13 Emerging Technologies in Interventional Oncology
13.1 Introduction
Interventional oncology (IO) is a rapidly expanding field with continuous emergence of new concepts and approaches to both percutaneous and intravascular treatment of neoplasms. These approaches offer minimally invasive treatments targeting both primary and metastatic lesions. Although the modalities discussed earlier in the book are well entrenched in oncology treatment, numerous new approaches are under investigation. This chapter discusses promising new technologies in IO.
13.2 Interventional Navigational Systems
The concept behind an interventional navigational system (INS) is to provide a real-time, intraprocedural display of the spatial positions of various instruments. This typically involves integrating the real-time patient position and instrument data with preoperative imaging data. 1 , 2 INS has been successfully used in neurosurgery, otolaryngology, and orthopedic surgery. 3 In IO, INS is increasingly being used to co-locate the intraprocedural position of a needle or probe within the patient with previously obtained imaging data delineating a lesion of interest.
Preoperative images, usually from computed tomography (CT) or magnetic resonance imaging (MRI), are loaded into a control unit of the INS. The target lesion or location is mapped with three-dimensional (3D) coordinates; fiducial markers or standard anatomical landmarks are used for purposes of registration. These images may be used for surgical planning, including determining the entry point on the skin surface and the trajectory. During the procedure, the critical task of the INS is to track the 3D position of the surgical instrument with respect to the patient and to register this information superimposed on the preprocedure imaging. The performance of the INS is therefore dependent on a robust tracking system.
Tracking systems must be able to follow the location of the surgical instrument in real time because it is used to direct the operator. These systems generally fall into three main categories: optical, electromagnetic, and magnetic gradient tracking.
An optical tracking system requires two components—a position sensor and tracking markers. The position sensor will emit infrared light into a specified volume containing the surgical instrument to be tracked. The tracking markers are physically located on the instrument itself and may consist of passive or active markers. In the case of passive markers, a reflective coating redirects the infrared light back to the position sensor. The position of the instrument may then be calculated using various approaches, such as geometric triangulation and time-of-flight calculation. With active markers, the position sensor emits infrared light, which then triggers the marker to activate and emit its own infrared signal. The position sensor detects this signal and again calculates the position and orientation of the instrument. The information is transmitted to a host computer, which analyzes and displays the data. The information may be fused with the preoperative imaging displaying the real-time relationship of the instrument to a target of interest.
Despite improvements in accuracy and speed of processing, the main drawback of optical detection is that a line of sight must be maintained between the position sensor and the tracking marker. Any object, including the physician, disrupting this line of sight will disable the system. Additionally, accuracy of the system is decreased by any deformation of the instrument. With longer probes, a slight bending can occur with repositioning, causing the accuracy of the system to decrease during the procedure with repeated manipulation of the probe.
The principle of an electromagnetic (EM) tracking system is to localize the surgical instruments by detection of ferromagnetic probes within a magnetic field. 4 Rather than using light, the EM field is used for tracking. Instruments are fitted with sensor coils that move within a magnetic field created by an EM generator. This generates an electrical current according to Faraday’s law of electromagnetic induction. The magnitude of the induced voltage is used to calculate the position in Cartesian coordinates. At this point, the system is similar to optical systems where the information is passed to a host computer for analysis and data display. The advantage of EM is that the device does not require a direct line of sight. The sensor may in fact be within the patient’s body, allowing for direct tracking of the needle tip. This would theoretically reduce inaccuracies caused by bending of the needle/probe. A drawback of EM tracking is that the working space is smaller (< 1 m3). Metallic artifacts from objects such as tables and X-ray sources or detectors are also a problem, though to a lesser extent in newer-generation technologies.
INS has been developed for multiple imaging modalities ( Fig. 13.1 ). Computed tomography (CT) is generally the preferred imaging modality due to its spatial resolution, ability to provide quantitative information on the density and geometry, and widespread availability. Regardless of the tracking system, optical or EM, respiratory motion and deformation of the target organ remain as problems. Some of these inaccuracies can be reduced by tracking reference points and applying geometric transformation methods.
