Basic Concepts of Chemotherapy and Irradiation Interaction
For decades, radiation therapy has been a major treatment modality for locally or regionally confined cancers. The rate of treatment failure is still high, particularly for large tumors or advanced disease. Technologic improvements in radiation therapy have continuously been made that allow delivery of higher radiation doses to the tumor or lower doses to normal tissues, and in the implementation of strategies that modulate the biologic response of tumors or normal tissues to radiation. These strategies include altered fractionation scheduling including extreme hypofractionation (e.g., stereotactic body radiotherapy, stereotactic radiosurgery), combined-modality treatments using chemical or biologic agents, and, more recently, targeting molecular processes and signaling pathways that have become dysregulated in cancer cells.
The combination of chemotherapeutic drugs with radiation has perhaps had one of the strongest impacts on current cancer radiation therapy practice. This is particularly true for concurrent chemoradiation therapy, which has been shown in many recent clinical trials to be superior to radiation therapy alone in controlling locoregional disease and in improving patient survival. Combining chemotherapeutic drugs with radiation therapy has a strong biologic rationale. Such agents reduce the number of cells in tumors undergoing radiation therapy by their independent cytotoxic action and by rendering tumor cells more susceptible to killing by ionizing radiation. An additional benefit of combined treatment is that chemotherapeutic drugs, by virtue of their systemic activity, may also act on metastatic disease. Most drugs have been chosen for combination with radiation therapy based on their known clinical activity in particular disease sites. Alternatively, agents that are effective in overcoming resistance mechanisms associated with radiation therapy could be chosen. There have been clinical successes with concurrent chemoradiation therapy using traditional drugs, such as cisplatin and 5-fluorouracil (5-FU), and these studies have led to extensive research on exploring newer chemotherapeutic agents for their interactions with radiation. A number of potent chemotherapeutic agents, subsequently, have entered clinical trials or practice. These agents were selected after strong preclinical studies demonstrated that they are potent enhancers of the radiation response and thus might further improve the therapeutic outcome of chemoradiation therapy. Also, there are rapidly emerging molecular targeting strategies aimed at improving the efficacy of radiation therapy.
This chapter reviews the biologic rationale and principles fundamental to the use of chemotherapy and molecular targeted agents in conjunction with radiation treatments and discusses mechanistic interactions between drugs and radiation, the knowledge of which is essential in developing the optimal treatment strategies and designing appropriate clinical trials. It also provides a brief overview of current treatment applications and advances in the clinic. Owing to limited space, this review is far from comprehensive; additional information can be found in other reviews on this subject.1,2,3–4,5
THERAPEUTIC INDEX
Both radiation and chemotherapeutic drugs are cytotoxic to tumor and normal tissue cells. This lack of specificity is a major limitation in their use when applied either as individual treatments or in combination. Radiation inflicts damage to tumor and normal tissues in the radiation treatment field, whereas drugs, because of their systemic action, can affect any tissue in the body. Damage is often accentuated when the two agents are combined and when they affect the same tissue. In general, both the antitumor effectiveness and the severity of normal-tissue damage produced by either radiation or drugs are increased as their dose is increased. This dose–effect relationship is sigmoidal and enables estimation of the therapeutic index (ratio), which is defined as the ratio between the doses (radiation, drug) that produce the same level (probability) of antitumor efficacy and normal-tissue damage. To be therapeutically beneficial, the therapeutic ratio must be positive (>1); that is, individual agents or their combination must be more effective against tumors than normal tissues. To define therapeutic benefit in clinical settings, many factors must be taken into account, such as whether the treatment is curative or palliative, which tissues are dose limiting (critical tissues), what degree of tissue damage is acceptable, and so forth. The balance between a given level of antitumor efficacy and acceptable normal-tissue complications gives a measure of the therapeutic ratio of a treatment.
EXPLOITABLE STRATEGIES IN CHEMORADIATION THERAPY
The goals of combining chemotherapeutic drugs with radiation therapy are to increase patient survival by improving locoregional tumor control, decrease or eliminate distant metastases, or both, while preserving organ and tissue integrity and function. Combined-modality treatment can further improve positive therapeutic outcome of individual treatments through a number of specific strategies, which Steel and Peckham6 classified into four groups: “spatial cooperation,” independent toxicity, enhancement of tumor response, and protection of normal tissues.
Spatial cooperation was the initial rationale for combining chemotherapy with radiation therapy, in which the action of radiation and chemotherapeutic drugs is directed toward different anatomic sites. Localized tumors would be the domain of radiation therapy because large doses of radiation can be given. On the other hand, chemotherapeutic drugs are likely to be more effective in eliminating disseminated micrometastases than in eradicating larger primary tumors. Thus, the cooperation between radiation and chemotherapy is achieved through the independent action of two agents. Spatial cooperation is the basis for adjuvant chemoradiation therapy, in which radiation is given first to control the primary tumor and chemotherapy is given later to cope with micrometastases. The concept of spatial cooperation is also applied in the treatment of hematologic malignancies that have spread to “sanctuary” sites, such as the brain. These sites are poorly accessible to chemotherapeutic agents, and thus they are more appropriately treated with radiation therapy.
Independent toxicity is another important strategy for increasing the therapeutic ratio of chemoradiation therapy. Normal-tissue toxicity is the main dose-limiting factor for both chemotherapy and radiation therapy. Therefore, combinations of radiation and drugs would be better tolerated if drugs were selected such that toxicities to specific cell types and tissues do not overlap with, or minimally add to, radiation-induced toxicities. This strategy requires a thorough knowledge of drug toxicity, underlying mechanisms, and drug pharmacokinetics. Another strategy in chemoradiation therapy is to exploit the ability of chemotherapeutic agents to enhance tumor radioresponse. The enhancement denotes the existence of some type of interaction between drugs and radiation at the molecular, cellular, or pathophysiologic (microenvironmental, metabolic) level, resulting in an antitumor effect greater than would be expected on the basis of additive actions. Many mechanisms may be involved in drug–radiation interactions leading to tumor radio enhancement, and some of them are elaborated on further in the text. The enhancement must be selective or preferential to tumors compared with critical normal tissues to achieve therapeutic gain. The ability of chemotherapeutic agents to enhance tumor radioresponse by counteracting determinants associated with tumor radioresistance is a major rationale for concurrent radiation therapy.
An additional strategy is to protect normal tissues so that higher doses of radiation can be delivered to the tumor. This can be achieved through technical improvements in radiation delivery or administration of chemical or biologic agents that selectively or preferentially protect normal tissues against the damage by radiation or drugs. A separate section in this chapter discusses radioprotectors in more detail.
ASSESSMENT OF DRUG–RADIATION INTERACTION
Any drug considered for use in combination with radiation therapy needs to undergo preclinical evaluation for its interaction with radiation both in in vitro cell culture systems and in vivo, with the aim of assessing antitumor activity and normal-tissue toxicity. The interaction between two agents is more easily defined and quantified in vitro because complete cell survival curves are readily obtained. The in vitro cell survival assay measures the ability of cells to produce colonies of a defined minimum size. Cell survival is determined after treatment with a drug or radiation alone, given at different doses, or after treatment with both agents, in which case the cells are exposed to the drug before, during, or after irradiation. Survival curves are usually plots of the surviving fraction of cells on a logarithmic scale and the dose of radiation or drugs on a linear scale.
The cell survival curve after irradiation characteristically has a “shoulder” of varying width that denotes the capacity of cells to repair radiation damage. The curves that describe survival after chemotherapeutic agents show much more variation both in absolute sensitivity to drugs and their shape than those after radiation, all depending on the drug tested. Some curves possess shoulders, some lack them, and some show resistant “tails” at higher drug doses. The tails denote the existence of cell subpopulations resistant to chemotherapeutic agents.
