Fig. 1
Structure of a spherical nucleic acid (SNA)—gold nanoparticle conjugate. (Reproduced with permission from [8])
2.1 Synthesis of SNAs
We will first describe how these constructs are synthesized. SNAs can be prepared with inorganic cores (silver [14], gold [15], iron oxide [16], quantum dots [17], silica [18], infinite coordination polymers [19]) or be coreless (cross-linked alkyne polymers [20], liposomes [21]) in nature. However, SNAs with gold particle cores are most commonly used in gene detection assays involving nanoflares because gold is inert and has the ability to quench fluorescent molecules in a distance-dependent manner [9]. Citrate-capped gold colloids can be synthesized using the Frens method, where chloroauric acid (HAuCl4) is reduced by sodium citrate [22]. This well-established, solution-based method allows for the facile synthesis of highly uniform gold colloids of a specific size ranging from 5 to 150 nm in diameter [22]. The particle acts as a template for the subsequent attachment of nucleic acids and thus plays an important role in determining the final size of the overall nanostructure. Indeed, a core size must be chosen such that the SNA will be able to enter cells and function appropriately once inside. To date, the most commonly used SNAs for diagnostic applications have typically consisted of a 10–15 nm gold cores [9] with thiol [23] or cyclic disulfide [24] attachments.
The oligonucleotides that make up the nucleic acid shell are typically 25–40 bases in length (7–12 nm) and can be composed of single- and double-stranded DNA [10], short-interfering RNA (siRNA) [25], micro-RNA (miRNA) [26], RNA/DNA hybrids [27], and modified nucleic acids such as peptide nucleic acids (PNA) [28] and locked nucleic acids (LNA) [29]. Each oligonucleotide anchored to the core is made up of three distinct regions: (1) an alkylthiol [23] or cyclic disulfide [24] chemical tethering group that can be used to link the oligonucleotides to the gold nanoparticle’s surface, (2) a recognition element (usually 15–25 base pairs in length) that is complementary to the target biomolecule of interest, and (3) a spacer region that can be modulated in length to tune the distance between the recognition element and the nanoparticle surface. Because of the ability of nucleobases to interact with the gold surface, it is necessary to extend the recognition element away from the particle surface, giving it more free volume to interact with incoming strands due to the curvature of the nanoparticle [30]. Poly-adenine (poly-A) or oligo-ethylene glycol (OEG) are commonly utilized as spacers [31]. Poly-thymine (poly-T) spacers are usually avoided for intracellular applications because of their ability to interact with the poly-A tails of a variety of mRNA, diminishing the sequence specificity of the SNAs.
To create a dense nucleic acid shell, the alkylthiol modified oligonucleotides are first mixed in solution with the citrate-capped gold particles (Fig. 2). Thiol moieties have a high affinity for gold; thus, the thiolated oligonucleotides displace the citrate ions and adsorb onto the gold surface. To facilitate the formation of a dense monolayer of oligonucleotides, monovalent counterions (such as sodium ions) need to be slowly added into the mixture to screen the negative charges of the phosphate backbones. One can control the number of strands that adsorb to the gold surface (from 50 to 250 oligonucleotides per 15 nm particle) by varying the amount of added sodium ions from 0.05 to 1.0 M and the spacer type to achieve a dense multivalent nanostructure [31]. We will discuss the importance of tuning the oligonucleotide density in the context of cellular entry as well as endogenous nucleic acid binding in later sections. The three-dimensional architecture of the nucleic acids around the gold core confers some unique and biologically attractive properties, which make SNAs desirable for high sensitivity intracellular detection assays. These properties will be described in the next section.
