production of complex vocalizations. Therefore the telencephalic nuclei (TN), higher vocal center (HVC), nucleus robustus archistriatalis (RA), nucleus magnocellularis of the anterior neostriatum (MAN), and area X of the lobus paraolfactorius (X) are larger and more extensively connected in males [46]. The administration of exogenous estrogens to female zebra finches during the first week posthatch results in masculinization of the brain and behavior [47–49]. This includes masculinization of the TN, such that a functional circuit is created in the brains of females similar to that in the male, which enables learning and production of complex vocalizations [46]. As a result, when these masculinized female zebra finches are stimulated with testosterone as adults, they carry out male behaviors, including singing and dancing [49]. Interestingly, male zebra finches treated with estradiol during the first week posthatch are demasculinized and fail to mount as adults [49]. Thus, the pattern of behavioral sexual differentiation in the zebra finch is complex, with E2 during the first week posthatch masculinizing females and demasculinizing males. Several more recent studies have addressed subtle contaminant impacts on avian brain development and song [50–53].
The critical period for sexual differentiation of behavior varies among bird species. The sex-reversing behavioral effects of treatment with E2 occur in male Japanese quail (Coturnix japonica) only if the treatment is before day 12 of the 18-day incubation [43]. In contrast, estradiol-induced masculinization of female zebra finches is produced only by treatment after hatching [49]. Many of these apparent differences in the timing of critical events can be explained by the fact that quail are precocial (well developed at hatching) while zebra finches are altricial (poorly developed at hatching). Timing of sexual differentiation of behavior in these different groups is consistent with the observation that development processes in precocial and altricial birds proceed through the same sequence but that the two groups hatch at different times during ontogeny [42]. Thus, developmental stages that are protected from external chemical exposure in quail may be vulnerable in zebra finch since the former is still in ovo while the later have hatched and are exposed to external chemical influences. Put another way, chicks of altricial species can be exposed to chemicals in food at specific developmental stages while precocial chicks at the same stage of development are exposed only to chemicals maternally deposited in the egg. This difference in exposure, related to natural history, needs to be considered when designing studies such as the proposed avian two-generation test. Routes of exposure and the vulnerability of different developmental stages to chemical exposure might not be adequately addressed by the current single-species proposal [54].
There are several biological differences between birds and mammals that might confer differential sensitivity to estrogenic or androgenic substances during sexual differentiation. Because of differences in the default sex of these groups, exposure of an avian embryo to exogenous estrogens during the critical periods of development should have a greater effect than would exposure of a mammalian fetus to estrogens during the same critical period. This is because in birds, E2 is the differentiating hormone for both gonads and behavior. In contrast, E2 is not the differentiating hormone for gonadal development in mammals. While exposure of the mammalian fetus to E2 can cause changes in sexual characteristics, the changes are not as profound as those that would be caused by exposure to T or that alter endogenous production of T.
For the avian embryo to be exposed to exogenous estrogens during the critical period for gonadal differentiation, these compounds must be transferred to the egg from the female. Some hydrophobic estrogenic chemicals, such as o,p′-DDT and anti-androgenic chemicals such as p,p′-DDE, are readily transferred to the lipid-rich yolk. Birds can acquire substantial body burdens of these and similar contaminants from their diet, and these can then be transferred to the egg yolk [55]. However, if estrogenic substances are unable to pass from the female to the egg, the avian embryo is likely to be more protected from adverse effects than its mammalian counterpart due to its isolation from both the female and from the ambient environment. In contrast, the mammalian fetus is subject to the continuous transfer of these chemicals across the placenta [34] and, postpartum, via milk during lactation.
Avian embryos might be more susceptible than mammalian fetuses to those compounds that require metabolic activation to become endocrine-active chemicals since these chemicals are retained in the egg for the duration of incubation [38]. Water-soluble metabolic products of xenobiotics are not excreted from the avian egg; they either remain in the blood or are excreted into the allantois [56]. The availability of some compounds in the allantoic waste to be remobilized [57] suggests that excreted chemical metabolites still might be able to affect the avian embryo. Phase 1 hydroxylated transformation products of organochlorine contaminants are, in some cases, considerably more estrogenic than the parent compounds [58,59]. It has been demonstrated that during the last half of incubation, the avian embryo can produce mixed-function oxygenase enzymes [60,61] that could biotransform chemicals, including the hydroxylation of organochlorines, and yet still be within the critical period for sexual differentiation of brain and behavior. These findings clearly indicate differences in the metabolic processing and excretion of chemicals that have implications for endocrine-disruptor testing procedures comparing birds and mammals.