To assess the effect of the drug on cell radiosensitivity, the combined drug–radiation curve is commonly plotted after the cytotoxicity produced by the drug alone is excluded (“normalized”). The radiation cell survival curve is not changed if the drug does not influence cell radiosensitivity regardless of whether the drug is cytotoxic on its own. In this case, the cytotoxicity of the drug contributes only to the overall cell killing by the combined treatment (additive effect) of both agents. Chemotherapeutic agents may interact with radiation by altering cell radiosensitivity such that the combination results in a supra-additive or subadditive effect, depending on whether the cell killing is greater or smaller than the sum of cell killings produced by individual agents. Drugs may eliminate the shoulder on the radiation survival curve, implying that drugs can inhibit cell repair from radiation damage, or they may change the slope of the exponential portion of the survival curve. A steeper slope indicates increased sensitization to radiation, whereas a shallower slope indicates protection.
Because of nonlinear dose-related characteristics in cell killing by both chemotherapeutic agents and radiation, the effects of the combined treatment are best assessed using the “isobologram,” an isoeffect plot for the dose response to the combination of two agents6 (Fig. 32.1). Dose–response curves are determined for each agent to generate the isobologram, an envelope of additivity, which denotes expected additive response over a range of doses of the agents used. If the interaction between drugs and radiation is supra-additive or synergistic (i.e., the effect is caused by lower doses of the two agents than the envelope of additivity would predict), the effect is shown at the left side of the envelope. In contrast, the effect of the subadditive or antagonistic interaction is shown to the right of the envelope: the effect required higher doses of the two agents than predicted. The width of the envelope of additivity depends on the degree of the nonlinearity in the dose response to individual agents. The envelope is wider as the degree of nonlinearity increases. In the case of a linear dose–response relationship for each agent, which is rare, the isobologram is also linear, represented by a single straight line.
In vitro testing is often followed by in vivo exploration of drug–radiation interactions, which allows assessment of the combined treatment on both tumors and normal tissues. This is essential for determination of therapeutic gain, as discussed earlier in this chapter. Syngeneic animal tumors or human tumor xenografts in nude mice are most often used for this purpose. The efficacy of the treatment is determined by the extent of tumor growth delay or the rate of tumor cure. In normal tissues, the effect of chemotherapeutic drugs on radiation response of acutely and late-responding tissues can be assessed using a variety of available assays. Some of these assays are clonogenic, such as the jejunal crypt assay, where the end point depends directly on the reproductive integrity of individual cells. More frequently, however, dose–response relationships for normal tissues are based on functional end points (such as breathing rate in lung damage and paralysis in spinal cord damage). These end points tend to reflect the minimum number of functional cells remaining in tissues or organs and not the proportion of cells retaining reproductive integrity.
FIGURE 32.1. An isobologram for two agents when their dose–response curves are nonlinear. The isobologram shows the envelope of additivity and regions of supra-additivity and subadditivity. (Modified from Steel GG, Peckham MJ. Exploitable mechanisms in combined radiotherapy-chemotherapy: the concept of additivity. Int J Radiat Oncol Biol Phys 1979;5:85–91, with permission. Copyright 1979 by Elsevier Science, Inc.)
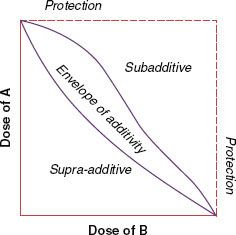
MECHANISTIC CONSIDERATIONS IN DRUG–RADIATION INTERACTIONS
Increasing Initial Radiation Damage
Radiation induces many different lesions in the DNA molecule, which is the critical target for radiation damage. The lesions consist of single-strand breaks (SSBs), double-strand breaks (DSBs), base damage, DNA–DNA and DNA–protein cross-links, and so forth. DSBs and chromosome aberrations that occur in association with or as a consequence of DSBs are usually considered to be the principal damage that results in cell death.7 Any agent that makes DNA more susceptible to radiation damage may enhance cell killing. Certain drugs, such as halogenated pyrimidines, incorporate into DNA and make it more susceptible to radiation damage.8
Inhibition of Cellular Repair
Both sublethal9 and potentially lethal10,11 damage inflicted by radiation can be repaired. Although sublethal damage repair (SLDR) denotes the increase in cell survival when the radiation dose is split into two fractions of radiation separated by a time interval, potentially lethal damage repair (PLDR) designates the increase in cell survival as the result of postirradiation environmental conditions. SLDR is rapid, with a half-time of approximately 1 hour, and is complete within 4 to 6 hours after irradiation. This time between two radiation fractions allows radiation-induced DSBs in DNA to rejoin and repair. SLDR is expressed as the restitution of the shoulder on the cell survival curve for the second dose. PLDR occurs when environmental conditions prevent cells from dividing for several hours, such as keeping in vitro growing cells in plateau phase after irradiation. Preventing cells from division allows the completion of repair of DNA lesions that would have been lethal had DNA undergone replication within several hours after irradiation. PLDR is considered to be a major determinant responsible for radioresistance in some tumor types, such as melanomas. The repair can be achieved through restoration of damaged molecules by reducing species that donate electrons to oxidized substrates or through involvement of enzymes mediating homologous and nonhomologous recombination repair of DNA DSBs, base excision repair of base damage, and nucleotide excision repair of DNA–protein cross-links.
Many chemotherapeutic agents used in chemoradiation therapy interact with cellular repair mechanisms and inhibit repair, and hence may enhance cell or tissue response to radiation. The aforementioned halogenated pyrimidines enhance cell radiosensitivity not only through increasing initial radiation damage but also by inhibiting cellular repair.8,12 Nucleoside analogs, such as gemcitabine, are a class of chemotherapeutic agents potent in inhibiting the repair of radiation-induced DNA and chromosome damage.13,14 They have been shown strongly to enhance tumor radioresponse in preclinical studies and have been, and are continuing to be, extensively investigated for such activity in patients with cancer.15,16
Cell Cycle Redistribution
Both chemotherapeutic agents and radiation are more effective against proliferating than nonproliferating cells. Their cytotoxic action further depends on the position of cells in the cell cycle. Cell cycle dependency in response to radiation was first described almost 40 years ago.17 Terasima and Tolmach.17 reported that the sensitivity of the cell response to radiation varied widely depending on which phase of the cell cycle the cells were in at the time of irradiation, and that cells in the G2 and M cell cycle phases were approximately three times more sensitive than cells in the S phase. The exact reason for this variability is still unknown.
The influence of the cell cycle on cell response to cytotoxic agents can be therapeutically exploited in chemoradiation therapy using cell cycle redistribution strategies. For example, some chemotherapeutic drugs, such as taxanes, can block transition of cells through mitosis, with the result that cells accumulate in the radiosensitive G2 and M phases of the cell cycle. Radiation delivered at the time of significant accumulation of cells in both the G2 and M phases results in enhanced radioresponse of cells in vitro18,19 and of tumors in vivo.20,21 However, this cell cycle mechanism of taxane-induced enhancement of tumor radioresponse is dominant only in tumors that are resistant to paclitaxel or docetaxel as a single treatment. Although tumor growth in taxane-resistant tumors is not substantially affected by the drug, tumors do exhibit significant transient accumulation of cells in mitosis 6 to 12 hours after the treatment.21 Taxanes also enhance the radioresponse of tumors that respond by significant tumor growth delay to taxanes given as a single treatment modality, but a major mechanism for radio enhancement in such tumors is reoxygenation of radioresistant hypoxic cells, as discussed later.20
Elimination of the radioresistant S-phase cells by the chemotherapeutic agents may be another cell cycle redistribution strategy in chemoradiation therapy. Nucleoside analogs, such as fludarabine or gemcitabine, are good examples of the agents that become incorporated into S-phase cells and eliminate them by inducing apoptosis.13,15 In addition to purging S-phase cells, the analogs induce the surviving cells to undergo parasynchronous movement to accumulate in the G2 and M phases of the cell cycle between 1 and 2 days after drug administration, a time when the highest enhancement of tumor radioresponse was observed.15 Tumors with a high cell growth fraction are likely to respond better to the cell cycle redistribution strategy in chemoradiation therapy than tumors with a low cell growth fraction.