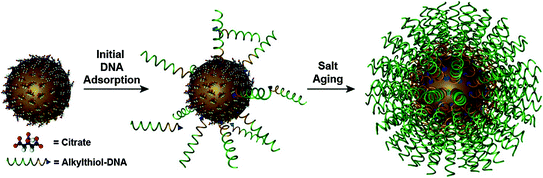
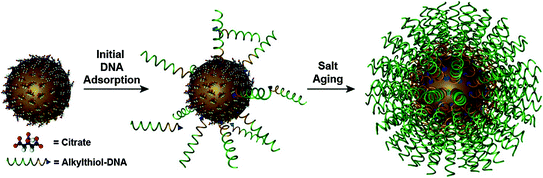
Fig. 2
Synthesis of SNA conjugates. Citrate-stabilized particles are incubated with alkylthiol-functionalized oligonucleotides in water to form a low-density monolayer. By incubating the nanoparticles in aqueous solutions with successively higher concentrations of salt (typically, 0.15–1.0 M), a high-density spherical nucleic acid shell is formed. (Reproduced and modified with permission from [8])
2.2 Properties that Make SNAs Ideal as Intracellular Diagnostic Probes
Unlike linear nucleic acids, SNAs have been shown to be capable of rapidly entering many cell lines without complexation to carrier moieties, despite their dense polyanionic shell (Fig. 3) [32, 9, 33]. Generally, negatively charged nucleic acids require cationic moieties, such as lipoplexes, peptides, or viruses, to traverse through the negatively charged cellular membrane. These transfection agents can be harmful to cells and result in off-target effects, which may alter the expression of a variety of genes in an uncontrollable manner [34]. In many detection assays, such perturbations are significant enough to result in false-positive signals [34]. One does not encounter this issue when using SNAs, as they do not require additional carriers. The dense, highly oriented array of nucleic acids on the surface of SNAs are recognized by class A scavenger receptors (SR-A), allowing for their uptake into cells in high quantities (on the order of 106/cell) as single entities via lipid raft-dependent, caveolae-mediated endocytosis [32]. We confirmed that this was the primary mechanism of SNA uptake by demonstrating that uptake was reduced by over 90 % when the SR-A levels of cells that normally took up SNAs in high quantities were depleted via conventional viral vector-based siRNA knockdown [32]. Further, our group found that the cellular uptake of SNAs scales with the density of oligonucleotides adsorbed to the nanoparticle core; a higher number of oligonucleotides loaded on the particle typically increase SNA entry into cells [35].
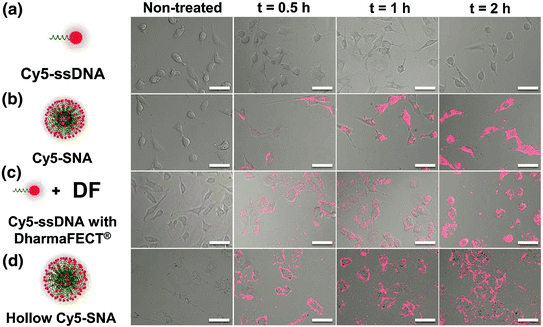
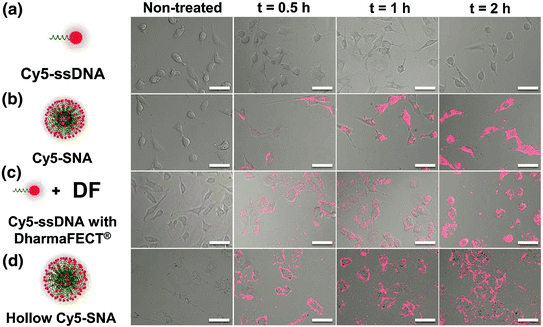
Fig. 3
SNA uptake into cells. The three-dimensional architecture of the SNA allows it to be rapidly endocytosed via scavenger receptor-mediated endocytosis. Linear nucleic acids of the same sequence are unable to enter cells without the use of transfection agents. (Reproduced and modified with permission from [32]
Upon entering cells, SNAs resist enzymatic degradation by nucleases [10] and treated cells exhibit no apparent toxicity [9] or off-target effects [12]. Each of these properties is necessary for the development of a nucleic acid detection platform that can be used in live cells. Nature has engineered intracellular machinery, such as nucleases to recognize and degrade exogenous DNA and RNA, rendering them unable to perform their intended function to protect cells from foreign organisms. Unlike free oligonucleotides, the SNA’s dense nucleic acid shell sterically resists the binding of nucleases to individual oligonucleotides [10]. We have shown that the salt cloud associated with SNAs that screen the negative charges of neighboring strands also inhibits enzyme activity [36]. Cellular cytotoxicity is not observed after 72 h of treatment with SNAs [20], and the whole-genome expression profiles of cells treated with SNAs did not show significant up- or down-regulation of genes compared to untreated cells [37]. However, elevated cytotoxicity was observed with linear nucleic acids of an identical sequence that were transfected into cells at therapeutically necessary doses using a cationic lipoplex (DharmaFECT 1); changes in 427 unintended genes were also observed (Fig. 4).