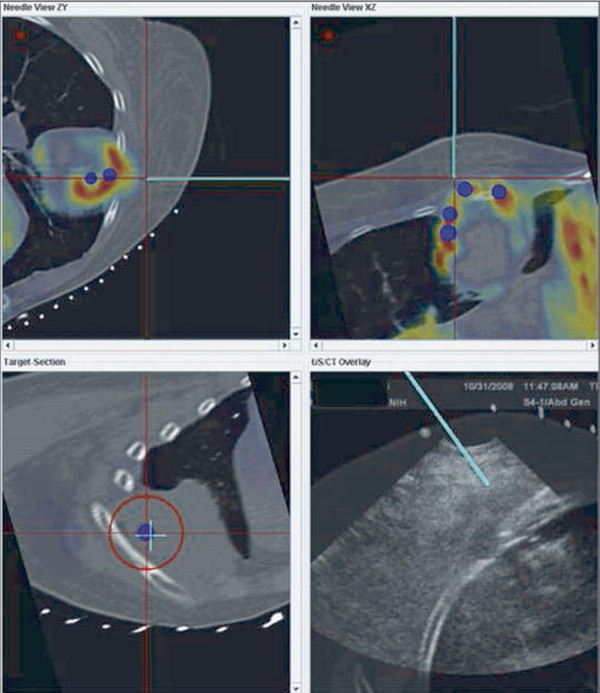
Ultrasound imaging has the advantages of being real time, low cost, and portable, as well as delivering nonionizing radiation. Again, optical and EM tracking has been explored, with some systems overlaying real-time ultrasound images with virtual images of the target.
Magnetic resonance imaging (MRI) systems contain a built-in gradient field, which may be used to implement EM tracking. 5 Three pairs of orthogonal coils are located in the hand piece of the sensor, which detect the gradient field of the MRI scanner. The location and orientation of the sensor are calculated by comparing the measured signals to stored maps of the gradient fields of the MRI scanner. The scan plane is prescribed in reference to the position and orientation of the tracking point by the tracking system. MRI has unique advantages, including superb soft tissue contrast and highly flexible image plane control. MRI-based navigation has been used in thermal ablation of liver tumors since 2000. 5 Real-time MRI scans are collected and overlaid with tracking coordinates from an optical or MRI gradient-based tracking system. However, the high cost and the large space and resource requirement are major impediments to wider adoption.
Different imaging modalities may also be combined. Preoperative imaging may be obtained on MRI while the procedure is performed by CT. If real-time visualization is desired, preoperative cross-sectional imaging from MRI or CT may be used with intraoperative ultrasound. In these systems, the tracking is again performed via optical or EM approach to localize the instrument. The data are then fused with the preoperative imaging to give a real-time display of the instrument position with respect to the target identified preoperatively ( Fig. 13.1 ). The ability to take advantage of multiple modalities may allow INS to use the best features of each modality, such as the soft tissue contrast of MRI with the real-time feedback of ultrasound.
INSs remain an area of great interest, with improvement in technology continually increasing accuracy, speed, and ease of use. These systems are poised to play an increasing role in interventional radiology.
13.3 Immunoembolization
Immunoembolization uses biological response modifiers or immunologic agents in combination with embolization to enhance the antitumor response, specifically in liver malignancies. It is a transcatheter intra-arterial treatment that consists of infusion of an immunologic agent into the hepatic artery followed by embolization.
The rationale behind the procedure is that, in normal liver, immune response is naturally suppressed despite the abundant presence of macrophages (Kupffer cells), antigen-presenting cells and cell populations part of innate immunity. This suppression is thought to be necessary to avoid overactivation of the immune system by persistent exposure to antigens from the gastrointestinal tract.
Immunoembolization, bland embolization, or chemoembolization initially leads to tumor destruction but also serves to provide tumor antigens to the local immune system. Concurrent use of biological response modifiers would then induce an inflammatory response, which improves the antigen presentation to the local immune system. Local stimulation of the immune system would then result in development of a systemic immune response against tumor cells suppressing the growth of untreated tumors and circulating cancer cells.