Counteracting Hypoxia-Associated Tumor Radioresistance
Solid malignant tumors usually are characterized by defective vascularization, both in the number of blood vessels and vessel function. Because of this, blood supply to tumor cells is inadequate, cells lack oxygen and nutrients, and multiple tumor microregions become hypoxic, acidic, and eventually necrotic. Hypoxia occurs at distances from blood vessels larger than 100 to 150 μm. The hypoxic cell content in tumors varies widely and can be more than 50%. The presence of hypoxia makes tumors more aggressive (hypoxia is conducive to the emergence of more virulent tumor cell variants and stimulates metastatic spread22,23) and more resistant to radiation as well as most chemotherapeutic agents. Hypoxic cells are 2.5 to 3 times more resistant to radiation than well-oxygenated cells. The fact that hypoxia may be a cure-limiting factor in radiation therapy, at least in some clinical situations, is suggested by the findings that reduced hemoglobin levels24 and low tumor pO225,26 are associated with higher treatment failure rates. Also, there are reports showing that local tumor control by radiation therapy can be improved by the use of hypoxic cell radiosensitizers27 or hyperbaric oxygen.28,29 With respect to the effects of chemotherapy, hypoxic regions are less accessible to chemotherapeutic drugs; in addition, hypoxic tumor cells are either nonproliferating or they proliferate poorly, and as such do not respond well to drugs.
Combining chemotherapeutic agents with radiation therapy can reduce or eliminate hypoxia or its negative influence on tumor radioresponse. Most chemotherapeutic drugs preferentially kill proliferating cells, primarily found in well-oxygenated regions of the tumor. Because these regions are located close to blood vessels, they are easily accessible to chemotherapeutic agents. Destruction of tumor cells in these areas leads to an increased oxygen supply to hypoxic regions, and hence reoxygenates hypoxic tumor cells. Massive loss of cells after chemotherapy lowers the interstitial pressure, which then allows the reopening of previously closed capillaries and the re-establishment of blood supply. It also causes tumor shrinkage so that previously hypoxic areas are closer to capillaries and thus accessible to oxygen. Finally, by eliminating oxygenated cells, more oxygen becomes available to cells that survived chemotherapy. It was recently shown that tumor reoxygenation is a major mechanism underlying the enhancement of tumor radioresponse induced by taxanes in tumors sensitive to these drugs.20
Another approach to counteract the negative impact of hypoxia is selective killing of hypoxic cells through bioreductive drugs, such as tirapazamine,22 which undergo reductive activation in a hypoxic milieu, rendering them cytotoxic. A related possibility is to exploit the acidic state (low pH) of tumors, which develops as a result of hypoxia-driven anaerobic metabolism that produces lactic acid,30 through the use of drugs that selectively accumulate in acidic environments or become activated by a low pH.31
The use of agents that selectively radiosensitize hypoxic cells to reduce their negative impact has been considered and tested in clinical trials for some time. These drugs increase radiation damage by mimicking the effect of oxygen. Many clinical trials have tested these drugs, particularly misonidazole, in combination with radiation therapy, but few of them have shown an improved treatment outcome. The exception is nimorazole, which also does not elicit the neurotoxicity commonly associated with hypoxic cell sensitizers that prevented the delivery of clinically effective doses of these agents.32
Inhibition of Tumor Cell Repopulation
The constant balance between cell production and cell loss maintains the integrity of normal tissues. When this balance is perturbed by cytotoxic action of chemotherapeutic drugs or radiation, the integrity of tissues is re-established by an increased rate of cell production. The cell loss after each fraction of radiation during radiation therapy induces compensatory cell regeneration (repopulation), the extent of which determines tissue tolerance to radiation therapy. In contrast to normal tissues, malignant tumors are characterized by an imbalance between cell production and cell loss in favor of cell production. As with normal tissues, tumors also respond to radiation- or drug-induced cell loss with a compensatory regenerative response. Preclinical studies provided ample evidence demonstrating that the rate of cell proliferation in tumors treated by radiation or chemotherapeutic drugs is higher than that in untreated tumors.33–35 This increased rate of treatment-induced cell proliferation is commonly termed accelerated repopulation. Accelerated repopulation of tumor clonogens has been shown to occur during clinical radiation therapy as well. Withers et al.36 showed that the total dose of radiation needed to control 50% of head and neck carcinomas progressively increased with time whenever radiation therapy treatment was prolonged beyond 1 month. This increase in radiation dose required to achieve tumor control was greater than what would be anticipated based on the pretreatment tumor volume doubling time of approximately 60 days for head and neck tumors. The increase was attributed to accelerated repopulation, and it was estimated to average approximately 0.6 Gy/day,36 but may be as high as 1 Gy/day.37
Although accelerated cell proliferation is beneficial for normal tissues because it spares them from radiation damage, it has an adverse impact on tumor control by radiation therapy or chemotherapy. Chemotherapeutic drugs, because of their cytotoxic or cytostatic activity, can reduce the rate of proliferation when given concurrently with radiation therapy, and hence increase the effectiveness of the treatment. Caution must be taken to select drugs that preferentially affect rapidly proliferating cells and preferentially localize in malignant tumors. However, the main limitation of concurrent chemoradiation therapy is the enhanced toxicity of rapidly dividing normal tissues because most available chemotherapeutic agents show poor tumor selectivity. Moreover, accelerated repopulation induced by chemotherapeutic drugs may have a negative influence on the outcome of tumor response to radiation when drugs are used in induction or neoadjuvant chemotherapy protocols. In this strategy, chemotherapy precedes radiation therapy. Treatment outcomes after induction chemotherapy followed by radiation therapy have not been overly encouraging in terms of both local tumor control and patient survival, even if a large proportion of tumors initially responded with total or partial clinical regression by the time of radiation therapy implementation. Some experimental evidence suggests that the drug-induced accelerated cell repopulation can actually make the tumor more difficult to control with radiation.33,34
Other Potential Interactions
Molecular Signaling Pathways That May Be Responsible for Radioresistance. Significant strides have been made in elucidating molecular pathways that may be involved in resistance to cytotoxic therapy including radiation treatments. These molecular determinants are being scrutinized in preclinical and clinical settings as potential targets for cancer therapy, of which some agents are being studied for the potential for enhancing radiation effects.38 For example, the efficacy of combining the epidermal growth factor receptor (EGFR) inhibitor cetuximab with radiation therapy has been demonstrated in randomized clinical studies in head and neck cancer patients.39 Molecular targeting has become an immensely important topic, and effective integration of these strategies with radiation therapy to foster improved efficacy of therapy is an active area of investigation. This topic is further discussed in significant detail in the section to follow.
Targeting the Tumor Microenvironment. Tumor microenvironment has been postulated as being a potential therapeutic target for cancer therapy. Tumor microenvironment is a complex system of many cell types including endothelial cells, smooth muscle cells, fibroblasts, and cells involved with the immune system (lymphocytes, macrophages, etc.).40 Among these different cells that make up the different components of the tumor microenvironment, the most studied preclinically and clinically are the cells that make up the tumor microvasculature. Preclinical studies have suggested the potential role for radiosensitization of the tumor microvasculature using compounds directed at targeting angiogenesis.41 Clinical studies have been performed with efforts to combine antiangiogenic agents with radiation therapy, with mixed results.41 Studies are ongoing, as many antiangiogenic agents are approved for cancer therapy, and are detailed in the section on antiangiogenesis agents.