Once inside cells, an ideal intracellular probe for early stage cancer diagnosis must also be able to detect gene expression at low levels. SNAs have significantly higher binding constants for free complementary oligonucleotide strands than free DNA of the same sequence (over 100 times higher) [13], indicating that these structures are able to be utilized for sensitive diagnostic assays (Nanosphere, Inc., Northbrook, IL). This enhanced binding strength manifests itself as an increase in the melting temperature, T m , the temperature at which half of the complementary strands in solution are dehybridized, as well as a decrease in the full width at half maximum (FWHM) of the melting transition (Fig. 5). In one proposed mechanism, the enhanced binding strength was found to be directly related to the high density of DNA strands on and high local salt concentration around the nanoparticle surface rather than the absolute amount of bound DNA [38]. Further, the high oligonucleotide density on the SNA restricts the configurational microstates that can be explored by individual strands and increases the binding constant of complementary nucleic acids even more [38]. The increase in binding strength exhibited by the SNAs in comparison to the free molecular probes directly translates into lower limits of detection for target sequences, and it allows for the differentiation of fully complementary and mismatched DNA targets that contain a single-nucleotide polymorphism [39].
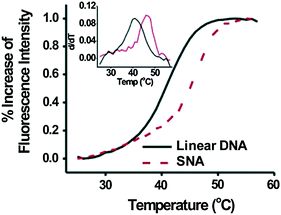
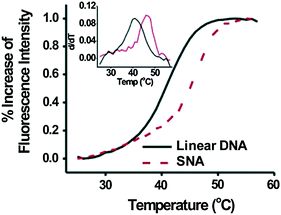
Fig. 5
Complementary strands in solution have a higher binding constant when bound to SNAs versus free linear nucleic acids of the same sequence. (Reproduced with permission from [13])
3 The Nanoflare: A Platform for Gene Detection and Regulation in Live Cells
The unique characteristics of the SNA have allowed this platform to thrive in numerous applications in biology and medicine. As a therapeutic agent, the nanoconjugate can be delivered intravenously [40] or as a topical agent [37] without toxicity or immunogenicity, enter most cells tested to date efficiently and in high quantities [35], and regulate the expression of targeted genes; they have even been found to cross the blood–brain and blood–tumor barriers [40]. Even so, the SNA platform has shown the most immediate success as a gene detection and diagnostic agent in an architecture known as the nanoflare. The nanoflare is an SNA-gold nanoparticle conjugate functionalized with ssDNA or DNA/LNA hybrid sequences, which are designed to be complementary to genes of interest (Fig. 6). These strands are referred to as the recognition or antisense strands. A short internal complementary strand containing a terminal fluorophore is hybridized onto the antisense strand. When bound in close proximity to the gold particle, the fluorescence of the fluorophore is quenched. However, in the presence of an mRNA target complementary to the antisense sequence, the target forms a longer, more stable duplex and displaces the shorter flare strand. Displacement of the fluorophore from the nanoparticle surface results in a “turn on” of a fluorescence signal proportional to the concentration of the target transcripts. It should be noted that at higher concentrations, nanoflares have also been shown to bind to target mRNA and inhibit translation of the corresponding protein [11]. The unique architecture of the SNA allows the nanoflare to enter live cells and detect mRNA expression without the significant disruption of cellular homeostasis, a result difficult to achieve with other fluorescence-based RNA detection assays, such as those based on fluorescent in situ hybridization (FISH) [41] or molecular beacons [42]. Furthermore, the increased binding thermodynamics and selectivity of complement hybridization exhibited by the SNA enable the nanoflare to attain extremely sensitive and specific detection of polynucleotide targets [43]; so much so that in vitro the nanoflare has been able to distinguish complementary targets from those with single nucleotide polymorphisms (SNPs) [11].