Given the differences in life cycle, sexual differentiation, and chemical sensitivity and susceptibility of birds compared to mammals, there is a need to incorporate avian-specific tests into current regulatory frameworks for environmental protection. This review describes existing methods for assessment of the effects of EDCs on avian species and possible future techniques. Existing techniques can be distinguished as in vivo or in vitro with in vivo techniques including egg injection, feeding studies, including multigeneration studies, and field assessment of effects at the level of either individuals or populations. In vitro techniques include the use of embryo culture primary cell cultures and stable cell lines.
10.3 IN VITRO TECHNIQUES
10.3.1 Primary Cultures
It is the general aim of in vitro techniques to provide rapid and cost-effective tools for the assessment of specific mechanisms of action while avoiding the need to use live animals. Even if tissues or cells need to be harvested from live animals, fewer animals can be used and the pain and suffering of the animals can be obviated because live animals are not exposed to the chemicals being tested. The most widely adopted methods currently used in the study of chemical effects on higher vertebrates involve the use of cell culture techniques. In the case of birds, tissues are removed from adult birds or embryos at different developmental stages. Individual cells are then isolated from tissues and are maintained in cell culture. The use of embryonic tissues at known stages of development from commercially available species greatly facilitates the preparation of cell cultures. The most commonly used tissue for these procedures is liver, producing hepatocyte cultures [62,63], although other tissues, such as heart [4], muscle [65], and tendon [66], can be used to examine effects on specific tissues.
Probably the greatest advantage of primary cell culture systems is that they permit the comparison among species and contaminants [62,67,68] when in vivo experiments are not logistically or ethically possible, such as for endangered or threatened species like the bald eagle [69]. It should, however, be remembered that some organisms need to be killed each time a primary cell culture is prepared and that suitable tissues, such as embryonic tissues, might not be available outside the organism’s normal breeding season.
Few studies have examined the effects of EDCs on endocrine tissues despite the existence of methods for preparation and culture of cells from the pituitary [70], follicle [71] and ovary [72]. The majority of studies have focused on effects in hepatocytes and expression of estrogen-dependent genes such as vitellogenin (VTG) [63]. Using these methods, it has been demonstrated that a variety of xenobiotics or environmentally derived xenobiotic mixtures can alter expression of VTG in hepatocytes. While moxestrol, E2, o,p′-DDT, 4-tert-octylphenol, and methoxychlor induced expression of VTG, BPA did not. It was also demonstrated that methoxychlor, o,p′-DDT, and tamoxifen inhibited moxestrol-induced expression of these genes, suggesting that these three compounds have anti-estrogenic potential in a similar concentration range to that in which they have estrogenic effects. How these findings relate to effects in other endocrine-active tissues such as the pituitary and ovary awaits assessment.
10.3.2 Immortal Cell Lines
In contrast to mammalian systems, the majority of studies of effects of xenobiotics on cultured cells of birds have focused on the use of primary cell cultures, although immortal avian cell cultures that respond to xenobiotics have been characterized [73,74]. The reason for this difference in emphasis between animal groups is difficult to explain but could be related to the fact that early mammalian models were used to develop an understanding of mechanisms of action and potential effects of chemicals on humans, while studies of birds have focused more on assessment of risk to a range of different avian species, particularly those for which stable immortal cell lines are not available. It is also possible that the limited number of available immortal avian cell lines has limited development in this direction. This scarcity of cell lines seems to be related to the inherent long, but still finite, life span of avian cells in culture, which results in a limited capacity for immortalization [75]. However, the development of several immortalized chicken embryo fibroblast cell lines may overcome some of these difficulties [75]. The major concern with the use of immortalized cell lines is that the alterations required to immortalize the cells might adversely affect response mechanisms to EDCs. It also remains to be seen whether stable cell lines from other avian species can be developed.