Cancer Stem Cells. Cancer stem cells are defined as being cells within a tumor that possess the capacity to self-renew and generate the heterogeneous lineages of cancer cells that make up the tumor.42 Cancer stem cells as a source of radiation resistance for solid tumors is an area of active investigation. Potential for the need to eradicate cancer stem cells for effective cure by radiotherapy has been postulated, and while radioresistance of cancer stem cells has been noted in in vitro studies,43 this notion has been challenged as some have demonstrated radiosensitivity of cancer stem cells.44 But, if in fact cancer stem cells are radioresistant, one possible strategy may be to identify molecular pathways that could influence cancer stem cell radiosensitivity and investigate the use of such agents in combination with radiotherapy at different stages during the course of therapy.45
TABLE 32.1 ADVANTAGES AND DISADVANTAGES OF DIFFERENT CHEMORADIATION SEQUENCING STRATEGIES
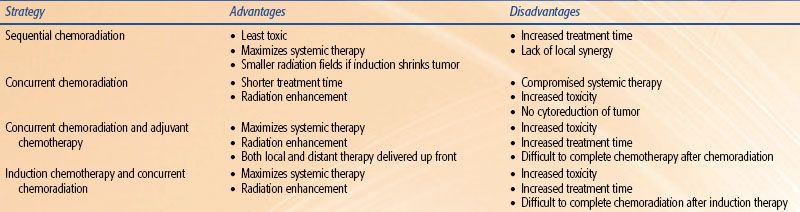
TABLE 32.2 MECHANISMS OF CHEMOTHERAPY-INDUCED RADIATION SENSITIZATION
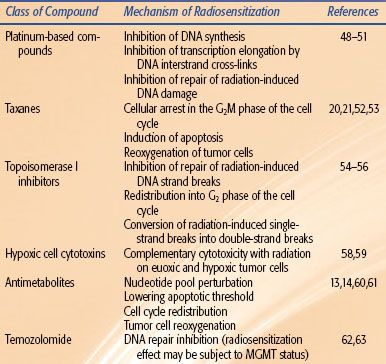
TIMING OF DRUG ADMINISTRATION IN RELATION TO RADIATION THERAPY
Most clinical chemoradiation therapy regimens evolved empirically: increasingly, information from preclinical studies is being considered in planning the optimal timing of drug administration in relation to radiation therapy. Depending on the principal aim of the therapy, drugs are administered before (induction or neoadjuvant chemotherapy), during (concurrent or concomitant chemotherapy), or after (adjuvant chemotherapy) the course of radiation therapy. The advantages and disadvantages of each approach are summarized in Table 32.1.
In regard to the primary tumor, induction chemotherapy may reduce the number of clonogenic cells and cause the reoxygenation of the surviving hypoxic cells, both of which render tumors more controllable by radiation. In addition, chemotherapy-induced tumor shrinkage may allow the use of smaller radiation fields, in which case less normal tissue is exposed and damaged by radiation. This treatment approach is often used in the therapy of solid tumors in children and of lymphomas. Induction chemotherapy precedes radiation therapy for a few weeks to a few months, which improves tolerability of the combined treatment.
Induction chemotherapy has resulted in therapeutic improvement in a number of clinical trials compared with radiation therapy, but in general the therapeutic benefits are below expectations. A number of factors could account for this, including accelerated proliferation of tumor cell clonogens and selection or induction of drug-resistant cells that are cross-resistant to radiation. The preclinical findings provide solid evidence for the existence of accelerated repopulation in tumors treated with chemotherapeutic agents. On the other hand, although development of drug resistance is a significant problem in chemotherapy, the evidence that cells that acquire drug resistance are also resistant to radiation is not convincing.
When chemotherapy is given during a course of radiation therapy, it is referred to as concurrent chemotherapy. This form of treatment is intended to cope with both disseminated lesions and the primary tumor, but it takes advantage of drug–radiation interactions to maximize tumor radioresponse. The drug scheduling in relation to individual radiation fractions is highly important, and the selection of optimal timing of drug administration must be based on mechanisms of tumor radio enhancement by a given drug, the drug’s normal tissue toxicity, and the conditions under which the highest enhancement is achieved. The data from preclinical studies can greatly contribute to the selection of the most optimal schedules. For example, it has been demonstrated that murine tumors sensitive to taxanes show enhanced radioresponse, but the best effect is achieved if drug treatment precedes radiation by 1 to 3 days.20 A major mechanism for tumor radio enhancement was reoxygenation of hypoxic cells. Based on this preclinical information, one would anticipate that in clinical protocols such tumors would best respond to a bolus of a taxane given once or twice weekly during radiation therapy. In contrast, tumors resistant to taxanes on their own would call for daily administration of a taxane because they show accumulation of radiosensitive G2– and M-phase cells 6 to 12 hours after drug administration. If the objective is to counteract rapid repopulation of tumor cell clonogens induced by radiation, then administration of cell cycle–specific chemotherapeutic agents during the second half of radiation therapy, when accelerated repopulation is more expressed, might be more effective. At present, the enhancement in normal-tissue complications remains the major limitation of concurrently combining chemotherapy with radiation therapy. Nevertheless, as is made evident later in the text, concurrent chemoradiation therapy has provided better clinical results both in terms of local tumor control and patient survival than have other modes of chemoradiation therapy combinations.46,47
Adjuvant chemotherapy designates a treatment modality in which chemotherapeutic drugs are given some time after completion of radiation therapy. The primary objective is to eradicate disseminated disease; however, the control of the primary tumor may also be improved by the ability of drugs to deal with tumor cells that survived radiation.
INTERACTION OF SPECIFIC CHEMOTHERAPIES AND RADIATION IN THE TREATMENT OF CANCER
This section provides an overview of the evidence that exists for combining particular chemotherapies with radiation. In many cases, the level of support that exists for combined therapy mirrors the age of the drug. However, as would be expected, newer drugs have generally been subject to more rigorous preclinical assessment of their efficacy before their introduction into the clinical setting (Table 32.2).
Platinum-Based Drugs
This group of compounds, distinguished from most others by its metallic element base, has come to be recognized as one of the most potent chemotherapies available to date. Cisplatin (cis-diamminedichloroplatinum II), which is the prototype drug, has been acknowledged to be a potent radiosensitizer for many years and has a significant role in clinical practice to date. Preclinical work done using murine models by Rosenberg et al.64 in the late 1960s showed that cisplatin is an effective antitumor chemotherapy. Subsequent efforts have shown that its primary mechanism of inhibition of tumor growth appears to involve the inhibition of DNA synthesis.65,66 Another secondary mechanism includes the inhibition of transcription elongation by DNA interstrand cross-links.67
Work on nonmammalian systems first demonstrated the radiosensitizing abilities of platinum-based compounds.68–70 This was confirmed in several mammalian systems as well.48,71,72 This makes inherent sense because these platinum compounds have a high electron affinity and react preferentially with hydrated electrons. The exact mechanism for the increased cell death seen with combinations of ionizing radiation and platinum drugs is not known for certain; however, the evidence would seem to point to the inhibition of PLDR49 and to the radiosensitization of hypoxic tumor cells.73 Cisplatin free radical–mediated sensitization may involve the ability to scavenge free electrons formed by the interaction between radiation and DNA. The reduction of the platinum moiety may serve to stabilize DNA damage that would otherwise be repairable.74 Greater than additive effects of cisplatin and radiation are seen in tumor models most reliably when the drug is administered with fractionated radiation,74 explained by its inhibition of SLDR.