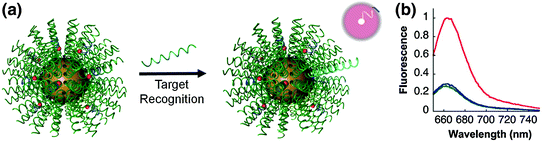
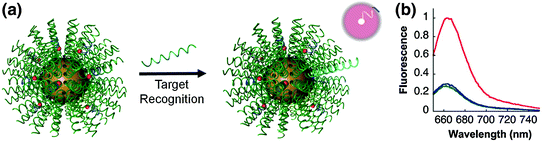
Fig. 6
a Schematic representation of sequence-specific recognition of target DNA using nanoflares. b Fluorescence response of nanoflares alone (green), and nanoflares upon recognition of target DNA (red) compared to noncomplementary DNA (blue). (Reproduced and modified with permission from [7])
4 Detection of Intracellular Oncogenes
In 2007, the nanoflare was first introduced as a promising platform for mRNA detection in living cells. In a proof-of-concept experiment, nanoflares were utilized to detect intracellular mRNA targets with single-cell resolution and without perturbing cell function. Such results cannot be attained with conventional mRNA quantification techniques, such as real-time polymerase chain reaction (RT-PCR) [44], and the nanoflare enables the profiling of cells based on their genetic content, while preserving their viability for further analysis (vide infra). Since the completion of this work, nanoflares have been commercialized by EMD Millipore and sold under the trade name SmartFlare™, with over 1700 genetically unique versions of these constructs currently available in over 230 countries [45].
4.1 Fluorescence Response to Target Oligonucleotides in Extracellular Conditions
To investigate the ability of nanoflares to detect oncogenes in a breast cancer cell model, a type of nanoflare-targeting survivin, an anti-apoptotic gene that is upregulated in a range of cancer types, was designed [7]. Prior to their use in cells, nanoflares were tested in extracellular conditions with synthetic DNA targets to confirm the sequence specificity of the release of the fluorophore-labeled DNA flare strands upon target recognition and binding. Specifically, upon incubation with target DNA, the Cy5 fluorescence of survivin nanoflares was enhanced 3.8-fold. In contrast, the fluorescence signal did not change in the presence of a noncomplementary DNA strand (Fig. 6). Taken together, these results demonstrate that nanoflares efficiently signal the presence of target oligonucleotide in a sequence-specific manner. This capability is vital to the use of nanoflares as intracellular mRNA detection probes.
