Figure 2.2 The amplification and maturation sequence in the development of mature red cells from the pronormoblast
Figure 2.3 Comparison of the DNA and RNA content, and marrow and peripheral blood distribution, of the erythroblast (normoblast), reticulocyte and mature red blood cell (RBC).
Erythropoiesis is regulated by the hormone erythropoietin. Erythropoietin is a heavily glycosylated polypeptide of 165 amino acids with a molecular weight of 34 kDa. Normally, 90% of the hormone is produced in the peritubular interstitial cells of the kidney and 10% in the liver and elsewhere. There are no preformed stores and the stimulus to erythropoietin production is the oxygen (O2) tension in the tissues of the kidney (Fig. 2.4). Hypoxia induces hypoxia-inducible factors (HIF-2α and β) which stimulate erythropoietin production. The erythropoietin gene contains a Hif response element at its 3 ′ end. Erythropoietin production therefore increases in anaemia, when haemoglobin for some metabolic or structural reason is unable to give up O2 normally, when atmospheric O2 is low or when defective cardiac or pulmonary function or damage to the renal circulation affects O2 delivery to the kidney.
Figure 2.4 The production of erythropoietin by the kidney in response to its oxygen (O2) supply. Erythropoietin stimulates erythropoiesis and so increases O2 delivery. BFUE, erythroid burst-forming unit; CFUE, erythroid colony-forming unit. Hypoxia induces hypoxia inducible factors (HIFs) α and β, which stimulate erythropoietin production. Von-Hippel–Lindau (VHL) protein breaks down HIFs. PHD2 (prolyl hydroxylase) hydroxylates HIF-2 α allowing VHL binding to HIFs. Mutations in VHL, PHD2 or HIF-2 α underlie congenital polycythaemia (see p. 208).
Erythropoietin stimulates erythropoiesis by increasing the number of progenitor cells committed to erythropoiesis. The transcription factors GATA-1 and FOG-1 are activated by erythropoietin receptor stimulation and are important in enhancing expression of erythroid-specific genes (e.g. haem biosynthetic and red cell membrane proteins) and also enhancing expression of anti-apoptotic genes and of the transferrin receptor (CD71). Late BFUE and CFUE, which have erythropoietin receptors, are stimulated to proliferate, differentiate and produce haemoglobin. The proportion of erythroid cells in the marrow increases and, in the chronic state, there is anatomical expansion of erythropoiesis into fatty marrow and sometimes into extramedullary sites. In infants, the marrow cavity may expand into cortical bone result ing in bone deformities with frontal bossing and protrusion of the maxilla (see p. 95).
Conversely, increased O2 supply to the tissues (because of an increased red cell mass or because haemoglobin is able to release its O2 more readily than normal) reduces the erythropoietin drive. Tissue hypoxia also stimulates new blood vessel formation by vascular endothelial growth factor (VEGF) and reduces transferrin but lowers hepcidin synthesis (see p. 38).
Plasma erythropoietin levels can be valuable in clinical diagnosis. They are high if a tumour-secreting erythropoietin is causing polycythaemia but low in severe renal disease or polycythaemia vera (Fig. 2.5).
Figure 2.5 The relation between erythropoietin (EPO) in plasma and haemoglobin concentration. Anaemias exclude conditions shown to be associated with impaired production of EPO. (From M. Pippard et al. (1992) B J Haematol 82 : 445, with permission.)
Indications for erythropoietin therapy
Recombinant erythropoietin is of great value in treating anaemia resulting from renal disease or from various other causes. It is given subcutaneously either 3 times weekly or once every 1–2 weeks or every 4 weeks depending on the indication and on the preparation used (erythropoietin alpha or beta, darbepoetin alpha, a heavily glycosylated longer acting form, or Micera the longest acting preparation). The main indication is end-stage renal disease (with or without dialysis). Other uses are listed in Table 2.1 In these conditions, higher doses are often needed. The haemoglobin level and quality of life may be improved (see Chapter 12). A low serum erythropoietin level prior to treatment is valuable in predicting an effective response. Oral or parenteral iron is often needed to maximize the response to erythropoietin therapy. Side-effects include a rise in blood pressure, thrombosis and local injection site reactions. It has been associated with progression of some tumours.
Table 2.1 Clinical uses of erythropoietin.
Anaemia of chronic renal disease |
Myelodysplastic syndrome |
Anaemia associated with malignancy and chemotherapy |
Anaemia of chronic diseases, e.g. rheumatoid arthritis |
Anaemia of prematurity |
Perioperative uses |
The marrow requires many other precursors for effective erythropoiesis. These include metals such as iron or cobalt, vitamins (especially vitamin B12, folate, vitamin C, vitamin E, vitamin B6, thiamine and riboflavin) and hormones such as androgens and thyroxine. Deficiency in any of these may be associated with anaemia.