Carboplatin, a second-generation platinum compound with a different toxicity profile, has also been studied as a radiosensitizer.50,51 Its potential efficacy as a radiosensitizer has allowed for its incorporation into regimens used in several randomized trials. Interest exists in the combination of radiation with other platinum analogs, including oxaliplatin75 as well as orally administered compounds like satraplatin.76–78
Oxaliplatin, although sharing a similar mode of action as other platinum compounds, has been shown to have activity in cisplatin-resistant systems in vitro.4 One potential explanation for this is that oxaliplatin is not affected by loss of mismatch repair, which leads to cisplatin resistance in vitro,79 and there are those who postulate that adducts formed by oxaliplatin are less well recognized by DNA repair systems.80 Whether oxaliplatin is truly active against cisplatin-resistant cancer clinically is an area of active investigation,81 and after encouraging findings in phase I to II rectal cancer studies, it entered into phase III studies, some of which have closed to accrual.80
Antimicrotubules
Taxanes
The radiosensitizing properties of plant-derived chemotherapeutic agents, the taxanes, have been studied extensively in both preclinical models and in clinical trials. Paclitaxel (Taxol) and docetaxel (Taxotere) act as mitotic spindle inhibitors through their promotion of microtubule assembly and inhibition of disaggregation.82 Both taxanes bind to the N-terminal 31–amino-acid sequence of the β-tubulin subunit of cellular tubulin polymers, stabilizing the polymers by shifting the dynamic equilibrium that exists between tubulin dimers and microtubules in favor of the polymerized state.83,84 Although there is preclinical evidence that docetaxel has both a higher affinity for the tubulin-binding site and greater in vitro cytotoxicity than paclitaxel, this has not necessarily translated into greater clinical efficacy because the toxicity profiles of the two drugs also differ.85,86
The administration of a taxane leads to cellular arrest in the G2/M phase of the cell cycle, which is the precise point associated with increased sensitivity to the lethal effects of ionizing radiation.87 Early laboratory studies with a human lung cancer cell line19 and human astrocytoma cells18 bore out the prospect of significant radiosensitization, with relative enhancement ratios in the 1.48 to 1.8 range when paclitaxel was administered before irradiation.
The exact conditions used in various studies appear to determine the strength of the interaction between radiation and paclitaxel because subadditive effects have been seen in addition to the more widely reported additive and supra-additive effects.21 In general, enhancement of radiation effects is seen when proliferating cells have been incubated with moderate concentrations of paclitaxel for 24 hours before irradiation. Conditions leading to a less-than-optimal response include paclitaxel-mediated G1 arrest, wherein a more resistant cell subpopulation counteracts the effects of a G2/M block; paclitaxel-induced cell cycle effects such as the G2/M block in cells destined to die before irradiation; and incubation conditions insufficient to exert cellular effects. The fact that nonproliferating cells are also sensitized to the effects of radiation by the use of paclitaxel suggests that mechanisms other than the cell cycle arrest in the G2/M phase underlie paclitaxel’s sensitizing abilities.
Paclitaxel also acts to induce programmed cell death; work from the M.D. Anderson Cancer Center21 has examined the relationship between mitotic arrest, apoptosis, and the antineoplastic activity of paclitaxel in 16 murine tumors. Single-dose paclitaxel (40 mg/kg) induced mitotic arrest to varying degrees in all tumors; however, apoptosis was induced in only 50% of tumors. This study also revealed that pretreatment levels of apoptosis correlated with both paclitaxel-induced apoptosis and tumor growth delay. Therefore, both the pretreatment apoptotic rate and paclitaxel-induced apoptotic rate could potentially act as predictors of the response to paclitaxel.
Milas et al.21 summarized observations that showed that (a) there was massive loss of tumor cells though the apoptotic pathway was restricted to the perivascular region, and (b) radio enhancement occurring during this period of cell loss became even more impressive when apoptotic cells were removed from the tumor. Experiments in which tumor xenografts were treated with paclitaxel and exposed to radiation under hypoxic or air-ambient conditions were pursued.20 It was found that the creation of hypoxic conditions greatly reduced the efficacy of paclitaxel in its enhancement of radioresponse. It appears that a combination of cell cycle effects, drug-induced reoxygenation, and drug-induced apoptosis underlies paclitaxel’s radiosensitizing abilities.
Docetaxel has also been found to be a respectable radiosensitizer in both in vitro52 and in vivo models.53 Radiation response in the presence of docetaxel was examined in three different cell lines that have widely different responses to radiation alone.88 Their findings suggest that the p53 status of tumor cells may have a profound effect on the radiosensitizing effects of a taxane. Other novel taxanes and analogs that continue to attract the interest of investigators for their potential to enhance radiation effects include Abraxane, paclitaxel poliglumex, larotaxel, cabazitaxel,89,90 and orally available taxanes.91
Epothilones
Epothilones are a novel class of antimicrotubule agents, originally derived from the myxobacterium Sorangium cellulosum. These agents bind to the site near the taxane binding site, and mechanism of action is similar to taxanes89,92,93; however, their chemical structures are unrelated, and their binding is specific and independent of the taxanes. Several epothilones are undergoing investigation in clinical trials: patupilone, ixabepilone, BMS-310705, ZK-EPO, and epothilone D.94 Epothilones enhance microtubule stability, formation of abnormal mitotic spindles inducing G2 and M arrest, and apoptosis. Interestingly, patupilone has been shown to cross the blood–brain barrier, and studies with concurrent radiation therapy for central nervous system (CNS) malignancies have been performed at the phase I level.95 The potential for patupilone to work as a radiosensitizer for multidrug-resistant cancer cells in vitro has been reported,96 as well as for medulloblastoma cells,97 lung cancer, and prostate cancer cells.98,99
Antimetabolites
5-Fluorouracil (5-FU)
The radiosensitizing properties of 5-FU have been known for years.100 Several mechanisms have been proposed for the cytotoxicity of this drug:
a. Its incorporation into RNA, which leads to a disruption of RNA function;
b. Inhibition of thymidylate synthetase function and subsequently of DNA synthesis; and
c. Direct incorporation of the drug into DNA.
It is believed that a combination of these effects underlies its radiosensitizing properties.101 Optimization of its schedule of delivery is crucial to obtaining an effect with this combination, and it is accepted that cytotoxic doses of 5-FU are needed to obtain a radiosensitizing effect. In general, it is thought that a continuous infusion of the drug is needed to obtain the desired drug levels after irradiation.102 Long-standing clinical experience with this drug bears out much of the laboratory studies of its effectiveness as a radiation sensitizer.
Capecitabine
Capecitabine is an oral prodrug of 5-FU. It is converted to its cytotoxic form in three enzymatic steps, the last of which is mediated by thymidine phosphorylase. One of the potential advantages of this mechanism for increasing tumor cytotoxicity is that thymidine phosphorylase is overexpressed in tumor tissues. Interestingly, radiation has been shown to stimulate expression of thymidine phosphorylase, which provides a further rationale for considering combined therapy with radiation treatments.103 Several phase I/II studies of radiation therapy and capecitabine primarily in rectal cancer have been completed,104,105 and a large phase III study from the National Surgical Adjuvant Breast and Bowel Project (NSABP) is under way.105
Gemcitabine
Gemcitabine is another nucleoside analog that acts as a very potent radiosensitizer. The biologic action of gemcitabine is due almost completely to its effects on DNA metabolism. Early studies of this drug in leukemic cell lines found that notable decreases in cellular deoxynucleotide triphosphates occurred with the use of the drug.106
Direct incorporation of the drug into DNA and drug-induced apoptosis are also thought to underlie its cytotoxicity.106 The metabolism of gemcitabine in the cell is complex, and it is able to potentiate its cytotoxicity as a sole therapy.106 Depending on the conditions, relative enhancement ratios in the range of 1.1 to 2.5 have been reported.
Gemcitabine is S-phase specific and as such should be selectively toxic to proliferating cells,107 decreasing the amount of proliferation that can occur during fractionated radiation therapy. In addition, cell cycle redistribution induced by these agents may improve cell kill by allowing more cells to be treated in the more sensitive parts of the cell cycle.108 As DNA synthesis inhibitors, these drugs may act to inhibit the repair of radiation-induced DNA damage.13 Finally, as DNA chain terminators, they may serve to trigger the apoptotic response.14
There is strong preclinical evidence to suggest that the radiosensitizing abilities of gemcitabine are intimately linked to cellular deoxyadenosine triphosphate levels.14 Interesting results from Latz et al.60 show quite clearly that cells that are pretreated with gemcitabine no longer show a progressive increase in radioresistance as they progress toward DNA replication, and sensitization, therefore, appears to be greatest in the S phase.