4.2 Uptake and Fluorescence Response in Cell Culture
Based on their ability to enter cells without additional transfection reagents, nanoflares can be used in cell culture models. This was first demonstrated in SKBR3 cells, a human breast cancer model that expresses high quantities of the survivin mRNA transcript [46]. As a control, noncomplementary nanoflares that did not recognize the survivin transcript were designed to have similar background fluorescence, melting properties, and signaling ability as the targeting probe. Cultured SKBR3 cells were treated with either survivin-targeting or noncomplementary nanoflares, and imaged by confocal microscopy (Fig. 7). A control cell line, C166 mouse endothelial cells [7], that does not express human survivin, was also treated with nanoflares and imaged. The fluorescence of SKBR3 cells treated with survivin nanoflares was significantly higher than those treated with noncomplementary nanoflares. In addition, there was no distinguishable difference in the fluorescence of C166 cells treated with either survivin or noncomplementary nanoflares. These results demonstrate the use of nanoflares to qualitatively profile cells for the expression of an important oncogene, survivin.
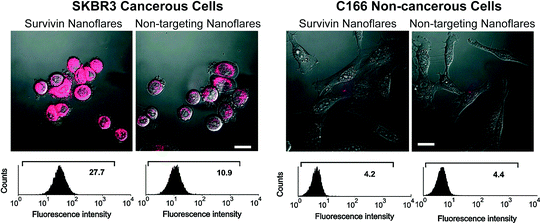
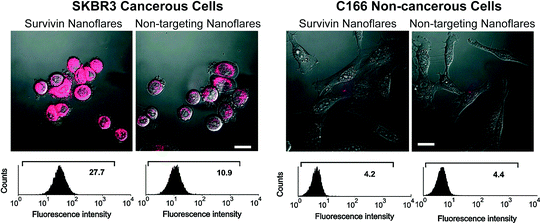
Fig. 7
Fluorescence microscopy (top) and flow cytometry data (bottom) for SKBR3 (breast cancer, left two) and C166 (healthy mouse endothelial, right two) cells following treatment with survivin or noncomplementary nanoflares. (Reproduced and modified with permission from [7])
In order to quantify the intracellular fluorescence signal from nanoflares, analytical flow cytometry was used. In addition to signal quantification, flow cytometry allows for the collection of data from a larger population of cells than practically attainable using microscopy. Cells that were treated with nanoflares were observed as a uniform population of fluorescent cells, consistent with the high cell penetration (>99 %) that is observed with SNAs (Fig. 7) [10]. The SKBR3 cells treated with survivin-targeting nanoflares were 2.5 times more fluorescent than those treated with noncomplementary nanoflares. In addition, as seen in the microscopy analysis, C166 cells treated with either survivin-targeting or noncomplementary nanoflares displayed low fluorescence. These results demonstrate the nanoflare’s capability of distinguishing cancerous cell populations based on the expression of an mRNA target of interest.
5 Concomitant Detection of Multiple Targets: Multiplexed Nanoflares
Cancers are highly heterogeneous and intricate, and in such disease states, multiple genes can be up- and/or down-regulated concurrently. The original nanoflare architecture only allowed for the detection of a single mRNA’s expression level. To target more than one gene, multiple, individual nanoflares would need to be designed and synthesized, significantly increasing the complexity of the system. Thus, to make the nanoflare more relevant as a diagnostic probe for diseases like cancer, its architecture was modified such that a single probe could be used to detect multiple mRNA expression profiles simultaneously. This modified architecture has been termed the multiplexed nanoflare. While our group pioneered the idea of the multiplexed nanoflare by showing that a single probe could be used to identify two independent mRNA expression profiles [47], other groups have since shown that three and four transcripts can also be detected simultaneously [48, 49].
While the original nanoflares were made by anchoring a single type of recognition strand to the gold core, the multiplexed nanoflares are synthesized by functionalizing multiple antisense sequences onto the same particle. As a proof-of-concept, our group developed multiplexed nanoflares that can recognize actin and survivin mRNA sequences (Fig. 8). Specifically, equimolar concentrations of actin and survivin recognition sequences were functionalized onto the particles and then hybridized with flare strands bearing Cy3 and Cy5 fluorophores, respectively. Therefore, in the presence of survivin mRNA, the probes are designed to release the Cy3 flares, and in the presence of actin, the probes are designed to release the Cy5 flares. To test the specificity of flare release, actin and survivin target DNA were incubated with the multiplexed nanoflares for 1 h at 37 °C, and Cy3 and Cy5 emission fluorescence spectra were collected. The Cy5 channel showed an increase in fluorescence only in the presence of actin target DNA, and the Cy3 channel showed an increase only in the presence of the survivin target, confirming that the flares are released in a sequence-specific manner (Fig. 8).