Haemoglobin synthesis
The main function of red cells is to carry O2 to the tissues and to return carbon dioxide (CO2) from the tissues to the lungs. In order to achieve this gaseous exchange they contain the specialized protein haemoglobin. Eachred cell contains approximately 640 million haemoglobin molecules. Each molecule of normal adult haemoglobin A (Hb A) (the dominant haemoglobin in blood after the age of 3–6 months) consists of four polypeptide chains, α2 β2, each with its own haem group. The molecular weight of Hb A is 68 000. Normal adult blood also contains small quantities of two other haemoglobins: Hb F and Hb A2. These also contain α chains, but with γ and δ chains, respectively, instead of β (Table 2.2). The synthesis of the various globin chains in the fetus and adult is discussed in more detail in Chapter 7.The major switch from fetal to adult haemoglobin occurs 3–6 months after birth (Table 2.2; see Fig. 7.1b).
Table 2.2 Normal haemoglobins in adult blood.
Haem synthesis occurs largely in the mitochondria by a series of biochemical reactions commencing with the condensation of glycine and succinyl coenzyme A under the action of the key rate limiting enzyme δ-aminolaevulinic acid (ALA) synthase (Fig. 2.6). Pyridoxal phosphate (vitamin B6) is a coenzyme for this reaction which is stimulated by erythropoietin. Ultimately, protoporphyrin combines with iron in the ferrous (Fe2+) state to form haem (Fig. 2.7), each molecule of which combines with a globin chain made on the polyribosomes (Fig. 2.6). A tetramer of four globin chains each with its own haem group in a ‘pocket’ is then formed to make up a haemoglobin molecule (Fig. 2.8).
Figure 2.6 Haemoglobin synthesis in the developing red cell. The mitochondria are the main sites of protoporphyrin synthesis, iron (Fe) is supplied from circulating transferrin; globin chains are synthesized on ribosomes. δ-ALA, δ-aminolaevulinic acid; CoA, coenzyme A.
Figure 2.7 The structure of haem.
Figure 2.8 The oxygenated and deoxygenated haemoglobin molecule. α, β, globin chains of normal adult haemoglobin (Hb A). 2,3-DPG, 2,3-diphosphoglycerate.
Haemoglobin function
The red cells in systemic arterial blood carry O2 from the lungs to the tissues and return in venous blood with CO2 to the lungs. As the haemoglobin molecule loads and unloads O2 the individual globin chains in the haemoglobin molecule move on each other (Fig. 2.8). The α1β1 and α2β2 contacts stabilize the molecule. The β chains slide on the α1β2 and α2β1 contacts during oxygenation and deoxygenation. When O2 is unloaded the β chains are pulled apart, permitting entry of the metabolite 2,3-diphosphoglycerate (2,3-DPG) resulting in a lower affinity of the molecule for O2. This movement is responsible for the sigmoid form of the haemoglobin O2 dissociation curve (Fig. 2.9). The P50 (i.e. the partial pressure of O2 at which haemoglobin is half saturated with O2) of normal blood is 26.6 mmHg. With increased affinity for O2, the curve shifts to the left (i.e. the P 50 falls) while with decreased affinity for O2, the curve shifts to the right (i.e. the P50 rises).
Figure 2.9 The haemoglobin oxygen (O2) dissociation curve. 2,3-DPG, 2,3-diphosphoglycerate.
Normally, in vivo, O2 exchange operates between 95% saturation (arterial blood) with a mean arterial O2 tension of 95 mmHg and 70% saturation (venous blood) with a mean venous O2 tension of 40 mmHg (Fig. 2.9).
The normal position of the curve depends on the concentration of 2,3-DPG, H+ ions and CO2 in the red cell and on the structure of the haemoglobin molecule. High concentrations of 2,3-DPG, H+ or CO2, and the presence of certain haemoglobins, e.g. sickle haemoglobin (Hb S), shift the curve to the right (oxygen is given up more easily) whereas fetal haemoglobin (Hb F)–which is unable to bind 2,3-DPG–and certain rare abnormal haemoglobins associated with polycythaemia shift the curve to the left because they give up O2 less readily than normal.
Methaemoglobinaemia
This is a clinical state in which circulating haemoglobin is present with iron in the oxidized (Fe3+) instead of the usual Fe2+ state. It may arise because of a hereditary deficiency of methaemoglobin reductase deficiency or inheritance of a structurally abnormal haemoglobin (Hb M). Hb Ms contain an amino acid substitution affecting the haem pocket of the globin chain. Toxic methaemoglobinaemia (and/or sulphaemoglobinaemia) occurs when a drug or other toxic substance oxidizes haemoglobin. In all these states, the patient is likely to show cyanosis.