Milas et al.15 found the largest enhancement of growth delay when gemcitabine was delivered 24 to 60 hours before irradiation in a murine sarcoma tumor model. The use of gemcitabine with radiation also decreased the risk for development of lung metastases in those mice that attained durable local control (73% in the radiation-alone group vs. 40% in the combined-modality group), which was confirmed in a second study with a larger number of mice.61 This preclinical work is supportive of the principles of combined-modality therapy in that better local control translated into decreased systemic spread of tumor cells. The same authors also report a dose-dependent increase in the apoptotic rate after the administration of gemcitabine,15 which they believe correlates with the elimination of the more radioresistant S-phase population of cells and a redistribution of the remaining cells into more radiosensitive parts of the cell cycle. They also report that reoxygenation of the resistant hypoxic fraction of tumor cells is a mechanism for the radiosensitizing action of gemcitabine.61
In summary, the preclinical evidence suggests that gemcitabine acts through several mechanisms (nucleotide pool perturbation, lowering of the apoptotic threshold, cell cycle redistribution, and tumor cell reoxygenation) to enhance the effect of ionizing radiation on tumors. Clinical experience has shown that this drug is indeed a potent sensitizer with the potential for significant toxicity as well as improvement in tumor control when combined with radiation.109,110,111–115
Pemetrexed
Pemetrexed is a novel multitargeted agent, exerting its effect via simultaneous inhibition of multiple folate-requiring enzymes, including thymidylate synthase, dihydrofolate reductase, and glycinamide ribonucleotide formyl-transferase.116 Pemetrexed has shown synergistic activity with radiation treatments, likely due to interference with DNA synthesis.117 There are indications that radiosensitization by pemetrexed is not cell cycle phase specific118 in vitro. Others have suggested that a combination of radiation therapy and pemetrexed results in supra-additive effects, which may be in part due to apoptosis induction.119 Phase I/II studies in non–small cell lung cancer (NSCLC) and esophageal cancer combining pemetrexed-based chemotherapy with radiation therapy have been completed demonstrating tolerability and encouraging results.120,121,122,123–126
Topoisomerase I Inhibitors
Camptothecin is a plant alkaloid obtained from the Camptotheca acuminata tree. Its initial clinical evaluation in the 1960s and 1970s was abandoned because of severe and unpredictable hemorrhagic cystitis.127,128 Camptothecin and its derivatives (e.g., irinotecan, topotecan, 9-aminocamptothecin, SN-38) target DNA topoisomerase I.129–130,131 This enzyme relaxes both positively and negatively supercoiled DNA and allows for diverse essential cellular processes, including replication and transcription, to proceed. In the presence of camptothecin, a camptothecin–topoisomerase I–DNA complex becomes stabilized with the 5′-phosphoryl terminus of the enzyme-catalyzed DNA SSB bound covalently to a tyrosine residue of topoisomerase I. These stabilized cleavable complexes interact with the advancing replication fork during the S phase or during unscheduled DNA replication after genomic stress and cause the conversion of SSBs into irreversible DNA DSBs, resulting in cell death.130
Several investigators have reported that camptothecin enhances the cytotoxic effect of radiation in vitro and in vivo.54–55,56 Chen et al.55 showed that cells exposed to 20(S)-10,11 methylenedioxycamptothecin before or during radiation had sensitization ratios of 1.6, whereas those treated with the drug after radiation had substantially less enhancement of radiation-induced DNA damage. There are several hypotheses regarding the mechanism of interaction between radiation and irinotecan: (a) inhibition of topoisomerase I by irinotecan leads to inhibition of repair of radiation-induced DNA strand breaks; (b) irinotecan causes a redistribution of the cells into the more radiosensitive G2 phase of the cell cycle; (c) topoisomerase I–DNA adducts are trapped by irinotecan at the sites of radiation-induced SSBs, leading to their conversion into DSBs.57 While there is currently insufficient evidence to identify the underlying mechanism with certainty, the primary mechanism involved with radiosensitization may depend on which camptothecin derivative is being used.
Data from in vivo experiments demonstrate that combination 9-aminocamptothecin and irradiation is more effective when fractionated, compared with single doses.132 There is also evidence for circadian-dependent cytotoxicity and radiation sensitization when camptothecin derivatives like 9-aminocamptothecin are used as radiation sensitizers.132 The integration of this group of drugs into clinical treatments with radiation therapy have been explored in NSCLC and brain tumors.133–135,136
Alkylating Agents
Temozolomide
Temozolomide, a relatively new drug, is a second-generation alkylating agent, which is orally administered, is readily bioavailable, and demonstrates broad-spectrum activity in a variety of difficult-to-treat malignancies including glioma and melanoma.137 It is unique in its ability to cross the blood–brain barrier (about 30% to 40% of plasma concentration found in CSF). In vitro studies demonstrate increased inhibition of cell growth in combination with radiotherapy.138 Radiosensitization appears to occur via inhibition of DNA repair, leading to an increase in mitotic catastrophe.62 It has proven efficacy as a first-line therapy for glioblastoma multiforme (GBM) patients in conjunction with radiotherapy based on a randomized phase III clinical study demonstrating survival benefit.139 Temozolomide spontaneously converts to the reactive methylating agent MTIC and transfers methyl groups to DNA, the most important one being at the O6 position of guanine, an important site for DNA alkylation.140 The MGMT gene encodes a DNA repair protein that removes the alkyl group from the O6 position of guanine, and high MGMT activity levels abrogate the effectiveness of alkylating agents. In vitro, temozolomide enhances the radiation response most effectively in MGMT-negative glioblastomas, and likely due to decreased double-strand DNA repair capacity and increased DNA double-strand break damage, which occurs when the combination of temozolomide and radiation therapy was administered.63 Analysis of a randomized study by the European Organization for Research and Treatment of Cancer (EORTC) and National Cancer Institute of Canada (NCIC) demonstrated that loss of MGMT expression by promoter methylation is correlated with a better outcome after treatment with radiation and temozolomide.141
Other Agents
Mitomycin-C
Mitomycin-C is a quinone, whose mechanisms of action include inhibition of DNA and RNA synthesis.142 When combined with radiation, the rationale for the use of mitomycin-C is based on its ability to target hypoxic cells that are known to be relatively radiation resistant.143 There is preclinical evidence to suggest that mitomycin-C administered before irradiation leads to a supra-additive interaction.58,144 The postulated mechanisms of action for supra-additivity include prevention of repopulation and hypoxic cell sensitization, although the definitive action is not fully elucidated. Given that normal tissues are not hypoxic, the selective targeting of this cell population with mitomycin-C has the potential to improve cures without compromising normal-tissue complication rates. The use of mitomycin-C is limited by its hematologic toxicities. Mitomycin-C combined with radiation therapy remains the standard-of-care therapy for anal carcinoma based on multiple clinical studies.145
Tirapazamine
Investigators have pursued a similar strategy as outlined previously with the development of tirapazamine, a hypoxic cell cytotoxin.22,59 Brown59 has discovered that tirapazamine, which is a benzotriazine di-N-oxide, is toxic to hypoxic cells at concentrations much lower than what is needed to radiosensitize cells. It has the greatest differential toxicity known between hypoxic and well-oxygenated cells. Essentially, this drug functions through its intracellular reduction to form a highly reactive radical capable of causing both SSBs and DSBs.146 In the presence of oxygen, a free electron is absorbed, and the compound is back-oxidized to the parent compound with the concomitant release of a superoxide radical, which is much less cytotoxic than the tirapazamine radical. Clinical trials are ongoing or have been completed in multiple tumor sites including cervix, head and neck, glioblastoma multiforme, and lung malignancies (small cell lung cancer).147–159 A phase III study by the TransTasman group (TROG 02.02) suggested no significant improvement in survival outcome in locally advanced head and neck cancer patients, unselected for hypoxia, with the addition of tirapazamine to a standard platinum-based chemoradiation regimen.157 A subset analysis of the phase II study from the TransTasman Group suggested that tumors with a hypoxic component as assessed by 18F fluoromisonidazole positron emission tomography (FMISO-PET) imaging had a higher likelihood of locoregional failure, while an improvement in local control was identified for those treated with the addition of tirapazamine, suggesting that it specifically targets hypoxic tumor cells.153 While most studies have demonstrated a reasonable toxicity profile, several studies have suggested caution in the appropriate selection of a dose and delivery regimen when combining tirapazamine to a standard chemoradiation regimen.151,154
EMERGING STRATEGIES FOR IMPROVEMENT OF CHEMORADIATION THERAPY
In spite of increasing therapeutic achievements of chemoradiation therapy, the use of this form of therapy is still very much restricted by its narrow therapeutic index. The available agents are either insufficiently effective on their own or in combination with radiation against tumors, or normal-tissue toxicity prevents the use of effective doses of drugs or radiation. Significant research efforts, both preclinical and clinical, have been undertaken to improve chemoradiation therapy. They include developing more selective and more effective chemotherapeutic agents, incorporating additional agents into chemoradiation therapy that protect normal tissues from injury by drugs or radiation, and improving the technique of radiation therapy delivery to minimize treatment of adjacent normal tissue while maximizing tumor dose delivery.