10.4 STUDIES WITH EMBRYOS
One advantage of avian models is that the embryo can be exposed by opening the eggshell for treatment and observation during development [33,76]. In this case, chemicals of interest can be applied directly to the embryo without incurring complications inherent in egg injection techniques. Using this method, the effects of both natural and the anthropogenic ER agonists nonylphenol and methoxychlor were investigated in quail embryos [77]. In this study, chemicals were administered to embryos by placing filter paper circles containing the treatment chemicals directly onto the blood vessels of the chorioallantoic membrane. This application technique results in difficulties for determining actual administered dose, but the sensitivity of the developing embryo precludes other more direct forms of exposure. Finally, although these ex ovo techniques have significantly improved over time, hatching success (i.e., carrying embryos to the final stages of development) is still considerably less than in egg injection systems. In addition, the ability of chicks to pip eggs, hatching success, as well as immediate posthatch end points cannot be determined with these ex ovo techniques. Finally, the effort and attention required to prepare eggs for embryo exposure and the care needed during incubation preclude use of these techniques as routine high-throughput chemical screening procedures.
10.5 IN VIVO TECHNIQUES
In vivo studies of the effects of EDCs on birds can be conducted at various complexities ranging from laboratory-based egg injection studies to population-level ecological investigations. For this review, we consider egg injection studies to be in vivo since, apart from the chemical introduction, egg laying, incubation, and hatching proceed in a normal developmental fashion. In contrast, the above-mentioned embryo exposure techniques are considered to be in vitro, since manipulations of the egg normally would result in death of the embryo without artificial life support mechanisms. An advantage of avian species is that they produce relatively large eggs that can be removed from the parents and incubated in isolation. Therefore, the developing embryo is not susceptible to toxic effects in the female, as would happen in species with internal gestation. It is also relatively easy to introduce chemicals of interest into the egg by injection or even by surface application, where this route of exposure is relevant such as for field-sprayed pesticides [78] or fire retardants used in aerial firefighting applications [79]. Injection techniques include injection into yolk, albumen, or air sac depending on chemical properties and experimental design [80]. A variety of techniques for injection of eggs have been evaluated, and best practices are understood with reasonable certainty [81]. For some studies, it is possible to incubate the embryo in a partially opened egg and so directly observe effects of introduced chemicals on development [82]. Egg injection studies are applicable to a variety of avian species and have been used in a number of studies to determine relative species sensitivities to various compounds [83–85]. Egg injection studies also provide a useful link between other in vivo techniques and cell-based in vitro systems [86]. Viable, fertilized eggs can be obtained on a year-round basis for some commercially significant species in comparison to some wildlife species for which eggs might be available only from field collections made during the natural breeding season. However, it must be remembered that the majority of commercially available eggs come from precocial species and so findings regarding EDCs and development may be less applicable to altricial species. In particular, altricial nestlings require extensive care posthatch, care that is usually provided by parent birds. This parental care is a critical segment of the life cycle, and chemicals able to interfere with these types of behaviors can adversely affect reproductive output [87,88].
Each of the in vivo approaches currently used in assessment of risks to birds has associated advantages and disadvantages [89]. While pure chemical screening assays, such as egg injection or oral exposure studies, can accurately control many variables and so produce large amounts of very consistent data, their applicability to field exposures in wild organisms can be limited. Therefore, while these studies are comparatively inexpensive, their findings are largely limited to the specific biochemical and physiological variables evaluated and might be of only limited utility in predicting effects on wild populations. It should also be remembered that egg injection studies remove any effects of parental behaviors as confounding variables, an advantage for the assessment of direct embryo impacts but a considerable disadvantage in relating the results to any real-life exposure scenario. In contrast, field ecological studies are capable of determining population-level effects but, due to interactions between chemicals and impacts of other environmental factors and stressors, it can be difficult to demonstrate cause–effect relationships. In field studies, it can also be difficult to accurately determine exposure of the population or even to determine relevant metrics of exposure, such as intensity, duration, or frequency. Field ecology studies are also more retrospective than predictive since they can in essence determine effects only after the chemical has been released to the environment. Often population-level effects are not detectable for significant periods of time after a chemical enters commercial use, particularly if food chain accumulation is required before significant effects can be observed in higher-trophic-level populations. Therefore, applicability of true ecological field studies is limited in prediction of environmental impacts before commercialization and release of chemicals. The only exception to this generalization may be that data obtained during the assessment of population-level effects of chemicals with comparable environmental chemodynamic and toxicological properties might be of use in predicting adverse effects of new chemicals. As a compromise or hybrid approach, between laboratory exposures and field ecology, controlled field exposure or mesocosm studies can provide more environmentally relevant exposure scenarios and can account for effects of other environmental stressors. While more logistically demanding than laboratory exposures, these studies offer the possibility of determining dose-related ecologically relevant end points while controlling all other variables. There are, of course, limitations of the size of the mesocosm area, which must, as a consequence, prevent assessment of some ecological variables, such as migration and predation. In addition, the area required for each mesocosm and logistics of maintaining it can lead to limited replication in this type of study.