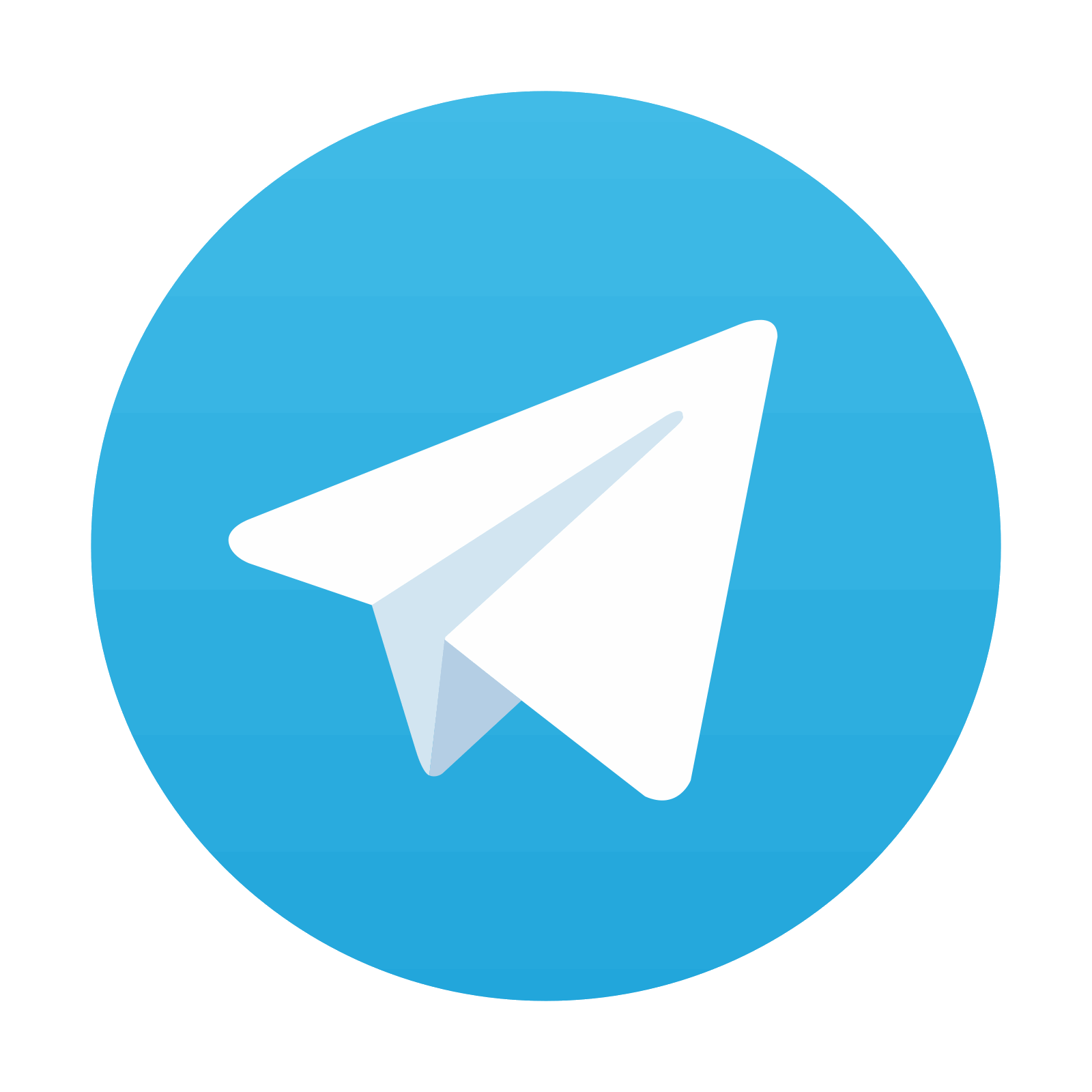
Stay updated, free articles. Join our Telegram channel
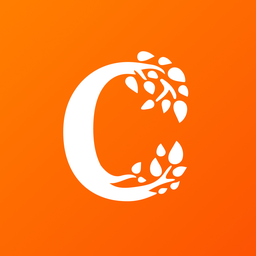
Full access? Get Clinical Tree
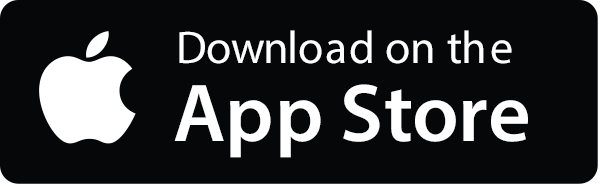
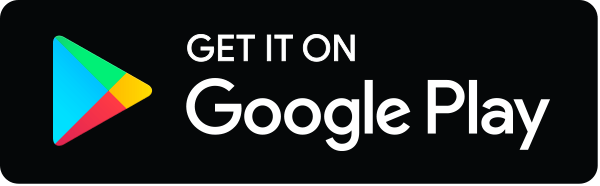