Increasing Antitumor Efficacy of Chemotherapeutic Drugs
A number of newer chemotherapeutic agents are being developed with the goal of enhancing antitumor effects by improving on the selective targeting of tumor cells via strategies such as chemical modification of known compounds including agents currently in use with radiation therapy due to their radiosensitizing properties.
Approaches for improvement of drug safety, convenience, or efficacy could include chemical modifications, or modifications of the formulation of a known drug. One example is that of Abraxane (nab-paclitaxel, ABI-007). Abraxane is a 130-nM particle form of paclitaxel that is bound to albumin and is solvent free. This avoids the need for Cremophor-based vehicles and lowers the risk of hypersensitivity reactions.89 Such vehicles have the potential for altered pharmacokinetics by drug entrapment, leading to decreased drug clearance, decreased volume of distribution, and ultimately nonlinear pharmacokinetics. Interestingly, Abraxane has been demonstrated to have higher antitumor activity compared to Taxol in preclinical studies. This is in part mediated via utilization of albumin receptor–mediated endothelial transport, which leads to higher intracellular accumulation compared to standard paclitaxel.160 Clinically, Abraxane has shown demonstrated efficacy in metastatic breast cancer in a phase III study.161 Phase I/II studies in advanced NSCLC, melanoma, bladder cancer, high-risk prostate cancer, and gynecologic malignancy have been reported.161,162,163–172 Preclinical studies have demonstrated Abraxane to have radiosensitizing properties.173 Therefore, there is significant interest in determining the potential efficacy of Abraxane in disease sites where combined therapy with taxanes and radiation has demonstrated therapeutic advantage, and clinical trials are under way.
Another approach to make current chemotherapeutic drugs more effective against tumors and at the same time less toxic to normal tissues is compound modification via conjugation with water-soluble polymeric drugs, such as polyglutamic acid. These conjugates accumulate in tumors and release the active drug into the tumor in high concentrations and for a longer time. The enhancement in uptake by and prolongation of drug release in tumors are thought to be due to the enhanced permeability and retention effect of macromolecular compounds in solid tumors.174,175 The abnormal vasculature in tumors is porous to macromolecules, but high concentrations of drug can build up in tumors owing to inadequate lymphatic drainage, whereas polymer–drug conjugates are confined to the bloodstream in normal tissue.174 This leads to improved spatial localization of the cytotoxic drug within tumor. A highly promising polyglutamic acid–paclitaxel conjugate was recently developed. It is less toxic, more effective against tumors, and more enhancing of tumor radioresponse in preclinical studies than unconjugated paclitaxel.175,176 Furthermore, there are in vivo laboratory data suggesting that radiation adds to the accumulation of drug within tumors by modification of tumor vasculature.177 Therefore, multiple mechanisms for spatial localization and cooperation, including inherent drug properties, radiation modification of vasculature, and radiation-based targeting of sites within the body, could make this an attractive strategy for combining this agent with radiation treatments. Clinical trials in esophageal cancer (phase II) and gastric cancer (phase I) have been reported in combination with radiation treatments.178,179
Normal-Tissue Protection
Because normal-tissue toxicity represents a major limitation of concurrent chemoradiation therapy, every effort must be taken to prevent or minimize complications. This could be achieved through the incorporation into the treatment of radioprotective or chemoprotective agents or through improvements of radiation delivery. A number of chemical and biologic compounds are available that exhibited either selective or preferential protection of normal tissues in preclinical in vivo testing.180–183,184 In addition, there are many candidate compounds, particularly extracts from plants used in traditional medicine settings, that are undergoing in vitro evaluation.185–186,187 The most commonly tested radioprotectors are thiol compounds, such as WR-2721 (amifostine), a prodrug that must be converted in vivo to its active metabolite WR-1065. Amifostine is currently the only Food and Drug Administration (FDA)-approved radioprotector. The principal mechanisms of protection by these agents include scavenging of free radicals generated by ionizing radiation and some chemotherapy agents, such as alkylating agents, and donating hydrogen atoms to facilitate direct chemical repair of DNA damage. However, amifostine modulates transcriptional regulation of genes involved in apoptosis, cell cycle regulation, and DNA repair as well.188 The protector is taken up preferentially by normal tissues, where the entry into cells is accomplished by active transport. In contrast, the drug diffuses passively into tumors, where its availability is also reduced by deficient tumor vasculature. Amifostine has been shown to reduce normal-tissue toxicity in a number of clinical settings, including protection of salivary glands in head and neck radiation therapy,189,190 prevention of acute and late normal-tissue toxicities from chemoradiation in cancers of the head and neck191 and the esophagus in chemoradiation therapy of lung cancer,192,193 without adversely affecting tumor response to treatment. The drug significantly protects against cisplatin-induced nephrotoxicity, ototoxicity, and neuropathy.
Emerging Strategies to Improve Radiation Therapy
Technology-Based Strategies
Several technologic strategies to improve the efficacy and decrease the toxicity of radiation therapy have been developed. These advances include improvements in radiation therapy delivery, such as three-dimensional treatment planning, conformational radiation therapy, intensity-modulated radiation therapy (IMRT), and image-guided radiation therapy (IGRT). Use of heavy particles, such as protons or carbons, which have a more favorable beam profile, is another approach likely to minimize the toxicity, and combining such treatments with chemotherapy may lead to enhancement of the effectiveness of not only radiation therapy but also chemoradiation treatments. The principle primarily exploited with this would be that of maximizing spatial localization, with technologic advances and/or heavy particles being used to maximize radiation therapy’s spatial localization. Further advances in imaging, with the advent of molecular-based imaging applications in oncology, will lead to further refinement of IGRT techniques. Because there are significant discussions of three-dimensional conformational radiation therapy (3DCRT), IMRT, IGRT, and heavy particles detailed in other chapters, we refer readers to those chapters for more in-depth discussion.