In a variety of controlled mesocosm or caging studies, different organochlorine chemicals have been demonstrated to alter avian reproductive behavior. Adult ring doves (Streptopelia risoria) fed a mixture of organochlorines exhibited alterations in hormone concentrations and reproductive behavior [90]. Consumption of contaminants led to a reduction and/or delay in behaviorally induced increases of sex hormones, contaminated females failed to respond to male courtship behaviors, and pairs receiving the greatest dose spent less time feeding the young. There was a dose-dependent decrease in fledging success, and the breeding cycle was greatly asynchronous in treated birds. Administration of a polychlorinated biphenyl (PCB) mixture to adult breeder doves resulted in aberrant incubation [88] and courtship [91] behaviors. PCB-dosed females were particularly affected in the later experiment, performing only a small number of courtship behaviors, resulting in impairment of reproductive success.
Other environmental contaminants have also been demonstrated to have negative effects on avian reproduction due to effects on endocrine function. Oral administration of crude oil caused elevated plasma corticosterone and thyroxine levels in herring gull (Larus argentatus) and black guillemot (Cepphus grylle) nestlings, apparently related to depressed growth [92]. Exposure to the organophosphorus insecticide parathion can also affect avian incubation behavior and reproductive success [93,94], although such effects are likely to be more acute and transient in nature than organochlorine-induced reproductive impairment. Natural environmental estrogens, such as phytoestrogens in the diet, can also influence avian reproduction. California quail (Callipepla californica) experienced a delayed onset of reproduction and decreased egg production when a plant extract containing isoflavones, such as genistein, was incorporated into their diet [95]. Recent studies of some avian species, however, indicate that the presence of estrogenic compounds in the diet is a prerequisite for successful reproduction. In the case of the endangered New Zealand kakapo (Strigops habroptila), a flightless parrot, it is believed that ingestion of a sufficient dose of estrogenic compounds is a signal of a year of adequate food abundance for reproduction and is a prerequisite to breeding [96].
10.5.1 Egg Injection Studies
The largest portion of toxicology studies involving avian species have been by injecting substances into the egg, into the air cell or albumen or directly into the yolk sac [97]. Eggs can be injected early in development or at specific times to capture effects on specific developmental processes. After injection, eggs are sealed and placed in incubators adjusted to provide species-specific incubation requirements for temperature, humidity, and turning of eggs. Considerations of variables and the development of standardized egg injection protocols have recently been evaluated [81,98]. During and after the incubation period, embryos can be sampled for a variety of end points [80]. While egg injection studies dominate the in ovo studies reported in the literature, some studies have used topical application of chemicals to the egg surface, when this route of exposure is potentially relevant to the chemical being tested, such as for field-sprayed organophosphate insecticides [78]. The location of injection can alter chemical effects as differences in the sensitivity of chicks have been observed between air sac and yolk injections of 2,3,7,8-TCDD [99].