Kanai et al first described the use of OK-432 (Picibanil, Chugai Pharmaceutical Co., Ltd.) for immunoembolization in hepatocellular carcinoma patients. 6 OK-432 is a freeze-dried biological product prepared from Streptococcus pyogenes (group A) by treatment with benzylpenicillin and heat. It has been used as an anticancer agent in Japan since 1975 and has been reported to induce multiple cytokines, including interleukin (IL)-1, IL-2, interferon (IFN)-gamma, tumor necrosis factor (TNF)-alpha, IL-6, IL-8, granulocyte colony–stimulating factor (G-CSF), granulocyte-macrophage colony–stimulating factor (GM-CSF), IL-12, and IL-18. 7 Initially, protocols involved transcatheter arterial administration of the OK-432 compound followed by bland embolization with fibrinogen, thrombin, and lipiodol. 8 Various protocols have been reported since its first use, with the central concept being transcatheter arterial administration of an immunologic agent, followed by embolization or chemoembolization.
The approach has been pursued for patients with metastatic uveal melanoma predominantly using GM-CSF, a glycoprotein secreted by immune cells that increases myeloid cell production, stimulates macrophages, increases cytotoxicity of monocytes toward tumor cell lines, and promotes maturation of dendritic cells. Intra-arterial GM-CSF with Ethiodol was shown to be safe in a phase 1 trial in which 34 of the 39 patients in the study had metastatic uveal melanoma. All were considered unresectable. Patients underwent lobar hepatic artery embolization every 4 weeks using an escalating dose of GM-CSF with Ethiodol, followed by Gelfoam. CT of the chest/abdomen/pelvis and MRI of the liver were performed to assess results using Response Evaluation Criteria in Solid Tumors (RECIST), along with clinical assessment. Two patients had a complete response, 8 patients had a partial response (32% response rate), and 10 patients (32%) had stable disease. The median survival was 33.7 months for those with complete or partial responses compared with 12.4 months for those with stable or progressive disease.
Systemic progression-free survival was also prolonged in patients receiving higher-dose therapy—12.4 months compared to 5.6 months in patients receiving low-dose immunoembolization (≥ 1,500 μg for high dose, ≥ 1,000 μg for low dose, p < 0.05). This suggests that immunoembolization may induce a systemic immune response against the melanoma cells.
During follow-up, six patients underwent resection of extrahepatic metastases with pathology showing signs of immunologic response. Two showed CD4+ and CD8+ T cell and dendritic cell infiltration, and one showed monocyte infiltration with tumor necrosis. Of the 10 patients undergoing administration of 2,000 μg of GM-CSF, there was only one grade 3 toxicity (asymptomatic elevation of liver function tests) and one grade 4 toxicity (respiratory suppression due to narcotic use).
A subsequent randomized, double-blind, phase 2 clinical study in patients with metastatic uveal melanoma included 52 patients randomized to undergo embolization with Ethiodol and Gelfoam, with or without 2,000 μg GM-CSF. Both groups demonstrated induced cytokine production, but it was more prominent in patients receiving immunoembolization. Survival in patients with 20 to 50% tumor involvement was 18.2 months in the immunoembolization group versus 16 months for those in the bland embolization group (p = 0.047). Both immunoembolization and bland embolization were well tolerated with low toxicity profiles.