Altering Fractionation Schemes to Improve Therapeutic Ratio for Chemoradiation Treatments
Accelerated fractionation regimens are designed to counteract tumor repopulation, and accelerated fractionation with concomitant boost technique has shown superiority in terms of local control over the standard fractionation regimen in a large randomized study for head and neck cancer patients.194 Head and neck cancer remains one of the best paradigms for effectiveness of accelerated fractionation methods in clinical use. However, when combined with chemotherapy, a benefit to accelerated fractionation over standard fractionation was not demonstrated.195
Hyperfractionation is another strategy aimed at increasing the tumoricidal dose by delivering smaller doses/fractions, which allows a higher total dose to be administered as smaller doses/fractions lead to improved tolerance of late-responding normal tissues.87 The efficacy of hyperfractionation over standard fractionation has been demonstrated in several disease sites, including lung and head and neck cancer.194,196,197 In head and neck cancer, hyperfractionated radiation therapy with chemotherapy has yielded superior outcomes compared to hyperfractionated radiation therapy (RT) alone198; however, there has been no trial to demonstrate superiority of hyperfractionated RT and chemotherapy compared to conventional RT and chemotherapy. Perhaps, given the limited success of concurrent therapy, methods of combining systemic agents sequentially either before or after radiation treatments with altered fractionation can be considered.
MOLECULAR-TARGETED THERAPIES: NEW AGENTS AND NOVEL PARADIGMS FOR OPTIMIZING COMBINED MODALITY THERAPY
Recent discoveries in molecular biology have identified a number of molecular pathways involving receptors, enzymes, or growth factors that may be responsible for resistance of cancer cells to radiation or other cytotoxic agents and as such may serve as targets for augmentation of radiation response or chemotherapy response. It is becoming more evident that with advances in the understanding of the molecular processes that are associated with various malignancies, a molecular profile of a tumor may prove to be as important as its pathologic profile. Further classification of a patient’s tumor molecular profile should, among other things, aid in selection of the appropriate targeted agents. The challenge for radiation oncologists in this molecular era is determining what would be the best method of integrating cytotoxic radiation treatments with a molecular-based therapeutic plan.
Among this expanding list of molecular targets are epidermal growth factor (EGF) and its receptor (EGFR); vascular endothelial growth factor (VEGF) and its receptor (VEGFR); mammalian target of rapamycin (mTOR); anaplastic lymphoma kinase (ALK) fusion proteins; heat shock protein 90 (hsp90); poly(adenosine diphosphate [ADP]-ribose) polymerase (PARP); mutated ras; histone deacetylase (HDAC) inhibitors; cell cycle checkpoint control proteins such as checkpoint kinase 1 (CHK1); a number of the DNA repair enzymes, including DNA-dependent protein kinase (DNA-PK), ataxia telangiectasia mutated (ATM), and RAD51; the proteosome; angiogenic molecules; and various other molecules that regulate different steps in their signal transduction pathways.199 A handful of agents have now gained FDA approval for cancer therapy in patients, and many are undergoing clinical trials to determine their efficacy when used in combination with radiation therapy. Some agents are potentially single-pathway targets, and others are able to target multiple molecular signaling pathways. Some newly emerging molecular strategies to improve chemoradiation therapy are shown in Table 32.3. The most clinically advanced of these strategies include agents targeting EGFR, VEGF and VEGFR, and ALK1 pathways. The scope of this section will be a discussion of relevant molecularly targeted agents that have been FDA approved for use, and which hold promise for use in conjunction with radiation treatments.
TABLE 32.3 MOLECULAR TARGETING POSSIBILITIES IN COMBINATION WITH CHEMORADIATION OR RADIATION
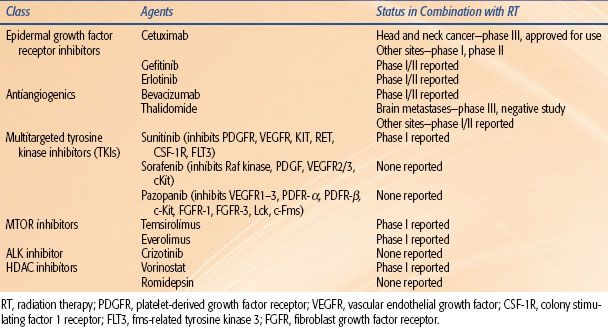
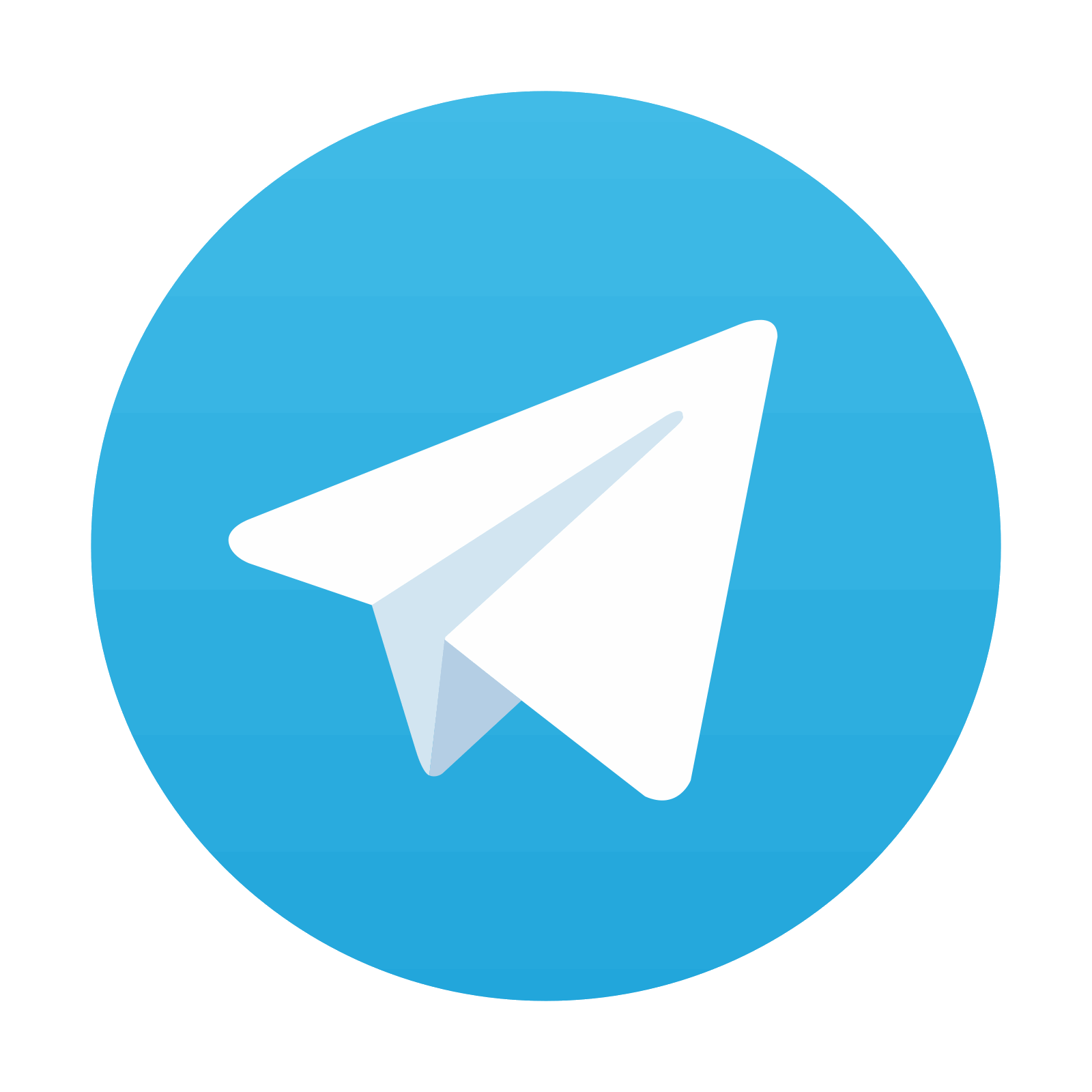