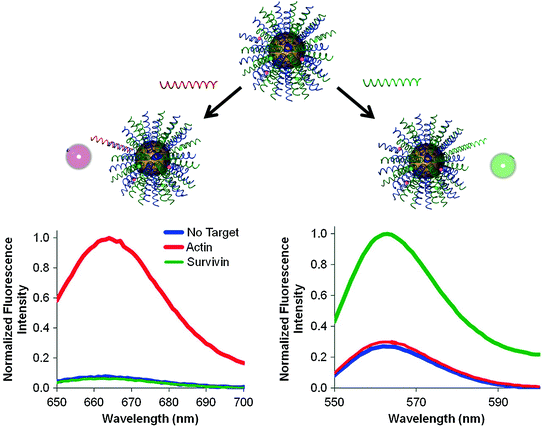
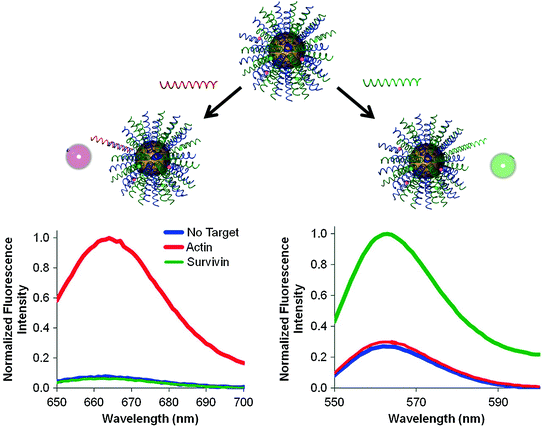
Fig. 8
The addition of DNA targets complementary to each flare (green and red) to multiplexed nanoflares, individually, caused the increase in fluorescence intensity of each flare on the Cy5 and Cy3 channels, respectively. (Reproduced and modified with permission from [47])
5.1 Confocal Measurement of Survivin and Actin Gene Expression in Live Cells
To determine if these constructs could distinguish the cellular expression levels of actin and survivin simultaneously, multiplexed nanoflares were added to HeLa cells, a cell line known to contain high levels of both actin and survivin, that were pretreated with either actin siRNA or survivin siRNA (Fig. 9). This way, one set of cells would have a high level of survivin mRNA present and low level of actin mRNA, while the other set of cells would have the opposite ratio. A decrease in Cy3 fluorescence (Fig. 9 red, left panel) was seen when the survivin expression was knocked down using survivin-targeted siRNA as measured by flow cytometry and confocal microscopy; the fluorescence in the Cy5 actin (Fig. 9 green, left panel) channel remained constant. A decrease in Cy5 fluorescence intensity (Fig. 9 green, right panel) was observed when the cells were treated with actin-targeted siRNA and multiplexed nanoflares; a significant change was not seen in the Cy3 survivin (Fig. 9 red, right panel) channel. These data demonstrated that the multiplexed nanoflares were able to enter live cells and release two different types of flares with a high level of specificity.
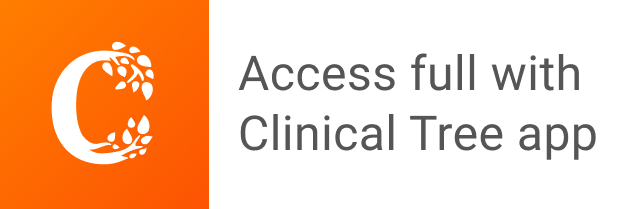