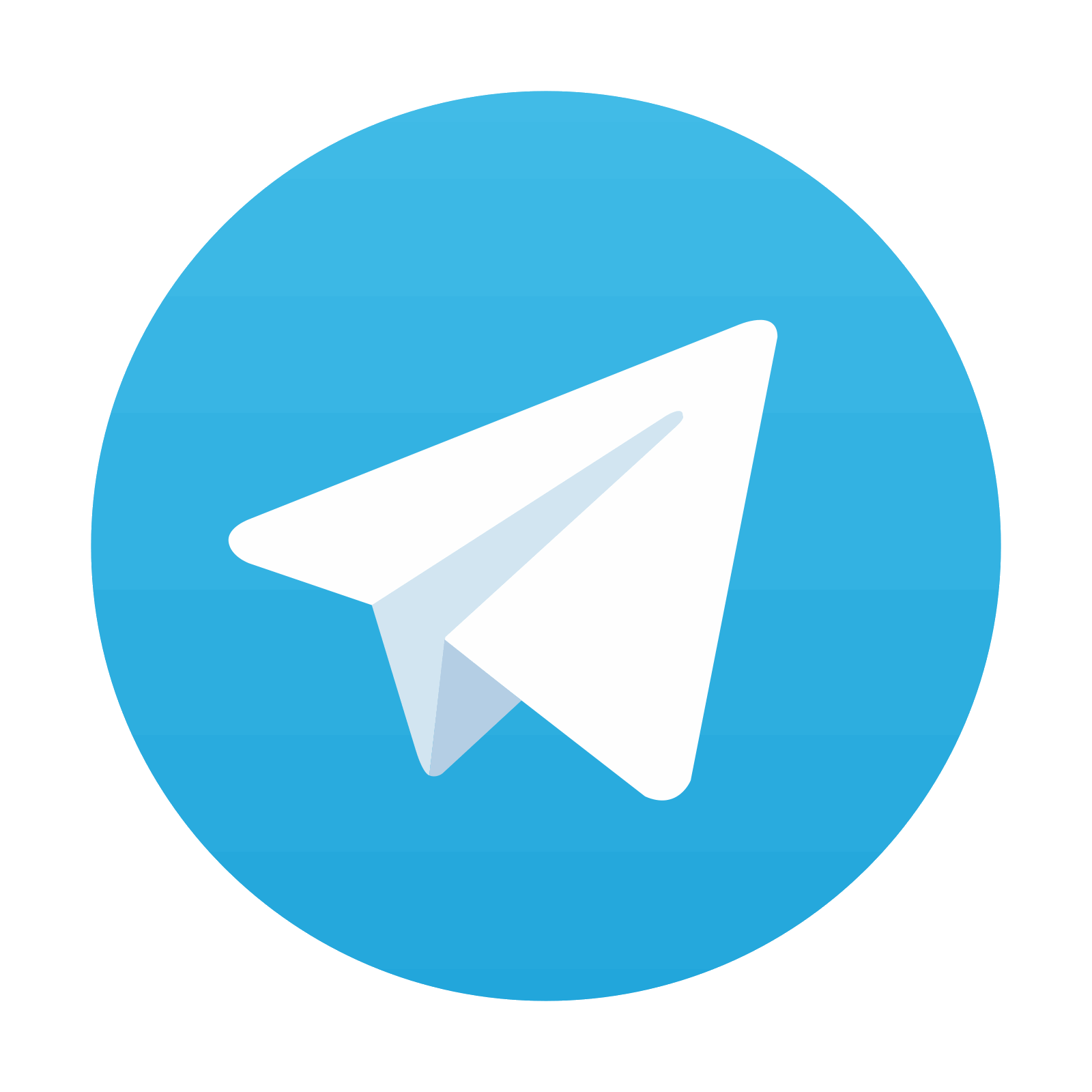
Stay updated, free articles. Join our Telegram channel
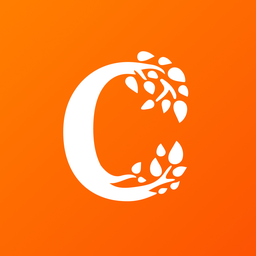
Full access? Get Clinical Tree
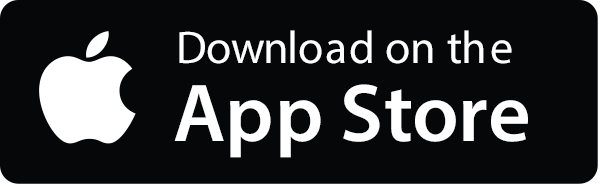
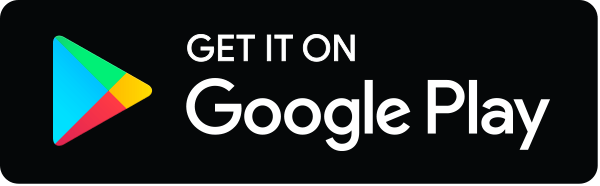