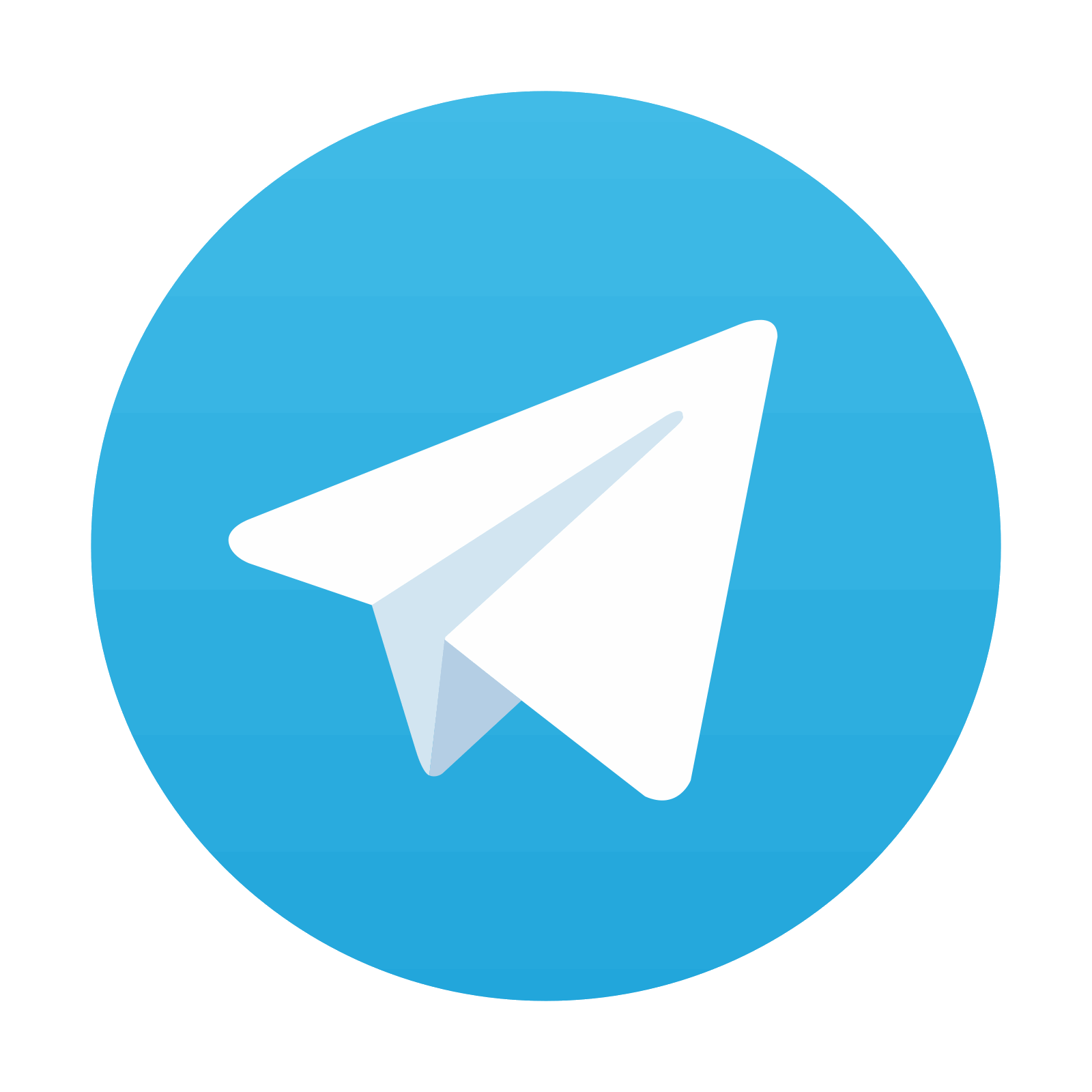
Stay updated, free articles. Join our Telegram channel
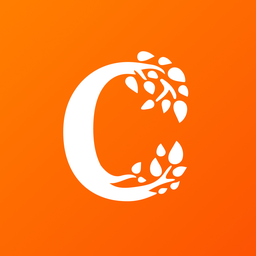
Full access? Get Clinical Tree
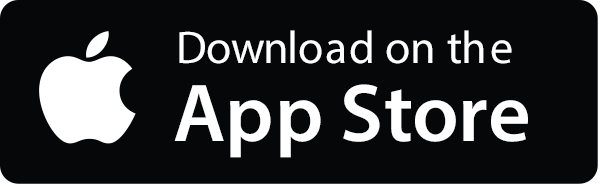
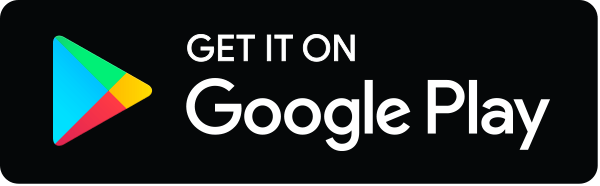